DOI:
10.1039/C7RA09828F
(Paper)
RSC Adv., 2017,
7, 50294-50299
One-pot enzyme-catalyzed synthesis of dual-functional polyester macromers towards surface-active hydrophobic films†
Received
4th September 2017
, Accepted 19th October 2017
First published on 27th October 2017
Abstract
Selective enzyme catalysis is a valuable tool for the processing of monomers into value-added materials. In the present study natural resources were used to retrieve an ω-hydroxy fatty acid monomer containing an epoxide functionality. A procedure was developed for the synthesis of dual-functional oligomers by utilizing lipase catalysis in a one-pot synthesis route. The chemoselectivity of the enzyme allowed addition of thiol monomers to the retrieved epoxy monomers, without harming the epoxides, achieving a thiol–epoxy functional polyester resin. The synthesis reached full conversion (>99%) after 8 h. It was possible to selectively crosslink the resin through UV-initiated cationic polymerization of the epoxides into thiol–functional thermosets. The curing performance was followed in situ by real-time FTIR. The thiol groups on the surface of the film were accessible for post-modification.
Introduction
The development of the bio-refinery concept has started to introduce totally new building blocks for future advanced materials.1,2 This also implies that new chemistries and technologies need to be further developed in order to utilize these sources optimally. Nature provides monomers containing wide variety of functional groups ranging from, hydroxyls, carboxylic acids, amines, epoxides, thiols etc.2 Combining these would allow totally new multifunctional structures to be made. These multifunctional structures have a great potential in applications where the surface properties needs to be tailored such as wetting,3 adhesion,4 post-functionalization,5,6 adhesion promoters (primers),7 microfluidics8 and biosensors9 just to mention a few.
One interesting renewable source of building blocks is epoxidized fatty acids either from vegetable oils10 or other sources such as suberin and cutin.11 The physical properties of thermosets based on these building blocks can be changed by connecting the epoxidized fatty acids to a more rigid core than in the naturally occurring triglycerides12,13 or by reducing the dangling-ends inside the network.14
One specific fatty acid structure in the context of multifunctional building blocks is 9,10-epoxy-18-hydroxyoctadecanoic acid (EFA) extracted from outer birch bark. EFA stands for about 10 wt% of the total dried outer birch bark.15,16 The molecule contains three functional groups hence; making it prone to many types of reactions.
Thermoset resins based on epoxide monomers have been extensively studied in the past. The epoxide group is considered versatile due to the fact that it is easily reacted with various functional groups.4 Epoxides can react in both chain-wise and stepwise polymerization techniques i.e. via cationic polymerization17–20 or amine coupling reactions21,22 respectively. The advantage of cationic polymerization is that it is considered as oxygen insensitive and has rapid curing rates. In addition the polymerization of epoxides proceeds for a long period of time due to the effect of post-polymerization i.e. propagating species proceeds after e.g. UV irradiation.17,19
Another type of chemistry that has recently received significant interest as a tool to enable the use of bio-derived monomers is the so-called thiol-ene reaction.23 This has been combined with fatty acids to make polyols24 and enabled the use of terpenes.25 Conventional thiol-ene chemistry is used for different applications such as surface modification,6 biomedical applications,26 microfluidics,8 and optics and electronics.23 The thiol-ene reaction proceeds via a free-radical step-growth process and is considered fast and insensitive to oxygen.23,27
Introducing thiol end-groups in resins enable post-functionalization reactions. However, such an introduction could be a tedious process when using conventional polymer synthesis because the thiol group requires protection and deprotection chemistry.28–30 In order to overcome this issue one can use biocatalysis e.g. enzymes.
Enzymes are considered environmentally friendly, selective, effective and operate under mild conditions.31 One of the most extensively used enzymes in polymer chemistry is Novozyme 435 (immobilized Candida antarctica lipase B (CALB)). CALB is considered versatile and robust due to its ability to catalyze acyl transfer reactions using a large number of different substrates and tolerate a variation of experimental conditions.32–34 It has previously been shown that end-capped thiol–functional resins can be synthesized by CALB.35–37 Moreover, CALB is more chemo-selective towards alcohols than thiols.38 Furthermore, CALB has been utilized to catalyze reactions with EFA effectively while preserving the mid-chain epoxy group39 and to add additional functionalities while forming the oligoesters.40–42 These additional functionalities play an important role in the final properties of the materials depending on the crosslinking method used. Biocatalysis broadens the spectrum of catalysts in polymer chemistry. Moreover, it is possible to obtain new bio-based building blocks by biocatalysis otherwise hard to obtain by conventional polymer chemistry.
In this paper we describe an enzymatic route for the synthesis of a thiol–epoxy-functional resin starting from renewable monomers. These resins are then cationically polymerized to lead to films with a thiol-functionality available for post-functionalization.
Experimental
Materials
The fatty acids were retrieved from natural resources; 9,10-epoxy-18-hydroxyoctadecanoic acid (EFA, 1) from birch bark provided by Holmen AB (c/o Holmen Energi, SE-89180) and epoxidized methyl oleate (EMO) from epoxidized linseed oil provided by Ackross Chemicals. 11-Mercaptoundecanol (98%) (2), dimethyl adipate (≥99%) (3), Novozyme 435 (immobilized CALB), 5,5′-dithiobis-(2-nitrobenzoic acid) (≥98%, Bioreagent) (DTNB), phosphate buffer pH 7.0 (1 M, 20 °C), and molecular sieves 4 Å (8–12 mesh) were purchased from Sigma Aldrich. The photoinitiators UVAcure 1600 and benzophenone (≥99%) were supplied by CYTEC and Fluka respectively. 1,6-Hexanediol mono vinyl ether (HMVE) was supplied by BASF. All materials were used as received, unless otherwise noted.
Analytical methods
Nuclear magnetic resonance (NMR). In order to obtain 1H NMR and 13C NMR spectra of the different compounds a Bruker spectrometer (400 MHz) was used. The data for 1H NMR spectra were recorded at 400 MHz using 32 scans and a relaxation time of 1 s. The data for 13C NMR spectra were recorded at 400 MHz using 512 scans and a relaxation time of 1 s. Samples were diluted with CDCl3 containing tetramethylsilane (TMS). All chemical shifts (δ) where reported in parts per million (ppm) and the residual solvent peak of CDCl3 singlet at 7.26 ppm for 1H NMR and the central line of the triplet at 77.16 ppm for 13C NMR was used as reference respectively. The obtained spectra were analyzed with MestReNova v9.0.0-12821 (Mestrelab Research S.L. 2013).
Matrix-assisted laser desorption ionization time of flight mass spectrometry (MALDI-TOF-MS). MALDI-TOF-MS measurements were conducted on a Bruker UltraFlex MALDI-TOF MS with SCOUT-MTP Ion Source (Bruker Daltonics, Bremen) equipped with a N2 laser (337 nm), a gridless ion source and reflector design. The recorded spectra were acquired using a reflector-positive method with an acceleration voltage of 25 kV and a reflector voltage of 26.3. The system was calibrated using SpheriCal™ calibrants and the detector mass range was set to 200–2500 m/z. A tetrahydrofuran (THF) solution of 2,5-dihydroxybenzoic acid (DHB) was used as a matrix and a counter-ion solution of sodium trifluoroacetate (NaTFA). The obtained spectra were analyzed with FlexAnalysis version 2.2 from Bruker Daltonics.
Fourier-transform infrared spectroscopy (FTIR) and real-time FTIR. The Fourier-transform infrared (FTIR) and real-time Fourier-transform infrared (RT-FTIR) analysis were performed using a Perkin-Elmer Spectrum 2000 FTIR instrument (Norwalk, CT) equipped with a single reflection (attenuated total reflection (ATR)) accessory unit (Golden Gate) from Graseby Specac LTD (Kent, England) and a triglycine sulphate (TGS) detector. Spectra were based on 16 scans averaged at 4.0 cm−1 resolution range of 600–4000 cm−1. The obtained data were analyzed with Spectrum software from Perkin-Elmer. For RT-FTIR a homogenous sample containing reactants and photoinitiator was placed directly on the ATR crystal. The solvent was allowed to evaporate (followed by FTIR spectra) before a quartz crystal was put on the sample. The data were collected at an optimized rate of 1 scan per 1.67 s with a resolution of 4.0 cm−1 using TimeBase® software from Perkin-Elmer.
Fourier-transform raman spectroscopy (FT-Raman). FT-Raman measurements were performed on a Perkin-Elmer Spectrum 2000 NIR FT-Raman instrument equipped with an Nd-YAG laser. The spectra were based on 32 scans with a laser power of 500–1000 mW.
Contact angle measurements. Static contact angle measurements were performed on a CAM 200 contact-angle meter (KSV Instruments, Helsinki, Finland) equipped with a Basler A602f camera. The sessile-drop technique was used and a 3 μL water droplet was dispensed on the surface using an automatic dispenser. MilliQ water droplets were recorded on 5 different spots of the sample surface and OneAttention software was used to calculate the contact angles using Young-Laplace fitting.
UV-light source. The UV-light source for the UV experiments was obtained from Hamamatsu L5662 equipped with a standard medium-pressure 200 W L6722-01 Hg–Xe lamp. The UV intensity was measured using a Hamamatsu UV-light power meter (model C6080-03) calibrated for the main emission line centered at 365 nm. The intensity of the lamp was measured to approximately 50 mW cm−2.
Procedures
Retrieval of the fatty acids EFA and EMO. The full procedures of the retrieval of the different fatty acids can be found in literature.43 EFA was retrieved from outer birch bark by alkaline hydrolysis and purified by recrystallization from toluene. Yield: 60–70%. ESI Fig. 1† shows both 1H NMR and FTIR of EFA. 1H NMR (400 MHz, CDCl3, δ): 3.65 (2H, t, –CH2–OH, J = 6.59 Hz), 2.90 (2H, bs, –CH–O–CH–epoxide), 2.35 (2H, t, –CH2–CO–OH, J = 7.45 Hz), 1.66–1.26 (26H, m, –CH2–).EMO was retrieved by methanolysis at 60 °C from epoxidized linseed oil. The obtained methyl esters were first extracted in n-heptane/deionized water (1
:
1) 4 times and then purified by column chromatography in n-heptane/EtOAc gradient solution. 1H NMR (400 MHz, CDCl3, δ): 3.66 (3H, s, –O–CH3), 2.89 (2H, bs, –CH–O–CH–epoxide), 2.30 (2H, t, –CH2–CO–, J = 7.5 Hz), 1.62 (2H, p, –CH2–CH2–CH2–CO–, J = 7.1 Hz), 1.54–1.20 (26H, m, –CH2–aliphatic), 0.90 (3H, t, CH3–, J = 6.7 Hz).
The other methyl esters after methanolysis were methyl stearate, epoxidized methyl linoleate and epoxidized methyl linolenate. These fatty acids were not used in this study.
Enzyme-catalyzed synthesis of the epoxy–thiol dual-functional oligomer based on EFA. The oligomer that was synthesized was targeted with degree of polymerization (DP) of 3. A synthetic pathway is shown in Scheme 1. EFA (213 mg, 0.68 mmol), 11-mercaptoundecanol (94.6 mg, 0.45 mmol) and dimethyl adipate (37.3 μL, 0.23 mmol) were mixed with 2 mL toluene in a 10 mL round-bottom flask at 60 °C. Molecular sieves (4 Å) were added to remove water and methanol from the system. The reaction was started by the addition of 35.4 mg of Novozyme 435 (10 wt% of the sum of the monomers). The reaction was monitored by 1H NMR and left for approximately 8 h. The reaction was stopped by filtering the mixture through a glass Pasteur pipette containing cotton in the tip. The toluene was evaporated using rotavapor. Fig. 1 shows the 1H NMR of the oligomer. The obtained oligomer was stored in a refrigerator at 4 °C and was used without further purification.
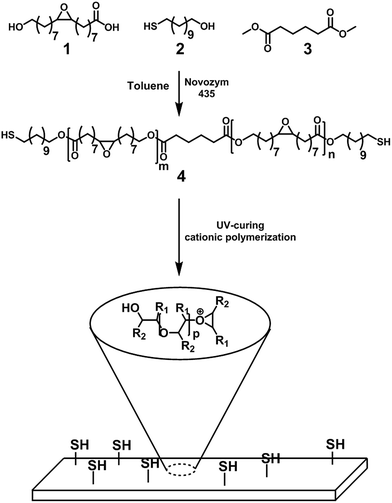 |
| Scheme 1 Schematic overview of the synthesis of the epoxy–thiol dual-functional oligomer (4) based on EFA (1). | |
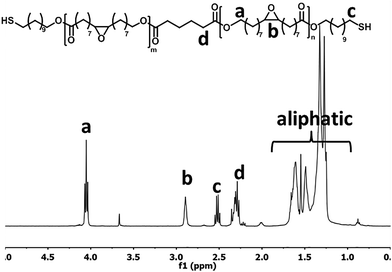 |
| Fig. 1 1H NMR of the synthesised EFA based oligomer (4). | |
General procedure for UV-initiated experiments. The photoinitiators chosen for this study were UVAcure 1600 and benzophenone. Photoinitiator solutions were made in vials totally covered with aluminum foil. The solutions were stored in a refrigerator at 4 °C. The concentrations of the solutions were 20 mg mL−1 and the solvent used was CHCl3. The weighing of the reactants for the different experiments occurred in a dark room and the vials used were totally covered with aluminum to minimize light exposure.
Model experiment A: secondary epoxide in presence of thiol. Monomers EMO (25 mg, 0.08 mmol) and 11-mercaptoundecanol (16.40 mg, 0.08 mmol) were weighed into a vial and 83 mL of UVAcure 1600 photoinitiator solution were added to the mixture. The mixture was then casted on a glass substrate using a Pasteur pipette and the solvent was allowed evaporate completely. A quartz microscope slide was then put on the sample prior to UV-light exposure. A control experiment with only EMO and initiator was also made following the same procedure. Both experiments proceeded for 20 min. For the results please see ESI.†
Model experiment B: aliphatic vinyl ether in the presence of thiol. Monomers HMVE (25 mg, 0.17 mmol) and 11-mercaptoundecanol (35 mg, 0.17 mmol) were weighed into a vial and 120 mL of benzophenone photoinitiator solution were added to the mixture. The mixture was then casted on a glass substrate using a Pasteur pipette and the solvent was allowed evaporate completely. A quartz microscope slide was then put on the sample prior to UV-light exposure. A control experiment with only HMVE and initiator was also made following the same procedure. The duration of the experiments were 20 min. For the results please see ESI.†
Procedures of the crosslinking of the oligomers. Approximately 25 mg of the enzymatically synthesized oligomer (4) was weighed and mixed with 50 μL of the UVAcure 1600 photoinitiator solution prior to homogenization. The mixture was then casted on a glass substrate using a Pasteur pipette and the solvent was let evaporate completely. A quartz microscope slide was then put on the sample prior to UV-light exposure. For UV initiated RT-FTIR measurements the casting of the reactant mixture was directly on the ATR diamond crystal following the same procedure before UV-light exposure. The duration of the experiments were 20 min.
Surface thiol test with Elman's reagent. DTNB was dissolved in an aqueous phosphate buffer (1 M, pH 7.0) to a concentration of 3.0 mM. The solution was made in a vial totally covered with aluminum foil. The solution was stored in a refrigerator at 4 °C and was taken out only before use. Crosslinked films were immersed in a vial containing 1 mL of the DTNB solution. The vial was vortexed for 5 min at room temperature.
Surface modification procedure. Crosslinked films were made on glass substrates from the synthesized oligomers. Photoinitiator benzophenone (1 mg, 6 μmol) was mixed with HMVE (25 mg, 0.17 mmol) in a vial and homogenized. Approximately 50 μL were taken from the mixture and put on the surface of the crosslinked films. Quartz microscope slides were put on the samples prior to UV-light exposure. After 20 min of UV-light exposure the microscope slides were removed and the surface of each film was washed three times with de-ionized water and was left to dry in air.
Results and discussion
Monomers retrieved from natural resources
Natural resources were used in order to isolate the fatty acid EFA. Birch bark was subjected to alkali hydrolysis at 100 °C. The alkali hydrolysis breaks the ester bonds in the suberin structure and a mixture of different free fatty acids was obtained. The solution was then acidified to pH 5.7 to protonate the carboxylic group of EFA. Subsequently a precipitate occurred. However, it is not recommended to acidify below pH 5.5 since the epoxide could ring-open and form 9,10,18-trihydroxyoctadecanoic acid.44 In a last step EFA was recrystallized from toluene. According to literature outer birch bark suberin structure contains 99 mg EFA per g dry outer birch bark.45 Comparing with the yield from literature our yield was 60–70%. The EFA monomer was confirmed by 1H NMR and FTIR (ESI Fig. 1†).
Synthesis of the multifunctional oligomer based on EFA
The purified EFA was used for an enzyme-catalyzed polymerization utilizing CALB as the catalyst and 11-mercaptoundecanol acid as end-capper. The one-pot enzymatic catalysis occurred for 8 h and reached conversions of >99% for the OH-groups in EFA and the 11-mercaptoundecanol. NMR analysis confirmed the structure of the product (Fig. 1). Furthermore, NMR showed that the DP was 2.5 and the Mw was 1189.85 g mol−1. The functional groups were unaffected during the synthesis (peaks b and c).
The MALDI-TOF-MS spectrum shows the main component with end-cappers on both sides, while showing a minor amount of the oligomer with end-capping only on one side (Fig. 2). This is also confirmed by 1H NMR as there is a CH3-peak at about 3.6 ppm (Fig. 1). The mass difference between the major peaks of the MALDI-TOF-MS spectrum is 296 Da which corresponds to an EFA-repeating-unit. A reaction in bulk at 85 °C resulted in an insoluble network. At this temperature the combination of a carboxylic acid, a thiol and epoxide groups led to reactions creating a network.
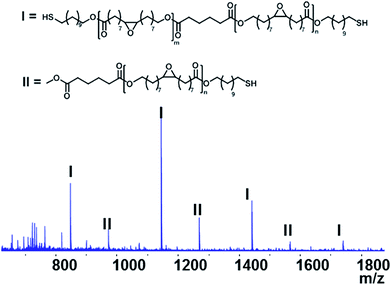 |
| Fig. 2 MALDI-TOF-MS of the synthesized EFA based oligomer (4). | |
Network formation and polymerization performance of the oligomers
The synthesized oligomer contained two functional groups, epoxides and thiols as confirmed by 1H NMR and MALDI (Fig. 1 and 2). In order to preserve the thiol groups, cationic polymerization initiated by UVAcure 1600 was chosen to selectively propagate the epoxides as indicated by model experiment A. The polymerization performance was followed by RT-FTIR (Fig. 3). The epoxide functional group at 827 cm−1 disappeared over time while the peak at 1073 cm−1 increased representing the formation of ether bonds. Furthermore, the result indicated that it was a moderately fast reaction. The epoxide was fully converted after approximately 10 min of UV-light exposure. A transparent film was obtained after the reaction. The thiol group has low absorbance in FTIR mode hence, FT Raman analysis was made in order to confirm the thiol groups. Fig. 4 shows FT Raman spectra of before and after UV-curing. The figure shows that the thiol groups at 2570 cm−1 were still intact after UV-curing. Furthermore, the FT Raman analysis of the oligomer further confirms the 1H NMR results, both analysis methods shows that the thiol groups were still intact after enzyme catalysis. However, FT Raman analysis provided information about the bulk properties of the film. In order to distinguish if there were any thiol groups on the surface of the film, Ellman's reagent was used. During such a test Ellman's reagent (DTNB) reacts with aliphatic thiol compounds and a highly yellow colored anion (p-nitrophenol anion) is produced.46 During this study DTNB was dissolved in an aqueous phosphate buffer solution (pH 7) and was used to confirm any presence of thiol groups. Crosslinked films were put in a vial with 1 mL DTNB solution. After approximately 1 min the color of the solution changed to yellow. Since the bulk of the film is hydrophobic due to ether bonds formed from the epoxide reaction, it was hypothesized that the reaction of the DTNB with thiol producing the highly yellow colored p-nitrophenol occurred on the surface of the film.
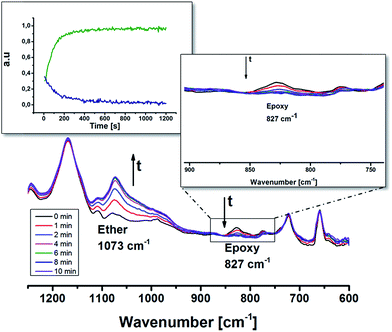 |
| Fig. 3 UV curing of EFA-DMA-SH (4) followed by RT-FTIR. | |
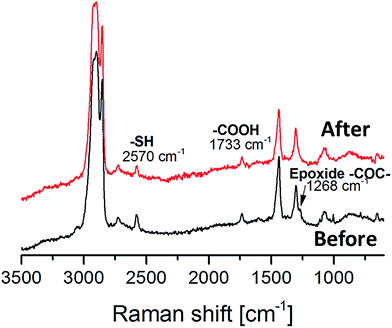 |
| Fig. 4 FT Raman before and after UV curing of compound 4. | |
Modification of surface properties
As previously mentioned, the FT Raman analysis and Ellman's reagent test indicated that the thiol groups were unharmed during UV-light exposure. Model experiment B proved that with HMVE no homopolymerization occurs when initiated by benzophenone. Furthermore, when thiol groups were presented to the reaction mixture HMVE reacted with the thiol (ESI Fig. 4†). To further investigate if the thiol groups are available on the surface of the films a thiol-ene reaction with HMVE was proposed. Fig. 5 shows the contact angle of a water droplet on a surface of crosslinked film before and after thiol-ene reaction. The measurement revealed that the surface properties of the film changed after the reaction. Moreover, the surface became more hydrophilic since the thiol groups were exchanged with hydroxyl groups on the surface. This further confirms the hypothesis that the thiol groups were accessible on the surface of the films.
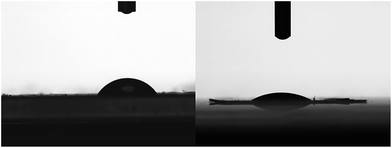 |
| Fig. 5 Contact angle before (to the left) and after (to the right) modifying the surface. | |
Conclusions
The present study demonstrates a chemo-enzymatic route to make multifunctional thermoset resins with both epoxy and thiol functionalities. The resins can be readily polymerized to form thermoset films with retained thiol functionalities active for subsequent post surface modifications. A thiol–epoxy functional oligomer was synthesized by utilizing a chemo-enzymatic route. The epoxy monomer was bio-based and extracted from outer birch bark (Betula pendula). By utilizing the enzyme CALB it was possible to selectively add the thiol group to the bio-based epoxide monomer under mild reaction conditions without harming the epoxide. Furthermore, it was possible to crosslink the synthesized thiol–epoxy oligomer by selectively reacting the epoxides. The remaining thiols where reacted to confirm their activity.
Conflicts of interest
There are no conflicts to declare.
Acknowledgements
This work was supported by the Swedish research council, FORMAS (Grant Number 211-2013-70), Lantmännen Research Foundation and the European Union's Seventh Framework Programme for research, technological development and demonstration under grant agreement no. 289253.
Notes and references
- J. H. Clark, J. Chem. Technol. Biotechnol., 2007, 82, 603–609 CrossRef CAS.
- B. Kamm and M. Kamm, Adv. Biochem. Eng./Biotechnol., 2007, 105, 175–204 CrossRef CAS PubMed.
- Y. Arima and H. Iwata, Biomaterials, 2007, 28, 3074–3082 CrossRef CAS PubMed.
- B. Ellis, Chemistry and technology of epoxy resins, Springer, 1993 Search PubMed.
- M. A. Gauthier, M. I. Gibson and H. A. Klok, Angew. Chem., Int. Ed. Engl., 2009, 48, 48–58 CrossRef CAS PubMed.
- S. Mongkhontreerat, K. Oberg, L. Erixon, P. Lowenhielm, A. Hult and M. Malkoch, J. Mater. Chem. A, 2013, 1, 13732–13737 CAS.
- V. Granskog, O. C. J. Andren, Y. K. Cai, M. Gonzalez-Granillo, L. Fellander-Tsai, H. von Holst, L. A. Haldosen and M. Malkoch, Adv. Funct. Mater., 2015, 25, 6596–6605 CrossRef CAS.
- Z. T. Cygan, J. T. Cabral, K. L. Beers and E. J. Amis, Langmuir, 2005, 21, 3629–3634 CrossRef CAS PubMed.
- N. Vasylieva, B. Barnych, A. Meillerd, C. Maucler, L. Pollegioni, J. S. Lin, D. Barbier and S. Marinesco, Biosens. Bioelectron., 2011, 26, 3993–4000 CrossRef CAS PubMed.
- M. A. R. Meier, J. O. Metzger and U. S. Schubert, Chem. Soc. Rev., 2007, 36, 1788–1802 RSC.
- J. Graca, Front. Chem., 2015, 3, 62 Search PubMed.
- X. Pan, P. Sengupta and D. C. Webster, Biomacromolecules, 2011, 12, 2416–2428 CrossRef CAS PubMed.
- K. Huang, P. Zhang, J. W. Zhang, S. H. Li, M. Li, J. L. Xia and Y. H. Zhou, Green Chem., 2013, 15, 2466–2475 RSC.
- S. Torron, S. Semlitsch, M. Martinelle and M. Johansson, Biomacromolecules, 2016, 17, 4003–4010 CrossRef CAS PubMed.
- A. Gandini, C. Pascoal and A. J. D. Silvestre, Prog. Polym. Sci., 2006, 31, 878–892 CrossRef CAS.
- R. Ekman, Holzforschung, 1983, 37, 205–211 CrossRef CAS.
- J. V. Crivello, Adv. Polym. Sci., 1984, 62, 1–48 CrossRef CAS.
- J. V. Crivello, J. Polym. Sci., Part A: Polym. Chem., 1999, 37, 4241–4254 CrossRef CAS.
- C. Decker and K. Moussa, J. Polym. Sci., Part C: Polym. Lett., 1990, 28, 3429–3443 CrossRef CAS.
- C. Decker, T. T. N. Viet and H. P. Thi, Polym. Int., 2001, 50, 986–997 CrossRef CAS.
- J. M. Barton, in Epoxy Resins and Composites I, Springer Berlin Heidelberg, Berlin, Heidelberg, 1985, pp. 111–154, DOI:10.1007/3-540-15546-5_5.
- B. A. Rozenberg, in Epoxy Resins and Composites II, ed. K. Dušek, Springer Berlin Heidelberg, Berlin, Heidelberg, 1986, pp. 113–165, DOI:10.1007/BFb0017916.
- C. E. Hoyle and C. N. Bowman, Angew. Chem., Int. Ed., 2010, 49, 1540–1573 CrossRef CAS PubMed.
- L. M. de Espinosa and M. A. R. Meier, Eur. Polym. J., 2011, 47, 837–852 CrossRef.
- M. Claudino, M. Jonsson and M. Johansson, RSC Adv., 2013, 3, 11021–11034 RSC.
- G. J. Chen, S. Amajjahe and M. H. Stenzel, Chem. Commun., 2009, 10, 1198–1200 RSC.
- A. B. Lowe, Polym. Chem., 2010, 1, 17–36 RSC.
- M. Liras, I. Quijada-Garrido, M. Palacios-Cuesta, S. Munoz-Durieux and O. Garcia, Polym. Chem., 2014, 5, 433–442 RSC.
- G. Carrot, J. Hilborn, J. L. Hedrick and M. Trollsas, Macromolecules, 1999, 32, 5171–5173 CrossRef CAS.
- A. Southan, E. Hoch, V. Schonhaar, K. Borchers, C. Schuh, M. Muller, M. Bach and G. E. M. Tovar, Polym. Chem., 2014, 5, 5350–5359 RSC.
- S. Shoda, H. Uyama, J. Kadokawa, S. Kimura and S. Kobayashi, Chem. Rev., 2016, 116, 2307–2413 CrossRef CAS PubMed.
- S. Kobayashi, Proc. Jpn. Acad., Ser. B, 2010, 86, 338–365 CrossRef CAS PubMed.
- R. A. Gross, M. Ganesh and W. H. Lu, Trends Biotechnol., 2010, 28, 435–443 CrossRef CAS PubMed.
- B. Yeniad, H. Naik and A. Heise, Biofunctionalization of Polymers and Their Applications, 2011, vol. 125, pp. 69–95 Search PubMed.
- C. Hedfors, E. Ostmark, E. Malmstrom, K. Hult and M. Martinelle, Macromolecules, 2005, 38, 647–649 CrossRef CAS.
- M. Finnveden, S. Nameer, M. Johansson and M. Martinelle, Macromol. Chem. Phys., 2016, 217, 1335–1341 CrossRef CAS.
- M. Takwa, N. Simpson, E. Malmstrom, K. Hult and M. Martinelle, Macromol. Rapid Commun., 2006, 27, 1932–1936 CrossRef CAS.
- C. Hedfors, K. Hult and M. Martinelle, J. Mol. Catal. B: Enzym., 2010, 66, 120–123 CrossRef CAS.
- A. Olsson, M. Lindstrom and T. Iversen, Biomacromolecules, 2007, 8, 757–760 CrossRef CAS PubMed.
- S. Torron, S. Semlitsch, M. Martinelle and M. Johansson, Macromol. Chem. Phys., 2014, 215, 2198–2206 CrossRef CAS.
- S. Torron and M. Johansson, J. Polym. Sci., Part A: Polym. Chem., 2015, 53, 2258–2266 CrossRef CAS.
- S. Semlitsch, S. Torron, M. Johansson and M. Martinelle, Green Chem., 2016, 4003–4010 Search PubMed.
- S. Nameer and M. Johansson, J. Coat. Technol. Res., 2017, 14, 757–765 CrossRef CAS.
- P. A. Krasutsky, Nat. Prod. Rep., 2006, 23, 919–942 RSC.
- P. C. R. O. Pinto, A. R. Sousa, A. J. D. Silvestre, C. P. Neto, A. Gandini, C. Eckerman and B. Holmbom, Ind. Crops Prod., 2009, 29, 126–132 CrossRef CAS.
- G. L. Ellman, Arch. Biochem. Biophys., 1958, 74, 443–450 CrossRef CAS PubMed.
Footnotes |
† Electronic supplementary information (ESI) available: ESI One-pot enzyme-catalyzed synthesis of dual-functional polyester macromers towards surface active hydrophobic films. See DOI: 10.1039/c7ra09828f |
‡ These authors contributed equally to the work. |
|
This journal is © The Royal Society of Chemistry 2017 |