DOI:
10.1039/C7RA08319J
(Paper)
RSC Adv., 2017,
7, 50300-50306
Collagen modified with epoxidized safrole for improving antibacterial activity
Received
27th July 2017
, Accepted 21st October 2017
First published on 27th October 2017
Abstract
An epoxidized safrole, 5-(oxiran-2-ylmethyl)-benzo[d][1,3]dioxole (OYBD), was synthesized and employed to modify collagen for improving its antibacterial activity. The interaction between collagen and OYBD, and the structure/properties of the modified collagen were investigated in detail. The results indicated that the OYBD-modified collagen showed a higher de-nature temperature (Td, 90.2 °C), improved hydrophobic properties (contact angle from 84.2° to 89.1°) and enhanced tensile strength (6.2–11.0%) without destroying its triple helix structure. From observation of scanning electron microscopy (SEM), a higher density of intertwining morphology and a more stable network structure were observed, which was consistent with improved tensile strength and reduced breaking extension stress. The antibacterial test and LIVE/DEAD Baclight bacterial viability assay illustrated that the modified collagen exhibited excellent antibacterial activity to both Gram-negative and Gram-positive bacteria. Furthermore, the OYBD-modified collagen still exhibited cytocompatibility, supporting human fibroblast proliferation, which holds a great potential for developing antibacterial collagen-based biomaterials.
1. Introduction
Collagen is the major structural and functional element of connective tissues, and is also found in large quantities in the interstitial tissues of virtually all parenchymal organs, where it maintains the structural integrity of tissues and contributes to various physiological functions.1,2 Unfortunately, natural collagen is particularly susceptible to microbial colonization. Microbial contaminations compromise the quality of the collagen product as they introduce variability and cause loss of potency due to collagen degradation by microbial enzymes, changes in impurity profiles, and also an increase in the levels of bacterial endotoxins. Generally, cross-linking is an efficient strategy to improve the durability of collagen towards bacteria degradation, as well as to optimize its mechanical properties.3–5 Collagen contains abundant amino and carboxyl side groups, which provides convenience for its chemical modification.6 Although some modifiers, such as diisocyanates, glutaraldehyde, and aziridin could connect the amine groups between two adjacent polypeptide chains of collagens, improving the resistance against collagenase and bacteria,7,8 it is noteworthy that these crosslinkers might cause cytotoxicity and further destroy of the triple helical structure of collagen that has been identified as being of importance for its functional and bioactive properties.
Previous studies demonstrated that collagen cross-linked with epoxy compounds displayed comparable mechanical properties to those cross-linked by glutaraldehyde.5 Additionally, cross-linking with epoxy compound showed no signs of cytotoxic degradation products.9,10 To improve the antibacterial activity of collagen, we synthesized a new modifier in this study, named 5-(oxiran-2-ylmethyl)-benzo[d][1,3]dioxole (OYBD), derived from natural safrole (4-allyl-1,2-(methylenedioxy)benzene). This modifier contained an epoxy group and a piperonyl group. The former could react with amine groups of collagen, and the latter was known as antibacterial. After modification, the collagen would be expected to yield desirable antibacterial activity as well as enhanced thermal and mechanical properties.
2. Experimental
2.1 Materials
Pigskins were obtained from a local tannery. Safrole (≥97%) was purchased from Sigma-Aldrich (St. Louis, MO) and used as received. Pepsin (3000–3500 U g−1), MTT (dimethyl thiazolyl tetrazolium bromide), and 3-chloroperbenzoic acid (mCPBA) were purchased from Sino Standards Biotechnology Co. Ltd. (Chengdu, China). Dichloromethane, NaOH, MgSO4, NaCl, and HAc were obtained from Kelong Chemical Engineering Co. Ltd. (Chengdu, China). All chemicals were of analytical grade or biological reagent grade.
2.2 Synthesis of 5-(oxiran-2-ylmethyl)-benzo[d][1,3]dioxole (OYBD)
The synthesis of OYBD was conducted according to the following procedure. m-chloroperbenzoic acid (mCPBA) (31.8 g, 0.15 mol) was first dissolved in 200 mL of dichloromethane (DCM), followed by addition of safrole (16.2 g, 0.1 mol) at room temperature. After stirred for 24 h, the mixture was filtered, and the precipitate was discarded. The supernatant was washed with 5% NaOH solution three times and subsequently with deionized water three times. The organic layer obtained was dried over anhydrous magnesium sulfate (MgSO4). The solvent was removed by distillation (110 °C, 530 Pa), and OYBD was finally obtained with a yield of 82%.
2.3 Preparation of native collagen
The pig skin was first pulverized with a mill (Fristsch puluterisette, ldar-Oberstein, Germany) and then suspended in a mixture of 0.5 M HAc (3%, w/v) and pepsin (2%, w/w) at 4 °C for 48 h under magnetic stirring. After that, the supernatant was collected through centrifugation at 10
000 rpm for 10 min (4 °C) and then salted using excessive NaCl. The precipitate was subsequently dialyzed against 0.1 M HAc at 4 °C for 3 days to obtain native collagen. The collagen was lyophilized using a lyophilized at −50 °C for 48 h for further use.
2.4 Modification of collagen by OYBD
About 0.5 g native collagen was first dissolved in 0.5 M HAc. The mixture was cast to form an aqueous membrane, which was then dried at 37 °C until a constant weight. The resultant collagen membrane was immersed in 100 mL of an ethanesulfonic acid (MES, TRC, Canada) buffer solution (pH = 8.0) containing 5 wt% OYBD. The epoxidation reaction was conducted at room temperature for 36 h. After that, the collagen membrane was rinsed in phosphate buffer saline for 24 h and washed with deionized-water five times. The resultant material was denoted as collagen-OYBD.
2.5 1H NMR detection
The 1H NMR spectrum of OYBD was recorded by a NMR spectrometer (AV11-600MH, Bruker, Swiss) with a test frequency of 600 MHz. The trifluoroacetic acid-D (99.5 atom% D) was used as deuterium reagent, and tetramethylsilane (TMS) as internal standard.
2.6 FTIR spectra measurement
Fourier transform infrared (FTIR) spectra were collected using a Nicolet iS10 FTIR spectrometer (Thermo Fisher Scientific, United States) over a wavenumber range from 500 to 4000 cm−1 after 64 scans at a resolution of 4 cm−1.
2.7 DSC measurement
Briefly, 3 mg collagen specimens were loaded in an aluminum pan. The sample pan and the empty reference aluminum pan were both placed in a Netzsch DSC 200 PC operating in N2 flow. Differential scanning calorimetry (DSC) analysis was conducted in the temperature range from 20 to 180 °C with a heating rate of 4 °C min−1.
2.8 SEM observation
The morphology of the lyophilized collagen samples (1 cm × 1 cm) was observed with a scanning electron microscopy (SEM, Nova Nano SEM450, FEI, USA). The collagen specimen was sputter-coated with aurum and imaged at an accelerating voltage of 5 kV.
2.9 Water contact angle (WCA) test
The hydrophilicity of the OYBD-modified collagen was measured using a goniometer (Dataphysics, OCAH200, Germany) at room temperature. Briefly, 5 μL per drop of distilled water was drop-wise deposited on the surface of the collagen, and the WCA was calculated using a shape DSA100 analysis system. Each sample was tested for five different surface areas to obtain an average value.
2.10 Mechanical measurement
The collagen membrane was cut into a dumbbell shape with length and width of 50.0 mm × 10 mm using a dumbbell-shaped knife. The thickness of the samples was measured at three different areas using a micrometer, and an average value was calculated. Using a universal tensile testing machine (GT-AI-7000S, Hi tech, Taiwan), a crosshead speed of 1 mm min−1 was applied until the collagen membrane ruptured. The tensile strength, elongation at maximum force, and elongation at break of the collagen sample were calculated, and each sample was measured three times.
2.11 Antibacterial measurement
Escherichia coli (E. coli, ATCC 25922) was selected as the indicator in evaluating the antibacterial activity of the OYBD-modified collagen. A small quantity of recovered cultures of E. coli was diluted with saline to a concentration of 1 × 108 colony-forming unit (cfu) per mL. Subsequently, 2 mL of E. coli suspensions were transferred into a tube containing 18 mL of sterile water, followed by addition of 0.15 g collagen membrane that had been subject to irradiation sterilization. Furthermore, the suspension was shook for 1 h. Afterwards, 1 mL of the resulting mixture was incorporated into 9 mL of sterile water to produce a series of dilution. For each diluted tube, 0.1 mL of the bacterial suspension was evenly distributed onto a sterilized nutrient agar Petri dish and incubated for 48 h in an incubator at 37 °C. The number of viable micro-organisms colony and the antibacterial ratio were measured.
where, w1 is the cfu of collagen and w2 is the cfu of the OYBD-modified collagen.
2.12 LIVE/DEAD Baclight bacterial viability test
The LIVE/DEAD Baclight bacterial viability kit was used to visualize the bacterial cells on the modified collagen. The fluorescent dyes agents of green stain SYTO 9 (label both live and dead cells) and red stain propidium iodide (only penetrate cells with compromised or damaged membranes) could bind to nucleic acids to assay cell viability. A suspension of Staphylococcus aureus (S. aureus, ATCC 25923) in PBS (105 CFU mL−1, 50 μL) was added onto the collagen samples,11 which was then incubated for 24 h at 35 °C. Subsequently, the collagen sample was washed with PBS buffer and soaked in SYTO 9 (4 μL) and propidium iodide (6 μL) dye solution in dark condition for 15 min. Finally, the stained bacterial cells were observed using an IX-71 inversed fluorescent microscope (Olympus America Inc., Melville, NY).
2.13 Cytocompatibility analysis
The cell proliferation assay was carried out to evaluate the cytocompatibility of the modified collagen according to ISO 10993-5. Normal human dermal fibroblasts (NHDFs) were seeded in a culture dish and cultured to near confluence at 37 °C under 5% CO2. Collagen solution was diluted to a concentration of 20 μg mL−1 with 0.05 M acetic acid solution, and then added into the wells of a 24-well polystyrene plate. After incubation for 12 h, the solution was neutralized with 0.05 M sodium hydroxide solution, and the wells were rinsed with PBS three times. Subsequently, NHDFs (1 × 105 cells) in 2 mL Dulbecco's Modified Eagle's Medium (DMEM) with 10% fetal bovine serum were seeded. After incubation at 37 °C with 5% CO2 for 1, 3, and 7 d respectively, cell proliferation was measured using the methyl thiazolyl tetrazolium (MTT) assay. In addition, the morphologies of cells were observed by employing an IX83 2-deck inverted microscope (Olympus).
2.14 Statistical analysis
The reported data are the means ± standard deviation of triplicate samples for each measurement. Statistical analysis was performed with Student's t-test. P < 0.05 was accepted as statistically significant.
3. Results and discussion
3.1 Cross-linking between collagen and OYBD
The 1H NMR measurement was performed to confirm the structure of 5-(oxiran-2-ylmethyl)benzo[d][1,3]dioxole (OYBD) and the results were shown in Fig. 1. Safrole was oxidized by the weak oxidant (mCPBA), forming a monofunctional epoxy group, which could react with those amino groups originated from collagen. The 1H NMR detection results of OYBD are as follows: 1H NMR (600 MHz, DMSO-d6) δ: 6.91–6.66 (m, 3H, ArCH), 5.98 (s, 2H, –OCH2O–), 3.06 (dp, J = 5.9, 3.0 Hz, 1H, –OCH(CH2)CH2–), 2.79–2.61 (m, 3H, –ArCH2–CH and –OCH2CH), 2.53 (dd, J = 5.1, 2.6 Hz, 1H, –OCH2CH). Such result is consistent with that as previously reported.12,13
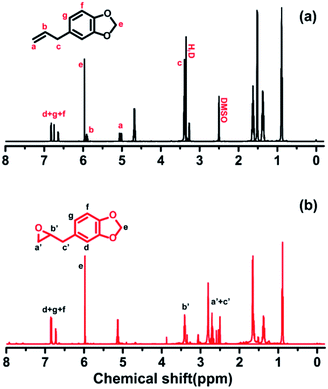 |
| Fig. 1 1H NMR spectra of (a) safrole and (b) 5-(oxiran-2-ylmethyl)benzo[d][1,3]dioxole. | |
Possible interactions between collagen and OYBD are illustrated in Fig. 2. According to previous studies, collagen can be modified by monofunctional and multifunctional poly epoxy compounds under alkaline condition.14 The synthesized OYBD contained an epoxidized structure that could react with the amine groups of lysine residues in collagen under alkaline condition (pH 8.0). Thus, the antibacterial piperonyl group can be covalently introduced into the collagen macromolecules.
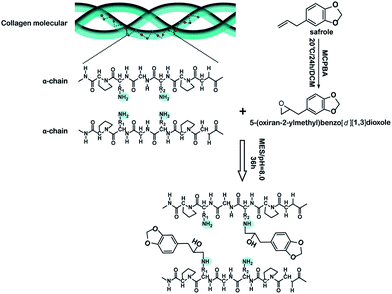 |
| Fig. 2 Schematic illustration of possible interactions between collagen and OYBD. | |
3.2 The triple-helical structure retention of collagen
An ideal modification strategy should not destroy the special triple-helical structure of tropocollagen molecules that is the basis of many important and specific biological functions of collagen.15,16 In general, the FTIR absorption band at 1235 cm−1 corresponds to the C–N bond in-plane vibration (amide III) and the N–H stretch (amide I), which is associated with the triple-helical structure of tropocollagen molecules. The absorption band at 1450 cm−1 can be ascribed to the pyrrolidine ring vibration of proline and hydroxyproline, which is not affected by changes in collagen secondary structure. Thus, it is widely accepted that the intensity ratio of FTIR absorption at 1235 and 1450 cm−1 (I1235/1450 cm−1) can be used as a sensitive indicator of the integrity of triple-helical structure in collagen.17 Typically, an I1235/1450 cm−1 close to unity indicates intact triple-helical structure, whereas an I1235/1450 cm−1 significantly lower than unity suggests the triple helicity has been completely destroyed.18 Based on the FTIR spectra in Fig. 3, the I1235/1450 cm−1 of the OYBD-modified collagen was 0.91 and is comparable to the value of native collagen (1.0). This result revealed that the triple helix conformation was not destroyed in the modification process by OYBD.
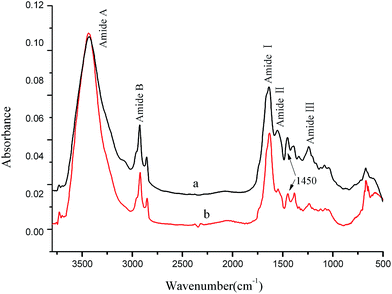 |
| Fig. 3 FTIR spectra of (a) native collagen and (b) OYBD-modified collagen. | |
In addition, quantitative analysis of molar ellipticity changes in CD spectra before and after OYBD treatment could also provide valuable information regarding the integrity of triple-helical structure. CD spectroscopy is the most widespread technique used for estimating the secondary structures of proteins and polypeptides in solution. This technique can be used to distinguish between unordered (random coil) and ordered (triple helix) structures of collagen. CD detects wavelength-dependent differences in the absorption of right and left circularly polarized light by optically active molecules such as collagen. The CD spectrum of unordered collagen is usually characterized by a single band below 200 nm, whereas triple-helical structure usually presents one negative band at 223 nm, along with one positive band at 197 nm.19,20 It has been well established that the absolute ratio value of molar ellipticity at 220 nm over molar ellipticity around 195–197 nm (Rpn) can be used to characterize the integrity of triple-helical conformation of collagen in solution. As shown in Fig. 4, the OYBD modified collagen exhibited a positive peak at 223 nm and a negative peak at 197 nm, respectively. Additionally, the Rpn value of the OYBD-modified collagen (0.13) was comparable to that of the native collagen (0.14), suggesting that modification by OYBD did not destroy the triple helix structure of collagen. This conclusion is well consistent with FTIR analysis.
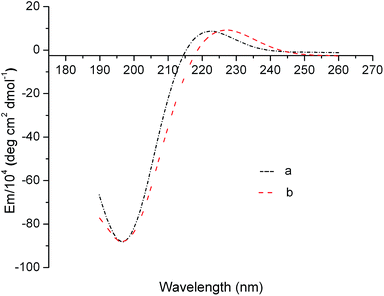 |
| Fig. 4 CD spectra of (a) native collagen and (b) OYBD-modified collagen. | |
3.3 De-nature temperature of collagen
Differential scanning calorimetry (DSC) is used to analyze the endothermic shrinkage transition of collagen. As shown in Fig. 5, the OYBD-modified collagen showed higher de-nature temperature (90.2 °C) than native collagen (69.9 °C). This phenomenon occurred because OYBD reacted with –NH2 from lysine or hydroxylysine side groups of collagen and, forming a more stable structure, which enhanced the thermal stability of collagen.21
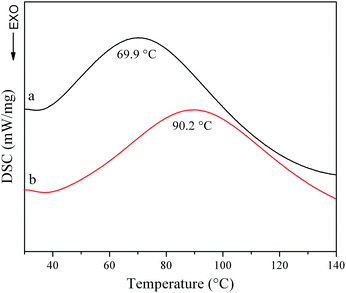 |
| Fig. 5 Differential scanning calorimetry curves of (a) native collagen and (b) OYBD-modified collagen. | |
3.4 Microstructure appearance of collagen
The morphology and porosity of porous materials are very important for the scaffolds. The morphology of collagen membrane was observed by SEM. As shown in Fig. 6, the fibers of the OYBD-modified collagen exhibited an intertwining morphology and more dense, intensive fiber structure under ×100, ×1200, and ×5000 magnification compared with native collagen, indicating efficient crosslinking between collagen and OYBD.
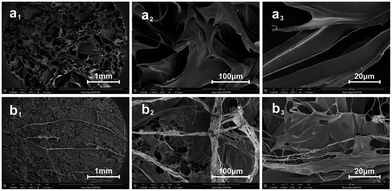 |
| Fig. 6 SEM images of (a1), (a2), and (a3) native collagen and (b1), (b2), and (b3) OYBD-modified collagen under different magnifications. | |
3.5 Hydrophobic–hydrophilic properties of collagen
The hydrophobic/hydrophilic properties of collagen can be evaluated by the value of water contact angle (WCA). In general, a low value of WCA means higher hydrophilicity, whilst a high value of WCA indicates better hydrophobicity.22 As shown in Fig. 7, the value of WCA is 89.1 ± 1.4° for OYBD-modified collagen and 84.2 ± 0.8° for native collagen, suggesting that OYBD modification increased the hydrophobicity of collagen. The underlying mechanism is that a certain amount of the hydrophobic phenoxy groups is introduced into the collagen side chains in the modification process, which leads stronger water repellence of the surface, and thus hydrophobicity. An increased hydrophobic property of the OYBD-modified collagen is believed to be favorable in preventing water and bacteria intrusion for collagen-based biomaterials.
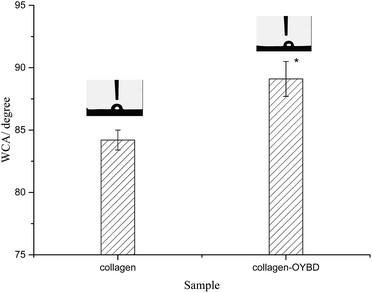 |
| Fig. 7 Water contact angle of native collagen and OYBD-modified collagen. Statistical significance: *p < 0.05. The reported data are mean ± S.D. (N = 3). | |
3.6 Mechanical properties of collagen
Cross-linking can efficiently increase the mechanical properties of the collagen-based materials, especially, the tensile strength and strain modulus.23 As illustrated in Fig. 8, collagen crosslinked with OYBD at pH 8.0 had exhibited increased tensile strength (42.9 MPa) and elongation at break compared with native collagen. The enhanced tensile strength of the modified collagen may be related to the nature of inter-microfibrillar cross-links.14 As shown in the SEM images in Fig. 6, the modified collagen displays a more dense microfibrillar structure which suggests that the OYBD-induced cross-links hinder the slippage of collagen fibrils and consequently creates internal stresses in the fibers. Thus, the modification with OYBD increases the mechanical properties of the collagen.
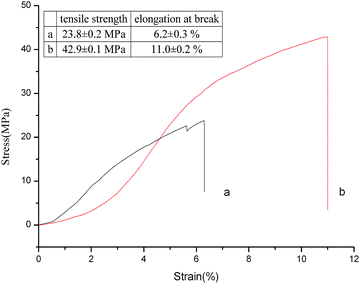 |
| Fig. 8 Typical uniaxial stress–strain curves of (a) native collagen and (b) OYBD-modified collagen (20 °C, distance between clamps: 1 cm, pull speed: 1 mm min−1, N = 3). The reported data are mean ± S.D. (N = 3). | |
3.7 Antibacterial activity of collagen
As shown in Fig. 9, the colony-forming unit (cfu) of all dilution from the modified collagen is less than those from the native collagen. The antibacterial ratio of the OYBD-modified collagen is 51.6% when the suspension was diluted to 1
:
100, while the antibacterial ratio reached up to 100% at the dilution of 1
:
1000. The antibacterial activity against E. coli is the result of the unique piperonyl structures from OYBD. This structure is suggested to be very important in many compounds with physiological activity.24 For E. coli, a type of typical Gram-negative bacteria, the inhibitory activity of the OYBD-modified collagen is attributed to the destruction of the peptidoglycan layer and lipo-polysaccharide. More interestingly, the antibacterial activity of the OYBD-modified collagen for Gram-negative bacteria has a potential application in collagen based biomaterials.
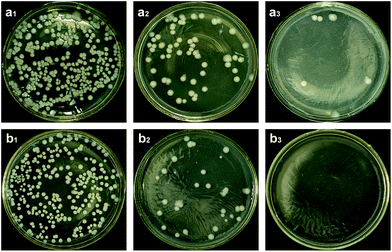 |
| Fig. 9 The cfu of (a) native collagen and (b) OYBD-modified collagen. a1(b1), a2(b2) and a3(b3) represent the dilution of 10−1, 10−2 and 10−3. | |
The LIVE/DEAD Baclight bacterial viability test is another analytical method to detect the antibacterial activity of both Gram-negative and Gram-positive bacterial. The green stain SYTO 9 is particularly useful as a nuclear counter stain for bacterial assays since it can stain both live and dead Gram-positive bacteria.25 The red stain propidium iodide (PI) can only penetrate cells with compromised or damaged membranes. As shown in Fig. 10, the predominance of green fluorescence (live bacteria) on native collagen after dyed by SYTO 9 and PI indicated most of the seeded S. aureus bacteria remained viable, which suggested the collagen and/or polypeptide, amino acid are beneficial for bacterial growth. Nevertheless, little green fluorescence could be found on collagen-OYBD after stained by SYTO 9 and PI, which suggested that the collagen-OYBD remained antibacterial activity for Gram-positive bacteria.
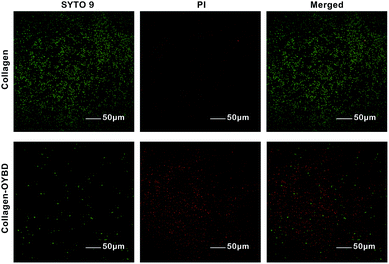 |
| Fig. 10 Fluorescent images of S. aureus seeded on native collagen and OYBD-modified collagen (green, live bacteria; red, dead bacteria). The merged images represent the superposition of live and dead bacteria. | |
3.8 Cytocompatibility assay
With intact triple-helical confirmation, the biological function of collagen modified with OYBD remained uncompromised. The morphologies of human fibroblasts cultured in collagen-coated PS wells for three days are illustrated in Fig. 11(1). Clearly, the cells seeded on OYBD-modified collagen showed similar morphologies to those on native collagen, exhibiting typical fibroblast morphologies, a spindle shape. This observation demonstrated that the modified collagen supported attachment and differentiation of human fibroblasts as efficiently as native collagen. In addition, the fibroblast proliferation rate was also quantitatively evaluated by using the MTT assay. As illustrated in Fig. 11(2), human fibroblast not only remained viable, but also proliferated on the OYBD-modified collagen, significantly faster than those on gelatin-coated wells for the same seeding time. This was because gelatin has no triple-helical confirmation,26 and thus did not act as an efficient support for cell attachment and proliferation.
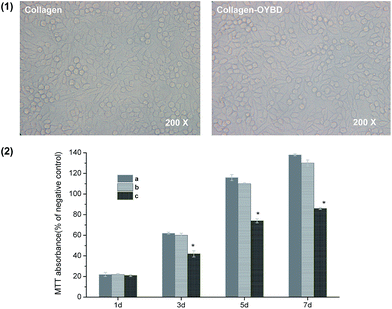 |
| Fig. 11 (1) The morphology of fibroblasts on the culture plates at day 3; (2) cytotoxicity tests from MTT assays of cell viability over a period of 7 days ((a) collagen, (b) OYBD-modified collagen, (c) gelatin). Statistical significance: *p < 0.05. The reported data are mean ± S.D. (N = 3). | |
4. Conclusions
Modification of collagen with epoxidized safrole, 5-(oxiran-2-ylmethyl)-benzo[d][1,3]dioxole (OYBD) under alkaline condition resulted in a modified collagen with increased thermal stability, enhanced mechanical properties, improved hydrophobic properties together with more density intermicro-fibrillar structure. Especially, the introduction of OYBD did not destroy the triple helix conformation of native collagen according to FTIR and CD analysis. Additionally, antibacterial tests revealed that the modified collagen inhibited proliferation of both Gram-negative bacterial E. coli and Gram-positive bacteria S. aureus efficiently. Simultaneously, the collagen-OYBD exhibited excellent cytocompatibility. According to these results, OYBD holds a great potential as a novel modifier for producing antibacterial collagen-based biomaterials.
Conflicts of interest
There are no conflicts to declare.
Acknowledgements
The authors wish to acknowledge the financial support from National Natural Science Foundation of China (No. 51273128, 21206096) and National Key R&D Program of China (No. 2017YFB 0308600).
References
- R. Zeeman, P. J. Dijkstra and P. B. Van Wachem, J. Biomed. Mater. Res., 1999, 46, 424–433 CrossRef CAS PubMed.
- D. M. Simmons and J. N. Kearney, Biotechnol. Appl. Biochem., 1993, 17, 23–29 CAS.
- R. Tu, S. H. Shen and D. Lin, J. Biomed. Mater. Res., 1994, 28, 677–684 CrossRef CAS PubMed.
- J. M. Lee, C. A. Pereira and L. W. K. Kan, J. Biomed. Mater. Res., 1994, 28, 981–992 CrossRef CAS PubMed.
- T. Xi and F. Liu, J. Biomater. Appl., 1992, 7, 61–75 CrossRef CAS PubMed.
- R. Sripriya, R. Kumar, S. Balaji, M. S. Kumar and P. K. Sehgal, React. Funct. Polym., 2011, 71, 62–69 CrossRef CAS.
- L. H. H. Olde Damink, P. J. Dijkstra, M. J. A. van Luyn, P. B. van Wachem, P. Nieuwenhuis and J. Feijen, J. Mater. Sci.: Mater. Med., 1995, 6, 429–434 CrossRef.
- R. Tu, R. C. Quijano and C. L. Lu, et al., Int. J. Artif. Organs, 1993, 16, 537–544 CAS.
- J. M. Lee, C. A. Pereira and L. W. K. Kan, J. Biomed. Mater. Res., 1994, 28, 981–992 CrossRef CAS PubMed.
- J. M. Lohre, L. Baclig, J. Sagartz, S. Guida, K. Thyagarajan and R. Tu, Artif. Organs, 1992, 16, 630–633 CrossRef CAS PubMed.
- R. Garcíapumarino, G. Pascual and M. Rodríguez, et al., J. Biomed. Mater. Res., Part B, 2013, 102, 366–375 CrossRef PubMed.
- L.-Y. Wang, X.-H. Wang and J.-L. Tan, Bull. Korean Chem. Soc., 2012, 33, 3571–3575 CrossRef CAS.
- B. X. Zhao, D. W. Wang, H. Zuo, G. T. Qian and J. Y. Miao, Chin. J. Org. Chem., 2003, 23, 1026–1028 CAS.
- R. Zeeman, P. J. Dijkstra and P. B. V. Wachem, Biomaterials, 1999, 20, 921–931 CrossRef CAS PubMed.
- H. Zhou, R. P. Xun, Z. H. Zhou, Q. Q. Liu, P. Wu and K. J. Wu, Fibers Polym., 2014, 15, 519–524 CrossRef CAS.
- M. E. Andrews, J. Murali, C. Muralidharan, W. Madhulata and R. Jayakumar, Colloid Polym. Sci., 2003, 281, 766–770 CAS.
- L. He, C. Mu, J. Shi, Q. Zhang, B. Shi and W. Lin, Int. J. Biol. Macromol., 2011, 48, 354–359 CrossRef CAS PubMed.
- B. Madhan, V. Subramanian and J. R. Rao, Int. J. Biol. Macromol., 2005, 37, 47–53 CrossRef CAS PubMed.
- D. I. Zeugolis, S. T. Khew and E. S. Yew, et al., Biomaterials, 2008, 29, 2293 CrossRef CAS PubMed.
- J. Chang, Y. Chen and S. Zhao, et al., Polym. Chem., 2015, 6, 8150–8160 RSC.
- B. Balakrishnan, M. Mohanty, P. R. Umashankar and A. Jayakrishnan, Biomaterials, 2005, 26, 6335–6342 CrossRef CAS PubMed.
- P. Fratzl, Collagen: Structure and Mechanics, Springer, New York, NY, USA, 2008 Search PubMed.
- P. F. Gratzer and J. M. Lee, J. Biomed. Mater. Res., 1997, 37, 497–507 CrossRef CAS PubMed.
- D. Ge, Q. Jing, W. Zhao and H. Yue, et al., PLoS One, 2014, 9, e99378 Search PubMed.
- C. Yang, X. Ding and R. J. Ono, et al., Adv. Mater., 2014, 26, 7346–7351 CrossRef CAS PubMed.
- G. Y. Li, S. Fukunaga, K. Takenouchi and F. Nakamura, Int. J. Cosmet. Sci., 2005, 27, 101 CrossRef CAS PubMed.
|
This journal is © The Royal Society of Chemistry 2017 |
Click here to see how this site uses Cookies. View our privacy policy here.