DOI:
10.1039/C7RA08138C
(Paper)
RSC Adv., 2017,
7, 41869-41879
Synthesis, cytotoxic evaluation and DNA binding study of 9-fluoro-6H-indolo[2,3-b]quinoxaline derivatives†
Received
24th July 2017
, Accepted 21st August 2017
First published on 29th August 2017
Abstract
A series of novel 9-fluoro-6H-indolo[2,3-b]quinoxaline derivatives as antitumor agents were synthesized and studied. The designed compounds feature positive charges both at the 11-N position of the aromatic scaffold and the side-chain alkylamino group. The corresponding in vitro antitumor activities were evaluated against MCF-7, HeLa and A549 cancer cell lines and the DNA binding properties of these compounds were characterized by UV-vis, fluorescence, and circular dichroism (CD) spectroscopy as well as thermal denaturation. The obtained results indicate that the dicationic quaternary ammonium salt derivatives of 9-fluoro-6H-indolo[2,3-b]quinoxaline may serve as intercalators with increased DNA binding affinity. Furthermore, both the incorporation of fluorine, and alkyl amino side chains and the introduction of a positive charge at the 11-N position of the aromatic scaffold was shown to be responsible for their antitumor activity and improved DNA binding ability. Taken in concert, these findings may be valuable in the understanding of the antitumor effect of these compounds and may further provide important information for the future optimization of the DNA binding properties of this drug type.
1. Introduction
As the second leading cause of death worldwide, cancer accounts for nearly one in four of all mortality cases. It is estimated that about 10 million new cancer cases are diagnosed every year, causing a major health concern in both the developing and the developed countries.1 Despite advances in surgery and radiation therapy, chemotherapy still plays a crucial role in cancer treatment. However, the overall chemotherapeutic success is still limited by several drawbacks, including inadequate drug concentrations at the tumor site, undesirable toxicity, poor tumor cell selectivity over normal cells, and the occurrence of multiple drug resistance.2,3 Therefore, the discovery and development of selective, efficient, and safe drug types for anticancer chemotherapy remains a critical goal with high priority in medical research.
Modification of existing active drug candidates, known as analogue design, is one of the most widely accepted approaches in drug discovery for the development of new drugs and improved therapeutic properties.4–9 Natural alkaloids and alkaloid derivatives, such as indoloquinoline alkaloid cryptolepine and pyridocarbazole alkaloid ellipticine, have attracted considerable attention due to their interesting biological activities demonstrated by both in vitro and in vivo studies. Their antitumor, mutagenic and cytotoxic activities were found to be due to a variety of mechanisms, including (i) DNA intercalation, (ii) inhibition of DNA topoisomerase II activity, and others.10 Unfortunately, toxicity issues have more or less limited the overall success of these drugs in pharmaceutical industry.11
In recent years, 6H-indolo[2,3-b]quinoxaline (cf. Fig. 1) have received much attention due to its important pharmacological activities, including antiviral (herpes simplex virus type 1 (HSV-1), cytomegalovirus (CMV), varicellazoster virus (VZV), etc.), cytotoxic and multidrug resistant (MDR) modulating activities, acting on ATP binding cassette transporters (ABC-transporters).12,13 6H-Indolo[2,3-b]quinoxaline combines the structural features of indoles and quinoxalines and may be regarded as an aza-analogue of the cytotoxic agents cryptolepine and ellipticine.14 The planar structure of this compound aids in the intercalation of DNA which in turn is responsible for biological activities such as cytotoxicity, antiviral activity, etc.12,13 The compound class has been well-studied and well-described in the literature, primarily by Bergman and co-workers.15–18 In recent years, several analogues of 6H-indolo[2,3-b]quinoxaline have been synthesized, e.g. B-220 (2,3-dimethyl-6-(2-dimethylaminoethyl)-6H-indolo[2,3-b]quinoxaline, Fig. 1), NCA0424, and others. Importantly, these compounds exhibited high antiviral activity and selective anticancer activity (Fig. 1).19–24 Hence, the indolo[2,3-b]quinoxaline nucleus was considered as a template for the design and development of DNA intercalating agents with cytotoxic activities in this study.
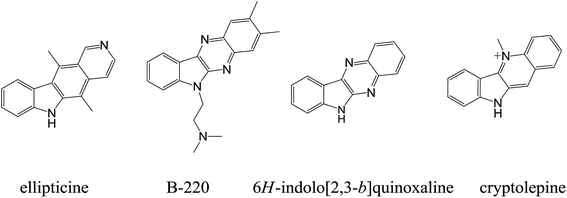 |
| Fig. 1 The structure of ellipticine, B-220, indolo[2,3-b]quinoxaline and cryptolepine. | |
Owing to the special nature of fluorine as a functional group, the incorporation of fluorine usually conveys a variety of properties to certain pharmaceuticals and includes enhanced binding interactions, metabolic stability, changes in physical properties, and selective reactivities.25 Today, fluorine is present in up to 30% of pharmaceuticals. More recently, Karki et al. reported that 9-fluoro-6-(4-methylbenzyl)-6H-indolo[2,3-b]quinoxaline exhibited a potent anticancer activity against various human tumor cell lines.26 Meanwhile, it is generally appreciated that the transformation of neutral chromophores into their cationic derivatives usually enhances their affinity for DNA, thus increasing the overall antitumor activity.27,28 This is also the case for elliptinium, a cationic derivative of 9-methoxyellipiticine.29,30 Apart from the contribution of planar polycyclic aromatic systems, higher DNA binding affinity and biological activities may also be affected by the introduction of substituted groups such as amino side chains,11,31–34 sugars,35,36 or heterocycles37,38 to the chromophore. Furthermore, a cationic amino side chain may play an important role in the increase of the DNA binding affinity and biological activities due to their electrostatic interaction with the phosphate moieties of DNA. Moreover, chains of varying length, different polarity, structural rigidity, charge and steric bulk may also result in different DNA binding affinities.39
This work was designed to synthesize and study a group of novel 9-fluoro-6H-indolo[2,3-b]quinoxaline derivatives with improved DNA binding affinity and antitumor activity by attaching various alkyl amino side chains to the chromophore and introducing multiple positive charges via selective methylation at the nitrogens of the quinoxaline ring and the terminal amino-group. The DNA binding affinity of the resulting 9-fluoro-6H-indolo[2,3-b]quinoxaline derivatives was evaluated based on interaction with calf thymus (CT) DNA and the cytotoxicity of the compounds was assessed on three types of tumor cell lines, human mammary cancer (MCF-7) cells, human cervical carcinoma (HeLa) cells, and human lung cancer (A549) cells. Furthermore, the molecular structure and bioactivity relationships of the compounds were studied as discussed in subsequent sections.
2. Results and discussion
2.1. Synthesis and characterization
The synthesis of the quaternary mono- or dicationic indoloquinoxaline derivatives was carried out as depicted in Scheme 1. Isatin was obtained from commercial sources. 5-Fluoroisatin was synthesized according to a published procedure.40 9-Fluoro-6H-indolo[2,3-b]quinoxaline (2a) and 6H-indolo[2,3-b]quinoxaline (2d) were prepared by condensation of 5-fluoroisatin (or isatin) with 1,2-phenylenediamine in glacial acetic acid according to a procedure reported previously.41 The key intermediates of 6-bromoalkyl indolo[2,3-b]quinoxaline 3 were synthesized via alkylation by the use of excess dibromoalkane in tetrahydrofuran (THF) in the presence of potassium hydroxide. Amination using the corresponding cyclic secondary amines access lead to aminoalkyl-indoloquinoxalines 4. The methylation of 4 with iodomethane in different solvents (acetone or tetramethylenesulfone) selectively afforded the mono- or dicationic fluorinated indoloquinoxaline derivatives in excellent yield.42
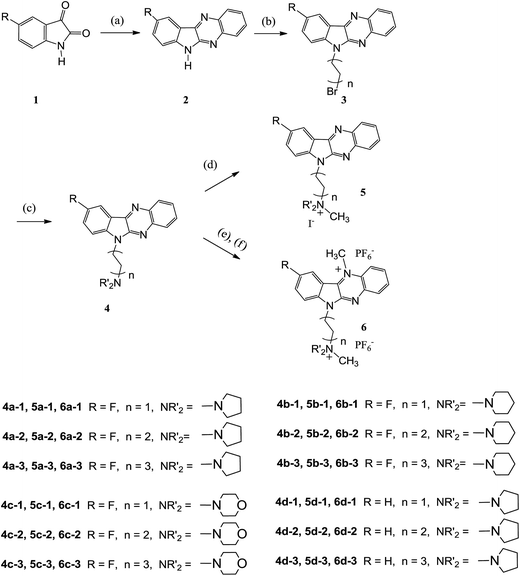 |
| Scheme 1 Synthesis of 9-fluoro-6H-indolo[2,3-b]quinoxaline derivatives. Reaction conditions: (a) o-phenylenediamine, acetic acid, reflux, 5 h; (b) dibromoalkane, KOH, THF, reflux, 6 h; (c) cyclic secondary amine, THF, reflux, N2; (d) CH3I, acetone; (e) CH3I, tetramethylenesulfone, 55 °C, overnight: (f) KPF6, H2O, 45 °C, 2 h. | |
The structures of the target compounds were characterized by IR spectroscopy, mass-spectrometry, NMR-spectroscopy, and single crystal X-ray diffraction analysis of the precursor of compound 6b-1. Vibrations of aromatic C–H bonds were observed in the IR spectra of the obtained compounds at 3096–3035 cm−1, with aliphatic signals appearing at 2980–2753 cm−1. Double bond vibrations of heterocyclic fragments exhibited a band at 1621–1450 cm−1. The bands at 1236–1058 cm−1 corresponded to CH2–N vibrations. The mass spectra displayed the correct molecular ion peaks and the measured high resolution (HRMS) data was also in good agreement with the calculated values.
Two “parts” corresponding to the aromatic and aliphatic moieties of the molecules were observed in 1H NMR-spectra of the synthesized indoloquinoxalines derivatives. The spectral ranges for the aliphatic regions were different due to the presence of terminal amino groups. In the 13C NMR spectrum of the 9-fluoro-6H-indolo[2,3-b]quinoxaline derivatives, the carbons in the fluorinated aromatic ring resonated as doublets due to spin–spin coupling with the fluorine atom. The carbon–fluorine spin–spin coupling constants of 1JCF, 2JCF, 3JCF and 4JCF have been determined as 234.0–240.0 Hz, 22.0–25.5 Hz, 9.0–10.5 Hz and 3.0–4.5 Hz, respectively.
2.2. Structural studies
An X-ray crystal structure determination was performed to confirm the structure of the precursor of 6b-1 in the solid phase. A summary of data collection and refinement parameters is provided in Table 1. Based on crystallographic data, the molecular structure of the precursor of compound 6b-1 is shown in Fig. 2. The molecule adopted an almost planar configuration with the piperidinyl ring in the expected chair conformation. The dihedral angle between the two planes intersecting along the N,N-axis in N,N-dihydropyrazine was 0.0038° (109). The position of methylation on the nucleus was unambiguously observed at N3 instead of N2. Another methylated position was found at N4. The bond length of N3–C23 was 1.4738 Å (60), while the bond length of N4–C22 was 1.510 Å (6) and the bond length of C4–F1 was 1.3761 Å (43) (Table 2).
Table 1 Crystallographic data for the precursor of 6b-1
Formula |
C23H29FI2N4O |
Fw |
650.30 |
T (K) |
296(2) |
Cryst syst |
Triclinic |
Space group |
P![[1 with combining macron]](https://www.rsc.org/images/entities/char_0031_0304.gif) |
a (Å) |
7.840(3) |
b (Å) |
12.067(5) |
c (Å) |
14.220(6) |
V (nm3) |
1312.3(9) |
Z |
2 |
Dc (Mg m−3) |
1.646 |
F (000) |
636 |
Cryst dimens (mm) |
0.35 × 0.15 × 0.13 |
θ Range (deg) |
2.18–26.00 |
hkl ranges |
−9 < h < 7 |
−14 < k < 14 |
−17 < l < 15 |
Data/parameters |
5085/290 |
Goodness-of-fit on F2 |
1.072 |
Final R indices [I > 2σ(I)] |
R1 = 0.0342 |
wR2 = 0.0831 |
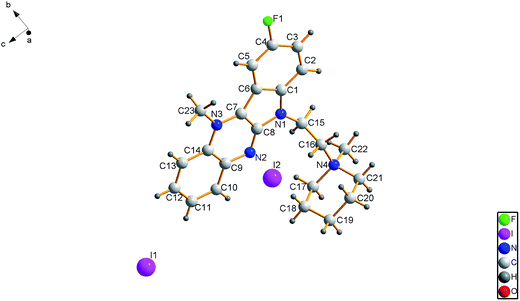 |
| Fig. 2 ORTEP view of the precursor of 6b-1. | |
Table 2 Selected bond lengths and angles for the precursor of 6b-1
Length/Å or angle/° |
F(1)–C(4) |
1.376(5) |
C(5)–C(4)–F(1) |
118.2(4) |
N(1)–C(8) |
2.281(4) |
F(1)–C(4)–C(3) |
117.1(4) |
N(2)–C(8) |
1.310(5) |
C(8)–N(1)–C(1) |
108.9(3) |
N(2)–C(9) |
1.360(5) |
C(8)–N(2)–C(9) |
114.5(3) |
N(3)–C(7) |
1.343(4) |
C(7)–N(3)–C(23) |
119.3(3) |
N(3)–C(14) |
1.399(5) |
C(7)–N(3)–C(14) |
119.0(3) |
N(3)–C(23) |
1.473(5) |
C(14)–N(3)–C(23) |
121.7(3) |
N(4)–C(22) |
1.510(5) |
C(17)–N(4)–C(16) |
112.6(3) |
N(4)–C(17) |
1.520(5) |
C(22)–N(4)–C(21) |
108.3(3) |
N(4)–C(16) |
1.521(5) |
C(17)–N(4)–C(21) |
110.1(3) |
N(4)–C(21) |
1.526(5) |
C(22)–N(4)–C(16) |
109.6(3) |
2.3. Cytotoxicity studies
The antitumor activities of aminoalkyl-indoloquinoxalines 4 as well as the quaternary mono- and dicationic indoloquinoxaline derivatives 5 and 6 were evaluated in vitro against tumor cell lines MCF-7, HeLa and A549, respectively, as listed in Tables 3–5. 9-Fluoro-6H-indolo[2,3-b]quinoxaline (FIQX) and cisplatin were used as positive controls. According to the data shown in Tables 3–5, most of the synthesized quaternary dicationic FIQX derivatives were found to be more active than the corresponding quaternary monocationic FIQX derivatives and the neutral 6H-indolo-[2,3-b]quinoxaline derivatives.
Table 3 The cytotoxicity of series of compounds (4) against MCF-7, Hela and A549
Compounds |
IC50 (μM)a |
MCF-7 |
Hela |
A549 |
IC50 values were calculated from the linear regression of the dose–log response curves. Values are mean ± S.D. of at least three independent experiments. Ref. 43. Ref. 44. Ref. 45. |
4a-1 |
3.68 ± 0.36 |
5.69 ± 0.48 |
4.32 ± 0.57 |
4a-2 |
8.11 ± 0.52 |
7.65 ± 1.24 |
6.36 ± 0.88 |
4a-3 |
4.76 ± 0.31 |
4.25 ± 0.59 |
4.13 ± 0.33 |
4b-1 |
45.58 ± 5.99 |
19.38 ± 2.10 |
25.70 ± 3.53 |
4b-2 |
34.33 ± 4.65 |
13.14 ± 1.46 |
9.01 ± 0.89 |
4b-3 |
28.89 ± 3.37 |
8.61 ± 1.38 |
10.23 ± 1.05 |
4c-1 |
42.68 ± 4.76 |
27.72 ± 3.48 |
53.41 ± 6.64 |
4c-2 |
11.85 ± 0.98 |
15.76 ± 1.04 |
15.00 ± 2.21 |
4c-3 |
5.48 ± 0.43 |
15.49 ± 2.11 |
19.70 ± 1.98 |
4d-1 |
3.69 ± 0.32 |
6.63 ± 0.55 |
4.36 ± 0.29 |
4d-2 |
10.50 ± 1.76 |
16.97 ± 2.10 |
7.45 ± 0.68 |
4d-3 |
6.44 ± 0.65 |
9.58 ± 1.03 |
3.38 ± 0.44 |
FIQX |
15.44 ± 1.46 |
20.83 ± 2.22 |
24.99 ± 3.19 |
Cisplatin |
8.25 ± 0.53b |
11.06 ± 1.08c |
8.61 ± 0.39d |
Table 4 The cytotoxicity of series of compounds (5) against MCF-7, Hela and A549
Compounds |
IC50 (μM)a |
MCF-7 |
Hela |
A549 |
IC50 values were calculated from the linear regression of the dose–log response curves. Values are mean ± S.D. of at least three independent experiments. Ref. 43. Ref. 44. Ref. 45. |
5a-1 |
22.31 ± 3.12 |
22.64 ± 2.43 |
20.28 ± 3.04 |
5a-2 |
31.57 ± 3.67 |
23.86 ± 2.75 |
75.99 ± 6.98 |
5a-3 |
16.12 ± 1.31 |
30.43 ± 3.66 |
17.82 ± 2.15 |
5b-1 |
29.65 ± 3.34 |
22.88 ± 4.02 |
17.83 ± 1.60 |
5b-2 |
16.58 ± 0.89 |
24.96 ± 3.57 |
16.78 ± 2.31 |
5b-3 |
12.45 ± 0.81 |
27.28 ± 2.64 |
16.25 ± 1.42 |
5c-1 |
12.92 ± 1.34 |
18.09 ± 1.73 |
12.33 ± 0.51 |
5c-2 |
14.38 ± 2.21 |
30.77 ± 4.03 |
22.35 ± 2.59 |
5c-3 |
19.19 ± 1.47 |
21.62 ± 2.85 |
26.84 ± 3.07 |
5d-1 |
8.70 ± 0.82 |
8.56 ± 0.67 |
7.80 ± 1.20 |
5d-2 |
14.75 ± 2.18 |
9.21 ± 0.62 |
12.81 ± 0.97 |
5d-3 |
8.66 ± 0.42 |
10.31 ± 1.23 |
11.41 ± 0.86 |
FIQX |
15.44 ± 1.46 |
20.83 ± 2.22 |
24.99 ± 3.19 |
Cisplatin |
8.25 ± 0.53b |
11.06 ± 1.08c |
8.61 ± 0.39d |
Table 5 The cytotoxicity of series of compounds (6) against MCF-7, Hela and A549
Compounds |
IC50 (μM)a |
MCF-7 |
Hela |
A549 |
IC50 values were calculated from the linear regression of the dose–log response curves. Values are mean ± S.D. of at least three independent experiments. Ref. 43. Ref. 44. Ref. 45. |
6a-1 |
3.14 ± 0.33 |
5.05 ± 0.41 |
6.31 ± 0.70 |
6a-2 |
3.54 ± 0.26 |
3.87 ± 0.35 |
5.68 ± 0.42 |
6a-3 |
1.80 ± 0.24 |
4.22 ± 0.63 |
5.25 ± 0.39 |
6b-1 |
2.89 ± 0.38 |
4.08 ± 0.37 |
6.09 ± 0.91 |
6b-2 |
1.94 ± 0.29 |
6.04 ± 0.67 |
8.45 ± 1.28 |
6b-3 |
1.60 ± 0.31 |
3.34 ± 0.55 |
6.86 ± 1.30 |
6c-1 |
7.23 ± 1.06 |
4.73 ± 0.49 |
6.82 ± 0.88 |
6c-2 |
4.47 ± 0.57 |
10.43 ± 1.38 |
6.96 ± 0.59 |
6c-3 |
3.01 ± 0.25 |
8.31 ± 0.66 |
9.70 ± 0.74 |
6d-1 |
3.73 ± 0.44 |
22.15 ± 2.61 |
13.33 ± 1.81 |
6d-2 |
3.99 ± 0.35 |
16.12 ± 1.55 |
9.34 ± 1.02 |
6d-3 |
2.02 ± 0.30 |
9.69 ± 0.77 |
6.20 ± 0.67 |
FIQX |
15.44 ± 1.46 |
20.83 ± 2.22 |
24.99 ± 3.19 |
Cisplatin |
8.25 ± 0.53b |
11.06 ± 1.08c |
8.61 ± 0.39d |
As shown in Table 3, most of the neutral 6H-indolo[2,3-b]quinoxaline derivatives with various alkyl amino side chains showed promising cytotoxicity properties against the tested carcinoma cell lines with low IC50 values. Compounds, which contain pyrrolidine as terminal amino-group, i.e. compound 4a-1, 4a-2 and 4a-3, exhibited potent antiproliferative activities, comparable to the compounds containing piperidinyl and morpholinyl as terminal amino group. Besides, the introduction of fluorine into the aromatic core of 6H-indolo[2,3-b]quinoxaline seems to enhance the antiproliferative activities of the corresponding compounds as judged by a comparison of the fluorinated indoloquinoxaline derivatives 4a-1, 4a-2 and 4a-3 as well as the non-fluorinated compounds 4d-1, 4d-2 and 4d-3.
As for the monocationic quaternary ammonium salts of 6H-indolo[2,3-b]quinoxaline (5), the N-methylation of the terminal amino-group resulted in an apparent decrease of cytotoxicity against MCF-7, HeLa and A549 cells, except for compound 5c-1.
As for the dicationic quaternary ammonium salts of 6H-indolo[2,3-b]quinoxaline, the preliminary results demonstrated that most of the compounds were more toxic against MCF-7 than HeLa and A549 cells. Firstly, this compound series exhibited potent antiproliferative activities compared to the corresponding monocationic quaternary ammonium salts derivatives of 6H-indolo[2,3-b]quinoxaline. For example, compounds 6a-3, 6b-3 and 6c-3 exhibited an increased antiproliferative activity against MCF-7, with IC50 values of 1.80, 1.60 and 3.01 μM, while IC50 values for 5a-3, 5b-3 and 5c-3 of 16.12, 12.45 and 19.19 μM were determined, respectively. It may be concluded that N-methylation at 11-position of the indoloquinoxaline ring is essential for the cytotoxicity of 6H-indolo[2,3-b]quinoxaline derivatives. Secondly, the fluorinated dicationic quaternary ammonium salts of 6H-indolo[2,3-b]quinoxaline also showed an increased activity compared to the non-fluorinated derivatives. For example, the IC50 values of compounds 6a-1, 6a-2 and 6a-3 were 3.14, 3.54 and 1.80 μM, while the IC50 values of compounds 6d-1, 6d-2 and 6d-3 were 3.73, 3.99 and 2.02 μM, respectively. Additionally, the fluorinated dicationic quaternary ammonium salt derivatives 6a–6c exhibited higher activities than cisplain against all tested cell lines, however, compound 6c-3 proved to be an exception. Thirdly, the anti-tumor activity results against MCF-7 cells suggested that the dicationic quaternary ammonium salts of 6H-indolo[2,3-b]quinoxaline with the largest alkyl chains, such as compounds 6a-3, 6b-3, 6c-3 and 6d-3, were more active than compounds with shorter alkyl chains as linkers attached between the terminal amino group and the indolo[2,3-b]quinoxaline nucleus.
2.4. DNA-binding property
To evaluate the DNA-binding properties of the cryptolepine analogues, UV-vis absorption spectra, fluorescent spectra, and circular dichroism (CD) spectra were recorded and a thermal denaturation study of the selected compounds 5a-1, 5a-2, 6a-1, 6d-1 and 6b-3 was carried out with CT DNA.
2.4.1. UV-vis titration. UV-vis spectroscopy, based on the absorption of photons of monochromatic light, is one of the most useful techniques to study the binding affinity of a given drug to DNA. Fig. 3 shows the absorption spectra of compounds 5a-1, 5a-2, 6a-1, 6d-1 and 6b-3 in the presence of increasing amounts of CT DNA. It is worthwhile to note that a significant hypochromic effect as well as a red-shift of the absorption maxima could be observed in the spectra of compounds 5a-1, 5a-2, 6a-1, 6d-1 and 6b-3. These are common effects observed when drugs bind to DNA by either intercalation or groove binding.11 The large hypochromic effect (29–50%) and red-shift (6–11 nm) displayed by these drugs generally suggest that intercalation is the favored binding mode, but it is also conceivable that electrostatic binding between the negatively charged DNA phosphate backbone and cationic or positive end of these compounds exist at the same time.
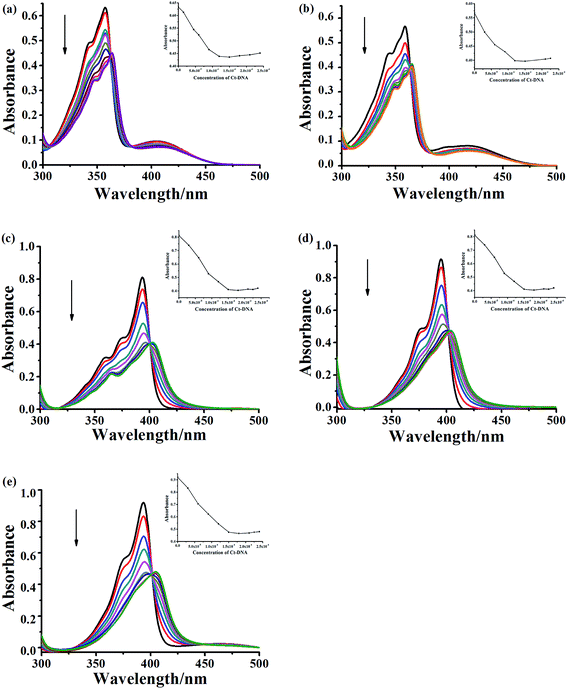 |
| Fig. 3 UV-vis spectral changes of compound 5a-1 (a), 5a-2 (b), 6a-1 (c), 6d-1 (d) and 6b-3 (e) at the concentration of 3.0 × 10−5 M upon addition of CT DNA (arrow: 0–210 μM) in phosphate buffer (1 mM, pH 7.4) containing 20 mM NaCl at 25 °C. | |
To further evaluate and compare the DNA binding performance of these compounds, the binding constants were determined from the following equation:
[DNA]/(εa − εf) = [DNA]/(εb − εf) + 1/Kb(εb − εf) |
where [DNA] is the concentration of DNA in base pairs,
εa,
εf and
εb correspond to average molar extinction coefficient of the solution (
Aobsd/[compound]), molar extinction coefficient of free compound, and molar extinction coefficient of completely bound compound, respectively.
Kb represents the intrinsic binding constant. A linear fit of the plot of [DNA]/(
εa −
εf)
versus [DNA] provides a slope of 1/(
εb −
εf) and an intercept of 1/
Kb(
εb −
εf).
Kb could be calculated. The binding constants of the compounds with CT DNA were obtained and found to be 3.33 × 10
4 M
−1, 1.67 × 10
4 M
−1, 3.50 × 10
5 M
−1, 1.42 × 10
5 M
−1, 2.34 × 10
5 M
−1, respectively. It was found that the DNA binding constants of the monocationic quaternary ammonium salts of 6
H-indolo[2,3-
b]quinoxaline derivatives are in the order of 10
4, while that of the dicationic quaternary ammonium salts are in the order of 10
5. This suggests that
N-methylation at 11-position of the indoloquinoxaline ring enhanced the binding ability of the compounds with DNA. This finding may also be attributed to the double-advantage of
N-methylated aza-aromatic moieties,
i.e. increasing the π stacking ability of the ligand due to the reduction of the electron density of the aromatic part and the introduction of a steadily positive charge for the electrostatic interaction.
46 Taking this latter notion into consideration, the dicationic quaternary ammonium salts of 6
H-indolo[2,3-
b]quinoxaline derivatives exhibited a remarkably high binding constant to DNA, which may explain their high antitumor activity compared to monocationic quaternary ammonium salts.
In addition, other variation in the structure, such as the linker length, terminal amino-group and introduction of fluorine atom in the indoloquinoxaline moiety, are shown to have an immediate significant influence on the DNA binding ability. For monocationic quaternary ammonium salts, the binding constants decrease as the linker length increases (5a-1 > 5a-2). The compounds with pyrrolidine at the end of side chain show more stronger CT DNA binding affinity than that of compound decorated with piperidine (6a-1 > 6b-3). The introduction of fluorine atom into the aromatic core could also enhance DNA binding affinity (6a-1 > 6d-3).
2.4.2. Fluorescence property. To gain insights into the binding mode of compounds 5a-1 and 5a-2 with CT DNA, fluorescence titration experiments were performed. Fig. 4 shows the emission spectra of the two compounds in the absence and presence of varying amounts of CT DNA. The results show that the emission intensities of compounds 5a-1 and 5a-2 increased with increasing the CT-DNA concentration, and the wavelength showed a distinct blue shift, similar to the classical model of ethidium bromide (EB) intercalating in DNA.47 An emission increase upon binding to DNA is often considered to be a good indicator for intercalation of the drug between the DNA base pairs, and thus, these measurements support an intercalative binding mode of 5a-1 and 5a-2 with CT DNA.
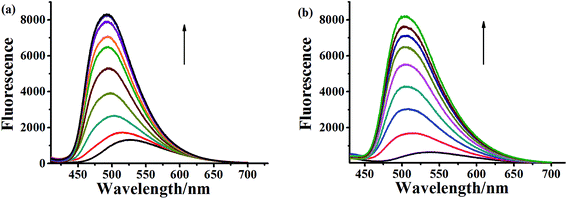 |
| Fig. 4 Fluorescence spectral changes of compound 5a-1 (a) and 5a-2 (b) at the concentration of 3.0 × 10−5 M upon addition of CT DNA (arrow: 0–210 μM) in phosphate buffer (1 mM, pH 7.4) containing 20 mM NaCl at 25 °C. | |
2.4.3. Circular dichroism (CD). The difference in absorption of polarised light provides the foundation of recording a CD spectrum. From the changes in the CD of DNA and the induced circular dichroism (ICD) of the compounds, the binding mode and the interaction mechanism could be explained. Compounds 5a-1, 5a-2, 6a-1, 6d-1 and 6b-3 were selected for the measurement of CD spectra at a certain ratio (cf. Fig. 5). Both data on the CD changes of DNA and ICD involving the tested compounds were obtained. In the absence of a drug, the CD spectrum of CT-DNA was of a typical B form, exhibiting a positive Cotton effect near 277 nm due to base staking and a negative Cotton effect near 247 nm due to polynucleotide helicity.48 Upon addition of these selected compounds, the CD spectrum of the resulting DNA underwent an increase in both the positive and negative bands as shown in Fig. 5. The result give evidence of a mode of intercalation with DNA, since it is generally accepted that the classical intercalation enhances the base stacking and stabilizes helicity, and thus increases intensities of the both bands, whereas simple groove binding and electrostatic interaction of small molecules show less or no perturbation on the base stacking and helicity bands.49
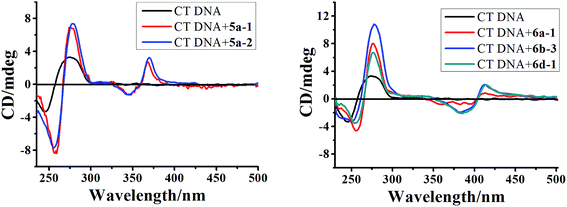 |
| Fig. 5 CD spectra of CT DNA (6.0 × 10−5 M) in the absence and presence of 5a-1, 5a-2, 6a-1, 6b-3 and 6d-1 (5.0 × 10−5 M) in phosphate buffer (1 mM, pH 7.4) containing 20 mM NaCl at 25 °C. | |
Further evidence for the binding modes of these compounds was obtained from the ICD spectra in the range of 325–450 nm corresponding to an indoloquinoxaline chromophore. The ICD signal, displayed by these achiral molecules as a result of interaction with the chiral DNA, usually depends on the position and orientation with respect to the polynucleotide. Generally, adducts displaying intercalation binding characteristics were found to exhibit a positive or negative ICD.50 Intriguingly, ICD spectra of the tested compounds and CT DNA exhibited a weaker and biphasic ICD signal. The biphasic shape of the CD spectrum, i.e. a negative band at lower wavelengths and a positive band at higher wavelengths, is characteristic of chromophores with a right-hand orientation, as expected for dyes bound to B-form DNA.51 The exciton CD observed in the drug absorption region is generally considered as evidence of groove binding or external stacking.44 However, the groove binding mode should be ruled out in this case due to the following consideration. Firstly, the ICD of groove binders is generally an order of magnitude stronger than those of intercalators and usually carries a positive sign if DNA is in B-form.48,52 Secondly, in the absorption titration we have demonstrated that these drugs mixed with CT DNA still contain a partially free chromophore which did not interfere with the DNA helix. This in turn may be due to the notion that an external binding mode may exist simultaneously. Thirdly, considering the positive nature of these compounds and negatively charged polyphosphate skeleton of DNA, the electrostatic interactions between the intercalator and DNA has to be considered. Taken in concert, these results, together with light absorption characteristics, may suggest multiple binding modes including intercalation as major and external binding as minor mode.
2.4.4. Thermal denaturation. Thermal denaturation studies of CT DNA are useful to determine the ability of the present compound to stabilize double stranded DNA. The intercalation of small molecules into the double helix is known to increase the DNA melting temperature (Tm). The Tm of DNA characterizes the transition from double-strand to single-strand nucleic acid.53 DNA melting studies were carried out with CT DNA in the absence and presence of compound. The melting profile for CT DNA in the absence of compound was 69.4 °C. As shown in Fig. 6 and Table 6, under the same experimental conditions, the presence of compounds 5a-1 and 5a-2 increased the Tm value about 4.1 and 8.4 °C, while the Tm value for compounds 6a-1, 6d-1 and 6b-3 increased 9.9, 10.8 and 11.7 °C, respectively. The dicationic quaternary ammonium salts derivatives possessed higher DNA melting temperatures than the corresponding mono quaternary ammonium salt derivatives. These results further indicate that compounds 6a-1, 6d-1 and 6b-3 with methylation at 11-N exhibited strong affinities with CT DNA, consistent with the results obtained from UV-vis analysis. Taken in concert, the above results further emphasize that the novel 9-fluoro-6H-indolo[2,3-b]quinoxaline derivatives as DNA intercalators exhibited significant DNA binding affinities.
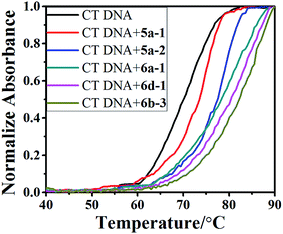 |
| Fig. 6 DNA melting curves for CT DNA (5.0 × 10−5 M) in the absence and presence of 5a-1, 5a-2, 6a-1, 6d-1 and 6b-3 with concentration of 5.0 × 10−6 M in phosphate buffer (1 mM, pH 7.4) containing 20 mM NaCl. | |
Table 6 Average Tm and ΔTm for CT-DNA in the absence and presence of 5a-1, 5a-2, 6a-1, 6b-3 and 6d-1
Compound |
Tm (°C) |
ΔTm (°C) |
CT DNA |
69.4 |
0 |
5a-1 |
73.5 |
4.1 |
5a-2 |
77.8 |
8.4 |
6a-1 |
79.3 |
9.9 |
6b-3 |
81.1 |
11.7 |
6d-1 |
80.2 |
10.8 |
3. Conclusions
A series of novel 9-fluoro-6H-indolo[2,3-b]quinoxaline derivatives functionalized with amino side chains and their monocationic/dicationic quaternary ammonium salts were designed and synthesized and their interactions with CT DNA were studied. The incorporation of fluorine, alkyl amino side chains and the introduction of positive charge at 11-N position of the aromatic scaffold were found to significantly influence the antitumor activity and further demonstrated to enhance the binding affinity of the compounds with DNA. The antitumor activity and the DNA binding affinity of most of the synthesized quaternary dicationic FIQX derivatives were found to be significantly higher compared to the corresponding monocationic FIQX derivatives and the neutral 6H-indolo[2,3-b]quinoxaline derivatives. The synthesized dicationic FIQX derivatives with pyrrolidine or piperidinyl as terminal amino-groups exhibited a higher inhibitory activity. The molecular structure–DNA binding relationships assessed in this study may be useful for the design of 6H-indolo[2,3-b]quinoxaline derivatives with desired DNA binding properties and promising antitumor abilities.
4. Experimental section
4.1. Materials
All chemicals and reagents were of analytical grade. RPMI-1640 medium, trypsin and fetal bovine serum were purchased from Gibco (Grand Island, NY, USA). CT DNA, MTT, benzylpenicillin and streptomycin were purchased from Sigma-Aldrich (St. Louis, MO, USA). Three different human carcinoma cell lines, MCF-7, HeLa and A549, were obtained from American Type Culture Collection.
4.2. Instrumentation and measurements
The melting points were determined on an XT-4 microscopic melting-point spectrometer and are shown uncorrected. Elemental analysis was performed on an Elementar Vario EL III elemental analyzer. Infrared (IR) spectra were measured on a Perkin-Elmer Model-683 spectrophotometer, with samples analyzed as KBr pellets. The 1H and 13C NMR spectra were recorded on a Bruker AVIII 600 NMR spectrometer. Mass spectra were measured using an Agilent 1200-6310 LC-MS apparatus. High-resolution mass spectra (HRMS) were recorded on a Bruker Apex Ultra 7.0T FTMS mass spectrometer. UV-vis absorption spectra were recorded on a Shimadzu UV-3600 UV-vis spectrophotometer and fluorescent spectra were measured on a Hitachi F-7000 luminescence spectrophotometer. The optical density (OD) was measured on a microplate spectrophotometer (Bio-Rad Model 680, USA). The yield, IR, 1H NMR, 13C NMR and high-resolution electrospray ionization (HRESI) MS spectral data of the obtained compounds are provided in the ESI section.†
4.3. Synthesis of compounds
4.3.1. 9-Fluoro-6H-indolo[2,3-b]quinoxaline. A mixture of 5-fluoroisatin (10 g, 0.04 mol) and o-phenylenediamine (6.6 g, 0.05 mol) in acetic acid (100 mL) was refluxed with stirring for 5 h. The reaction mixture was then cooled to room temperature and neutralized with a saturated solution of sodium hydroxide to pH 7. The solid product was filtered off, washed with acetic acid (3–5 mL) and recrystallized from ethyl alcohol.
4.3.2. General procedure for preparation of series of compounds (3). To a stirred suspension of 9-fluoro-6H-indolo[2,3-b]quinoxaline (2.39 g, 0.01 mol) in 30 mL of THF was added sodium hydroxide (2.81 g, 0.05 mol). The obtained solution was stirred for 30 min at 45 °C and dibromoalkane (0.05 mol) was added. After refluxing for 6 h, the reaction mixture was evaporated under reduced pressure and the residue was washed with water, filtered and dried. The product was purified by silica gel column chromatography using a mixture of petroleum ether and ethyl acetate as eluent.
4.3.3. General procedure for preparation of series of compounds (4). Cyclic secondary amine (10 mol) was added to a solution of compound 3 (1 mol) in 10 mL of THF. The reaction mixture was refluxed under nitrogen atmosphere until reaction completion as judged by TLC. The reaction mixture was then evaporated under reduced pressure and the residue was dissolved in 20 mL of water and extracted with ethyl acetate (3 × 15 mL). The extract was then neutralized with a saturated solution of sodium carbonate to pH 8–9 and the obtained precipitate was collected. The product was further purified by silica gel column chromatography using a mixture of petroleum ether and ethyl acetate as eluent.
4.3.4. General procedure for preparation of series of compounds (5). Iodomethane (1 mL) was added to a solution of compound 4 (0.5 mmol) in 5 mL of acetone under nitrogen atmosphere. The reaction mixture was stirred at room temperature until reaction completion as judged by TLC control. The precipitate was collected, washed with diethyl ether (3 × 5 mL) and dried.
4.3.5. General procedure for preparation of series of compounds (6). A suspension of compound 4 (0.5 mmol), iodomethane (2 mL) and tetramethylenesulfone (3 mL) was heated in a sealed flask overnight at 55 °C. The obtained clear, deep-red solution was cooled to room temperature. Next, 5 mL of water and 0.46 g of KPF6 (2.5 mmol) was added and the mixture was stirred at 45 °C for 2 h. The resulted brick precipitate was filtered off, dried, and recrystallized from methanol.
4.3.6. Data collection and structural refinement of the precursor of compound 6b-1. X-ray data collection of the precursor of compound 6b-1 was performed on a Bruker SMART APEX II CCD diffractometer equipped with a graphite monochromator (Mo Kα radiation) (λ = 0.71073 Å) at 296(2) K. Multi-scan absorption corrections were carried out using the SADABS program. The structure was solved by a direct method using the SHELXS-97 program. Refinements on F2 were performed using SHELXL-97 by a full-matrix least-squares method with anisotropic thermal parameters for all non-hydrogen atoms. Crystallographic data for the structural analysis of the precursor of compound 6b-1 have been deposited at the Cambridge Crystallographic Data Centre, CCDC-1541589.†
4.4. Procedure for in vitro cytotoxictity assessment
In vitro cytotoxictity of the obtained compounds was evaluated by a MTT colorimetric assay.54 Three different human carcinoma cell lines, MCF-7, HeLa and A549, were cultured in Dulbecco's modified Eagel's medium (DMEM, Gibco, USA) solution supplemented with 10% fetal bovine serum, 100 U mL−1 of penicillin, and 100 μg mL−1 of streptomycin. The cancer cells were seeded at a density of 1.5 × 104 cells per well into a 96-well microplate pre-incubated in a 5% CO2/95% air-humidified atmosphere at 37 °C for 12 h to acquire adherent cells.
Prior to dilution, a stock solution of the compounds (5 mM) was prepared in DMSO. The 50% inhibitory concentration (IC50) was determinated over a range of concentrations (0.1–100 μM). In all experiments, the concentration of DMSO was less than 0.1% (v/v) to avoid DMSO toxicity.
Wells containing culture medium without cells were used as blanks. Wells containing culture medium, FIQX and cisplatin were used as positive control. After incubation for 48 h with the test compounds, 20 μL of MTT solution (5 mg mL−1) was added to each well and the mixtures were incubated for an additional 4 h. Then, 100 μL of DMSO was added to solubilize the MTT formazan and the OD of each well was measured on a microplate spectrophotometer at a wavelength of 570 nm. The IC50 value was determined from plot of the %-viability against a defined dose of added compounds. Three independent experiments were performed in quintuplicate.
4.5. Procedure for DNA binding studies
4.5.1. UV-vis absorption spectra. To prepare the stock solutions of calf thymus DNA (CT-DNA), DNA powder (Sigma-Aldrich) was dissolved in Tris–HCl buffer (pH 7.4; concentration 10 mM) and the solutions were incubated at 4 °C for 12 h. The DNA solution showed a ratio of UV absorbance at 260 and 280 nm, A260/A280 of approximately 1.9, indicating that the DNA was sufficiently free of protein. The concentration of CT DNA was determined by measuring its absorption intensity at 260 nm with a known molar absorption coefficient value of 6600 M−1 cm−1. The selected compounds were dissolved in DMSO and diluted with Tris–HCl buffer to reach the required concentrations. Absorption spectra titration studies were performed by keeping the compound concentrations constant while varying the DNA concentrations at room temperature. Absorption spectra were recorded from 200 to 600 nm, and changes on the intensity of the bands were monitored. The titration processes were repeated until no spectral changes for at least four titrations could be observed, indicating that binding saturation was achieved.
4.5.2. Fluorescent spectra. Fluorescence absorption spectral studies were performed by keeping the compound concentration (30 μM) constant while varying the DNA concentration from 0 μM to 210 μM at room temperature. The fluorescence wavelength and intensity area of the samples were measured by the excitation wavelength at 360 nm.
4.5.3. Circular dichroism spectra. CD spectra of CT DNA modified by 5a-1, 5a-2, 6a-1, 6d-1 and 6b-3 were recorded at 25 °C in 25 mmol L−1 sodium phosphate buffer (pH = 7.4) by using a MOS-450 spectrophotometer (Bio-Logic, France) equipped with a thermoelectrically controlled cell holder with a cell path length of 1 cm. CD spectra were recorded by keeping the DNA concentration (60 μM) constant while varying the compound concentrations until no spectral changes for at least three titrations in the region of 200–500 nm could be observed. The spectrum of the corresponding buffer and of the compounds alone were also collected and subtracted from that of the reaction mixture.
4.5.4. DNA thermal denaturation. Thermal denaturation experiments were carried out on a Shimadzu UV-3600 spectrophotometer equipped with a high performance temperature controller (±0.1 °C). The melting temperature (Tm) was determined as the mid-point of the hyperchromic transition. The DNA (50 μM) was denatured by increasing the temperature from 35 to 90 °C at a speed of 1 °C min−1 in the presence and absence of compound 5a-1, 5a-2, 6a-1, 6d-1 and 6b-3 (5 μM) while the corresponding absorbance was monitored at 260 nm. The data were presented as (A − A0)/(Af − A0) versus temperature, where A, A0, and Af are the observed, the initial, and the final absorbance at 260 nm, respectively.
Conflicts of interest
There are no conflicts to declare.
Acknowledgements
This work was supported by the Nature Science Fund of Hebei Province (B2015201213, B2015201069), the Key Basic Research Special Foundation of Science Technology Ministry of Hebei Province (15962602D), the Key Research Project Foundation of Department of Education of Hebei Province (Grant No. ZH2012041).
References
- R. L. Siegel, K. D. Miller and A. Jemal, Cancer Statistics, Ca-Cancer J. Clin., 2015, 65, 5–29 CrossRef PubMed.
- B. G. Jackson, Science, 2000, 287, 1969–1973 CrossRef.
- American Cancer Society, Inc., Surveillance Research. Cancer Faiths and Figures, 2005, http://www.geocites.com.
- J. Fischer and C. R. Ganellin, Analogue-based drug discovery, Wiley-VCH, Weinheim, 2006, pp. 1–24 Search PubMed.
- X. B. Zhou, M. Chen, Z. Y. Zheng, G. Y. Zhu, Z. H. Jiang and L. P. Bai, RSC Adv., 2017, 7, 26921–26929 RSC.
- B. Zhang, R. Y. Guo, Y. Z. Hu, X. W. Dong, N. M. Lin, X. Y. Dai, H. H. Wu, S. L. Ma and B. Yang, RSC Adv., 2017, 7, 31899–31906 RSC.
- Y. Chen, H. Z. Lin, J. Zhu, K. Gu, Q. Li, S. Y. He, X. Lu, R. X. Tan, Y. Q. Pei, L. Wu, Y. Y. Bian and H. P. Sun, RSC Adv., 2017, 7, 33851–31867 RSC.
- T. Zawadowski and M. Klimaszewska, Acta Pol. Pharm., 1995, 52, 249–252 CAS.
- S. T. Hazeldine, L. Polin, J. Kushner, K. White, T. H. Corbett and J. P. Horwitz, Bioorg. Med. Chem., 2006, 14, 2462–2467 CrossRef CAS PubMed.
- P. J. Aragon, A. D. Yapi, F. Pinguet, J. M. Chezal, J. C. Teulade and Y. Blache, Chem. Pharm. Bull., 2007, 55, 1349–1355 CrossRef CAS PubMed.
- L. M. Wilhelmsson, N. Kingi and J. Bergman, J. Med. Chem., 2008, 51, 7744–7750 CrossRef CAS PubMed.
- N. S. H. N. Moorthy, E. Manivannan, C. Karthikeyan and P. Trivedi, Mini-Rev. Med. Chem., 2013, 13, 1415–1420 CrossRef PubMed.
- N. S. H. N. Moorthy, C. Karthikeyan and P. Trivedi, J. Enzyme Inhib. Med. Chem., 2010, 25, 394–405 CrossRef CAS PubMed.
- M. Stiborová, J. Poljaková, E. Martínková, L. Borek-Dohalska, T. Eckschlager, R. Kizek and E. Frei, Interdiscip. Toxicol., 2011, 4, 98–105 Search PubMed.
- A. M. A. Osman, E. B. Pedersen and J. Bergman, Nucleosides, Nucleotides Nucleic Acids, 2013, 32, 98–108 CAS.
- N. Patel, J. Bergman and A. H. Gräslund, Eur. J. Biochem., 1991, 197, 597–604 CrossRef CAS PubMed.
- I. Zegar, A. Gräslund, J. Bergman, M. Eriksson and B. Nordén, Chem.-Biol. Interact., 1989, 72, 277–293 CrossRef CAS PubMed.
- J. Bergman, C. Damberg and H. Valberg, Recl. Trav. Chim. Pays-Bas, 1996, 115, 31–36 CrossRef CAS.
- U. Sehlstedt, P. Aich, J. Bergman, H. Vallberg, B. Nordén and A. Gräslund, J. Mol. Biol., 1998, 278, 31–56 CrossRef CAS PubMed.
- J. Harmenberg, A. Åkesson-Johansson, A. Gräslund, T. Malmfors, J. Bergman, B. Wahren, S. Åkerfeldt, L. Lundblad and S. Cox, Antiviral Res., 1991, 15, 193–204 CrossRef CAS PubMed.
- K. Hirata, J. Araya, S. Nakaike, K. Kitamura and T. Ishida, Chem.
Pharm. Bull., 2001, 49, 44–48 CrossRef CAS PubMed.
- T. Ishida, Y. Mihara, Y. Hama, A. Hanatani, M. Tarui, M. Doi, S. Nakaike and K. Kitamura, Chem. Pharm. Bull., 1998, 46, 739–743 CrossRef CAS PubMed.
- S. P. Nikumbh, A. Raghunadh, V. N. Murthy, R. Jinkala, S. C. Joseph, Y. L. N. Murthy, B. Prasad and M. Pal, RSC Adv., 2015, 5, 74570–74574 RSC.
- S. P. Nikumbh, A. Raghunadh, T. S. Rao, V. N. Murthy, S. C. Joseph, Y. L. N. Murthy and M. Pal, RSC Adv., 2016, 6, 23489–23497 RSC.
- K. W. Hagmann, J. Med. Chem., 2008, 51, 4359–4369 CrossRef PubMed.
- S. S. Karki, R. Hazare, S. Kumar, V. S. Bhadauria, J. Balzarini and E. De Clercq, Acta Pharm., 2009, 59, 431–440 CrossRef CAS PubMed.
- M. F. Braña, M. Cacho, A. Gradillas, B. de Pascual-Teresa and A. Ramos, Curr. Pharm. Des., 2001, 7, 1745–1780 CrossRef.
- D. Monchaud and M. P. Teulade-Fichou, Org. Biomol. Chem., 2008, 6, 627–636 CAS.
- G. W. Gribble, Synthesis and antitumor activity of ellipticine alkaloids and related compounds, in The Alkaloids, ed. A. Brossi, Academic Press, New York, 1990, vol. 39, pp. 239–343 Search PubMed.
- V. K. Kansal and P. Potier, Tetrahedron, 1986, 32, 2389–2408 CrossRef.
- M. O. Shibinskaya, S. A. Lyakhov, A. V. Mazepa, S. A. Andronati, A. V. Turov, N. M. Zholobak and N. Y. Spivak, Eur. J. Med. Chem., 2010, 45, 1237–1243 CrossRef CAS PubMed.
- M. O. Shibinskaya, A. S. Karpenko, S. A. Lyakhov, S. A. Andronati, N. M. Zholobak, N. Y. Spivak, N. A. Samochina, L. M. Shafran, M. J. Zubritsky and V. F. Galat, Eur. J. Med. Chem., 2011, 46, 794–798 CrossRef CAS PubMed.
- P. B. Arimondo, B. Baldeyrou, W. Laine, C. Bal, F. A. Alphonse, S. Routier, G. Coudert, J. Y. Mérour, P. Colson, C. Houssier and C. Bailly, Chem.-Biol. Interact., 2001, 138, 59–75 CrossRef CAS PubMed.
- M. O. Shibinskaya, N. A. Kutuzova, A. V. Mazepa, S. A. Lyakhov, S. A. Andronati, M. J. Zubritsky, V. F. Galat, J. Lipkowski and V. C. Kravtsov, J. Heterocycl. Chem., 2012, 49, 678–682 CrossRef CAS.
- K. M. Driller, S. Libnow, M. Hein, M. Harms, K. Wende, M. Lalk, D. Michalik, H. Reinke and P. Langer, Org. Biomol. Chem., 2008, 6, 4218–4223 CAS.
- M. C. Wamberg, A. A. Hassan, A. D. Bond and E. B. Pedersen, Tetrahedron, 2006, 62, 11187–11199 CrossRef CAS.
- S. Avula, J. R. Komsani, S. Koppireddi, R. Yadla, A. K. R. Kanugula and S. Kotamraju, Med. Chem. Res., 2013, 22, 3712–3718 CrossRef CAS.
- A. H. Abadi, Arch. Pharm., 1998, 331, 352–358 CrossRef CAS PubMed.
- J. H. Tan, Q. X. Zhang, Z. S. Huang, Y. Chen, X. D. Wang, L. Q. Gu and J. Y. Wu, Eur. J. Med. Chem., 2006, 41, 1041–1047 CrossRef CAS PubMed.
- X. Q. Chen and J. M. Gu, App. Chem. Ind., 2007, 36, 901–902 CAS.
- V. Q. Yen, N. P. Buu-Hoi and N. D. Xuong, J. Org. Chem., 1958, 23, 1858–1861 CrossRef CAS.
- P. Helissey, S. Desbene-Finck and S. Giorgi-Renault, Eur. J. Org. Chem., 2005, 410–415 CrossRef CAS.
- Z. F. Chen, S. P. Zhang, J. Zhang and Z. Z. Zhu, New J. Chem., 2017, 41, 6760–6768 RSC.
- S. J. T. Rezaei, V. Amani, M. R. Nabid, N. Safari and H. Niknejad, Polym. Chem., 2015, 6, 2986 RSC.
- R. Raveendran, J. P. Braude, E. Wexselblatt, V. Novohradsky, O. Stuchlikova, V. Brabec, V. Gandin and D. Gibson, Chem. Sci., 2016, 7, 2381–2391 RSC.
- Y. J. Lu, T. M. Ou, J. H. Tan, J. Q. Hou, W. Y. Shao, D. Peng, N. Sun, X. D. Wang, W. B. Wu, X. Z. Bu, Z. S. Huang, D. L. Ma, K. Y. Wong and L. Q. Gu, J. Med. Chem., 2008, 51, 6381–6392 CrossRef CAS PubMed.
- R. Palchaudhuri and P. J. Hergenrother, Curr. Opin. Biotechnol., 2007, 18, 497–503 CrossRef CAS PubMed.
- J. H. Tan, Y. J. Lu, Z. S. Huang, L. Q. Gu and J. Y. Wu, Eur. J. Med. Chem., 2007, 42, 1169–1175 CrossRef CAS PubMed.
- M. V. Keck and S. J. Lippard, J. Am. Chem. Soc., 1992, 114, 3386–3390 CrossRef CAS.
- P. Pradhan, B. Jernström, A. Seidel, B. Nordén and A. Gräslund, Biochemistry, 1998, 37, 4664–4673 CrossRef CAS PubMed.
- K. M. Sovenyhazy, J. A. Bordelon and J. T. Petty, Nucleic Acids Res., 2003, 31, 2561–2569 CrossRef CAS PubMed.
- B. Norden and T. Kurucsev, J. Mol. Recognit., 1994, 7, 141–156 CrossRef CAS PubMed.
- Y. Cao and X. W. He, Spectrochim. Acta, Part A, 1998, 54, 883–892 CrossRef.
- T. Mosmann, J. Immunol. Methods, 1983, 65, 55–63 CrossRef CAS PubMed.
Footnote |
† Electronic supplementary information (ESI) available. CCDC 1541589. For ESI and crystallographic data in CIF or other electronic format see DOI: 10.1039/c7ra08138c |
|
This journal is © The Royal Society of Chemistry 2017 |
Click here to see how this site uses Cookies. View our privacy policy here.