DOI:
10.1039/C7RA07814E
(Paper)
RSC Adv., 2017,
7, 41936-41944
Probing the thermal-enhanced catalytic activity of CO oxidation over Pd/OMS-2 catalysts†
Received
16th July 2017
, Accepted 15th August 2017
First published on 30th August 2017
Abstract
The present research has probed the effect of different thermal-treatment temperatures on the catalytic activity of CO oxidation and the physio-chemical properties of an OMS-2 supported palladium catalyst. The catalytic activity of Pd/OMS-2 increased with an increase in the thermal-treatment temperature from 300 to 500 °C. The optimal Pd/OMS-2 catalyst exhibited over 99% CO conversion at 35 °C. An elevated thermal-treatment temperature up to 500 °C led to a decrease in OMS-2 crystallinity and surface chemisorbed oxygen (–OH), while the surface atomic ratios of oxygen/Mn and palladium increased with the calcination temperature. A high thermal-treatment temperature above 500 °C led to the phase transformation of OMS-2 into Mn2O3, and an abrupt alteration in the Pd–support interaction and deactivation of the Pd/OMS-2 catalyst.
1. Introduction
Catalytic oxidation of carbon monoxide (CO) has been widely explored in the fields of low-caloric-value gas catalytic combustion, automobile exhaust purification, indoor air cleaning and academic research.1,2 Supported Au,3 Ag4 and platinum group metals (PGMs)5 have been explored and regarded as active for a low-temperature catalytic oxidation of CO approach at below room temperature. In terms of commercial application, palladium-based catalysts have been applied to CO and hydrocarbons (HC) from automobile and industrial exhausts due to their reasonable price, superior low-temperature activity and stability.6 Multi-valence transitional and rare-earth metal oxides (oxides of Co, Mn, Fe, Ni, Cu, Ce, etc.) have been known to have oxygen vacancies, excellent oxygen storing capacity (OSC) and redox properties.7–9 Since CO adsorption, oxygen storing and transfer capacity are critical for the low-temperature catalytic oxidation of CO over metal oxide supported palladium catalysts, there remains a substantial amount of work to optimize oxygen vacancies, OSC and the surface dispersion of palladium over multi-valence metal oxide supported palladium catalysts. Molecular sieves with ordered porosity are preferred to enhance the stability and surface dispersion of palladium, while multi-valence manganese oxides are known for their excellent OSC and redox properties. Octahedral molecular sieves (OMS-2) simultaneously contain multi-valence Mn2+, Mn3+, Mn4+ manganese oxide and the ordered 2 × 2 microchannels of molecular sieves.10 However, in order to enhance the dispersion of palladium and the redox properties of Pd/OMS-2, there remain great efforts to optimize the morphology and surface chemical properties of the OMS-2 structure. In our previous work,11 we found that different preparation methods have an obvious effect on the crystallinity, morphology, surface chemical properties and catalytic oxidation of the Pd/OMS-2 catalysts. OMS-2 nanorods prepared by a hydrothermal method exhibit optimal catalytic properties. In recent years, a facile calcination-enhanced redispersion of palladium over metal oxide supported Pd catalysts has been reported to be active for low-temperature CO oxidation.12,13 Despite a substantial amount of work on the thermal stability of OMS-2,14,15 to the best of our knowledge, the thermal-enhanced catalytic activity of CO oxidation over a Pd/OMS-2 catalyst has rarely been reported.
Our interest has been attracted in exploring the effect of elevating thermal-treatment temperature on the phase composition, oxygen vacancies, surface chemical properties and catalytic activity of CO oxidation over Pd/OMS-2 catalysts.
2. Experimental
2.1 Catalyst preparation
2.1.1 Preparation of OMS-2. All OMS-2 supports are prepared with a conventional hydrothermal method, as described previously.11 In a typical procedure, 19.3 mmol of Mn(CH3COO)2·4H2O, 27.8 mmol of KMnO4 and 1 mL of concentrated nitric acid were added to 64 mL of distilled water with stirring. The mixture was then transferred into a Teflon-lined stainless steel autoclave and hydrothermally treated at 100 °C for 24 h. The resulting precipitate was filtered, washed with deionized water, dried at 100 °C overnight and calcined at 350 °C for 3 h with a heating rate of 3 °C min−1.
2.1.2 Preparation of Pd/OMS-2. Pd/OMS-2 catalysts are prepared with an impregnation method using palladium nitrate as precursor with a palladium content of 1% (wt%). The above prepared OMS-2 has been impregnated with an aqueous solution of Pd(NO3)2 and dried at 70 °C. The dried powders were calcined at 300, 400, 500 and 600 °C and denoted by Pd/OMS-2-300, Pd/OMS-2-400, Pd/OMS-2-500, Pd/OMS-2-600, respectively. For example, Pd/OMS-2-500 means calcining Pd(NO3)2 impregnated OMS-2 at 500 °C for 4 h with a heating rate of 3 °C min−1.
2.2 Catalyst characterization
X-ray powder diffraction (XRD) analysis was performed on a Bruker D8 Advance diffractometer system, with an operating voltage of 40 kV and a current of 40 mA, using Cu-Ka radiation (λ = 1.5406 nm) and a graphite monochromator. The samples were investigated in the 2θ range from 10 to 70° at a scanning speed of 0.03° s−1. X-ray photoelectron spectroscopy (XPS) tests were carried out on an ULVAC PHI 5000 Probe-II spectrometer using Al Kα radiation under UHV. The C 1s peak (284.8 eV) was used for the calibration of the binding energy values. The surface atomic concentrations of Mn, Pd, and O, as well as the peak areas were determined with the XPS Peak Fit (Version 4.0, AISN Software Inc.) software. Transmission electron microscopy (TEM) tests were measured on a FEI Tecnai G220 transmission electron microscope, using an accelerating voltage of 200 kV. N2 adsorption/desorption tests were carried out on a Micromeritics TriStar II 3020 instrument at 77 K using liquid N2 as adsorbent. Specific surface areas (SSAs) were determined by the Brunauer–Emmett–Teller (BET) equation. The pore volume was determined from the adsorption isotherms. Pore size distributions were determined by the non-local density functional theory (NL-DFT). The low-temperature H2-temperature programmed reduction (H2-TPR) tests were performed on a homemade U-shaped quartz-tube fixed-bed reactor (i.d. 3 nm). A 10 mg sample and 30 mg of quartz sand were mixed and filled for each measurement. The samples were pre-treated in flowing N2 (30 mL min−1) at 300 °C for 60 min and then cooled to 0 °C. After that, all H2-TPR runs were performed from 0 to 90 °C under 5% H2 in Ar with a heating rate of 92 °C min−1. Prior to the reaction, the catalysts were pre-treated in flowing N2 (30 mL min−1) at 300 °C for 60 min and then cooled to 0 °C. A thermal conductivity detector (TCD) was used to monitor the online consumption of H2. Raman tests were performed on a Renishaw inVia Laser micro confocal Raman spectrometer system equipped with a 50× Leica objective lense to select the target area (spot) of the sample. Laser Raman tests were carried out on the same spot irradiated by a visible Ar ion laser (514.5 nm). Prior to the test, steady-flow argon was introduced into the catalyst held at 25 °C for 30 min.
2.3 Catalytic activity test
Catalytic activity of CO oxidation was studied in a down-flow quartz-tube reactor (i.d. = 6 mm) with a K-type thermocouple enclosed to monitor the reaction temperature. 200 mg of catalyst was used for each reaction and the total gas flow rate was 50 mL min−1 under atmospheric pressure. The reaction gas contents consist of 1% CO (vol%) and 10.5% O2 (vol%) balanced with N2. The gas components were detected by a gas chromatograph with a TDX-01 column, a methanator and a flame ionization detector (FID) under steady-state conditions. The conversion of CO oxidation was calculated using the following equation:
where [CO]in is the CO concentration in the feed gas and [CO]out is the CO concentration in the effluent gas.
3. Results and discussion
3.1 Catalytic activity
It can be seen from Fig. 1 that the conversion of CO catalytic oxidation followed the orders of: Pd/OMS-2-500 > Pd/OMS-2-400 > Pd/OMS-2-300 > Pd/OMS-2-600. Over 99% CO conversion could be obtained by Pd/OMS-2-300, Pd/OMS-2-400 and Pd/OMS-2-500 at 35 °C, approaching room temperature. It should be noted that Pd/OMS-2-500 exhibits over 96% CO conversion below 20 °C. Over 96% CO conversion could also be obtained by Pd/OMS-2-400 and Pd/OMS-2-500. Pd/OMS-2-600 attained 99% CO conversion at no more than 50 °C. The overall catalytic conversion of CO oxidation increases with the calcination temperature of the palladium impregnated OMS-2. While a high calcination temperature above 500 °C may lead to an abrupt decrease in catalytic activity. Since the concentration of O2 is over 9% (v/v), much higher than the CO concentration, the reaction can be simplified to zero order with respect to oxygen and +1 with respect to CO for low CO concentration. The optimal Pd/OMS-2-500 exhibits an apparent activation energy of 12.8 kJ mol−1 (Fig. S1†). Therefore, it has attracted our great interest to further explore the potential reasons for this thermally-induced elevated activity and abrupt deactivation.
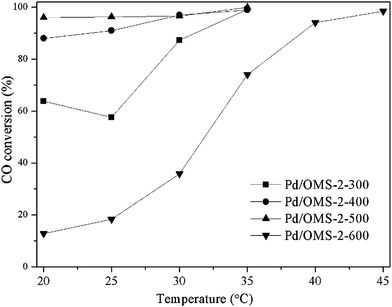 |
| Fig. 1 Conversion of CO catalytic oxidation over various catalysts (1.0 vol% CO, 10.5 vol% O2, N2 balanced and GHSV of 15 000 mL g−1 h−1). | |
3.2 XRD analysis
X-ray diffraction (XRD) spectra of various catalysts are shown in Fig. 2. All samples show obvious diffraction peaks of cryptomelane K2−xMn8O16 structure (PDF# 44-1386), indicating the existence of an octahedral molecular sieve (OMS-2) structure. The intensity of the diffraction peaks decreased when the calcination temperature increased from 300 to 500 °C. Therefore, it should be concluded that a relatively high calcination temperature is preferred to decrease the crystallinity. Obvious diffraction peaks of bixbyite Mn2O3 (PDF# 41-1442) could also be seen from the pattern of Pd/OMS-2-600, indicating that a relatively high calcination temperature beyond 500 °C could result in the formation of highly-crystalline bixbyite Mn2O3 and K2−xMn8O16. A potential phase transformation and sintering of the OMS-2 structure may have happened when the calcination temperature shifted from 500 to 600 °C. No obvious characterization peaks of palladium species could be observed from any of the samples, indicating that palladium species existed in the form of highly dispersed non-crystalline species or semicrystals beyond the detection limit of XRD analysis. Overall, it could be concluded that no sintering of palladium crystals could be observed from any of the samples within the detection limits of the XRD technique. Crystallinity decreased with an elevation of the calcination temperature from 300 to 500 °C, while the catalytic activity increased with the thermal-treatment temperature.
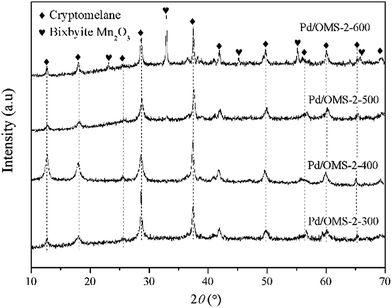 |
| Fig. 2 X-ray diffraction (XRD) patterns of the various catalysts. | |
3.3 N2 adsorption–desorption tests
N2 adsorption–desorption isotherms of various samples are shown in Fig. 3. Hysteresis loops of all samples starting at P/P0 ≥ 0.75 and terminating at P/P0 approach 1.0, indicating the presence of interparticle spaces and an abundance of ordered micropores over OMS-2's primitive topological structure (0.46 nm of 2 × 2 microchannels). The NL-DFT pore size distributions (PSD) of various samples are listed in Fig. 4. No obvious gradual uptakes or inflection points approaching P/P0 = 0 could be observed, indicating the low volumes of micropores in all the samples, despite the ordered 1-D microchannels of the OMS-2 structure. It should be noted that a majority of the OMS-2 reported previously exhibit the morphology of nanoparticles or nanorods, which may facilitate the formation of interparticle space. While our present PSD results (Fig. S2†) indicate a character which is more like interparticle space than the ordered microchannels of an OMS-2 structure. However, further morphological studies of TEM and STEM are required to further discuss the speculation mentioned above. It can be seen from Table 1 that the specific surface areas (SSA) of different samples follow the order of: Pd/OMS-2-400 > Pd/OMS-2-300 > Pd/OMS-2-500 > Pd/OMS-2-600. The present research indicates that SSA has no significant effect on the catalytic activity of the Pd/OMS-2 catalysts.
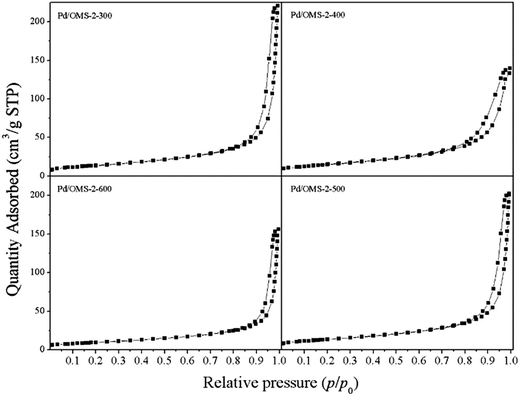 |
| Fig. 3 N2 adsorption–desorption isotherms of various catalysts. | |
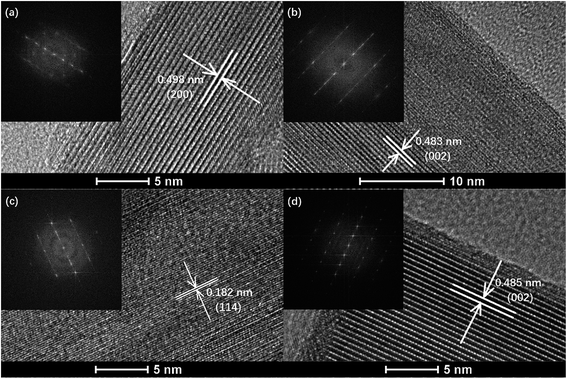 |
| Fig. 4 HR-TEM images of (a) Pd/OMS-2-300, (b) Pd/OMS-2-400, (c) Pd/OMS-2-500, (d) Pd/OMS-2-600. | |
Table 1 Specific surface area (SSA), pore volume and average pore size of various samples
Samples |
SSAa (m2 g−1) |
Pore volumeb (m3 g−1) |
Average pore sizec (nm) |
Specific surface area (SSA) was calculated from Brunauer–Emmett–Teller (BET) equation. Pore volume was calculated from single point adsorption total pore volume of pores less than 189.4 nm width at P/P0 = 0.990000000. Average pore size was determined from adsorption average pore diameter (4V/A by BET). |
Pd/OMS-2-300 |
49.4 |
0.311 |
25.2 |
Pd/OMS-2-400 |
53.5 |
0.195 |
14.5 |
Pd/OMS-2-500 |
47.0 |
0.297 |
24.8 |
Pd/OMS-2-600 |
35.0 |
0.211 |
24.1 |
3.4 High resolution transmission electron microscope (HR-TEM) analysis
High resolution transmission electron microscope (HR-TEM) images of various samples are shown in Fig. 4. All samples show a dispersed regular well-crystalline nanorod structure with a single or multiple crystal plane exposed. The diameter of the samples ranges from 35 to 55 nm and the length of the samples ranges from 200 to 400 nm (ESI Fig. S3 to S6†). No obvious aggregated particles of palladium species could be found, indicating high dispersion of the palladium species despite a high calcination temperature above 500 °C. A minority of semi crystals and irregular accumulated nanoparticles could be observed from all the samples. The irregular dispersed nanorods and accumulated nanoparticles may contribute to the interparticle space, as described in N2 adsorption–desorption analysis (Fig. 3 and Table 1). It should be noted that Pd/OMS-2-300 and Pd/OMS-2-400 show a relatively regular well-crystalline structure with the regular shape of a long longitude nanorod (ESI Fig. S3 to S6†).
3.5 X-ray photoelectron spectroscopy (XPS) analysis
X-ray photoelectron spectroscopy (XPS) analysis was employed to analyze the chemical state and atomic concentration of surface palladium, manganese, and oxygen species. ULVAC-PHI MultiPak™ Software Manual (Version 9, ULVAC-PHI Inc.) has been utilized to analyze the surface atomic concentration. It has also been reported that surface molar ratios of oxygen vacancies (Mn3+/Mn4+), Mnn+/Mntotal and Oadsorbed/Olattice have an important influence on oxygen storage and transfer capacity over multi-valence manganese oxides due to the dynamic balance of
and –Mn4+–O2−–Mn4+– → –Mn3+–O–Mn3+– + 1/2O2 in the presence of gaseous oxygen.16 Therefore, the present study has analyzed the surface molar ratios of Mn2+/Mntotal, Mn3+/Mntotal, Mn4+/Mntotal and Oadsorbed/Olattice over the various catalysts using XPS PeakFit (Version 4.0, AISN Software Inc.) software.
The surface atomic ratios of Mn2+/Mntotal, Mn3+/Mntotal and Mn4+/Mntotal were calculated as follows:
where
S1,
S2 and
S3 represent the corresponding peak areas of Mn
2+, Mn
3+ and Mn
4+, respectively.
The surface atomic ratios of Oadsorbed/Olattice were calculated using the following equation:
where
Sadsorbed and
Slattice are the peak areas corresponding to O
2− and OH
−/CO
32−, respectively.
It can be seen from Fig. 5 that Pd 3d5/2 and Pd 3d3/2 peaks located at 337.32 ± 0.04 eV and 342.65 ± 0.04 eV, respectively, indicate the existence of the Pd(II) state.17,18 No characteristics of Pd(IV) species could be observed from the peaks of the Pd 3d spectrum.19 It should also be noted that the present XPS technique has insufficient evidence to confirm the existence of strong metal–support interacted Pd(II) (PdSMSI). XPS spectra of Mn 2p in Fig. 6 showed 2p peaks of Mn located at 640.90 ± 0.04 eV, 642.10 ± 0.04 eV and 643.50 ± 0.04 eV indicating that Mn species coexisted in the multi-valence states of Mn2+, Mn3+ and Mn4+.20,21 The O 1s XPS spectra of the catalysts can be resolved into two peaks, as shown in Fig. 7. The peaks at 529.76 ± 0.03 eV (denoted by Olattice) could be attributed to the characteristic peak of metal–oxygen (the lattice oxygen, O2−), while the peaks at 531.5 eV (denoted by Oadsorbed) are generally regarded as the surface chemisorbed oxygen (OH− or CO32−).22,23
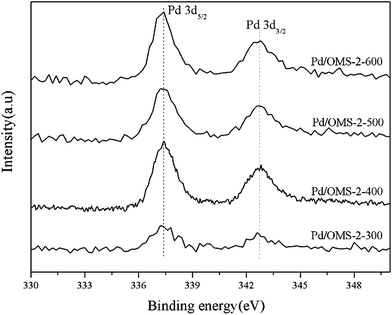 |
| Fig. 5 XPS spectra of Pd 3d over various samples. | |
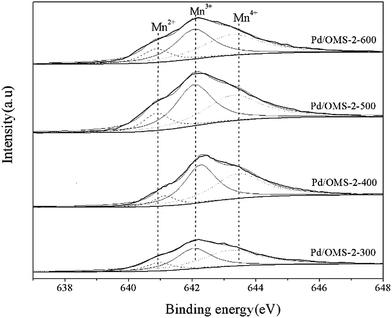 |
| Fig. 6 XPS spectra of Mn 2p over various samples. | |
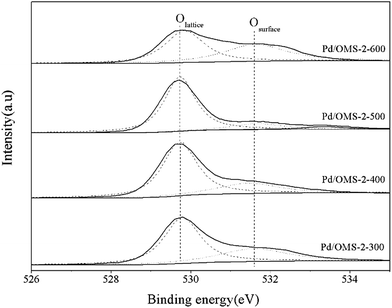 |
| Fig. 7 XPS spectra of O 1s over various samples. | |
Surface Mn2+
:
Mn3+
:
Mn4+ molar ratios of all samples are listed in Table 2. No obviously different molar ratios of Mn2+
:
Mn3+
:
Mn4+ could be observed from Pd/OMS-2-300, Pd/OMS-2-400 or Pd/OMS-2-500, indicating the maintenance of the OMS-2 structure.20 Rare surface potassium (K+) could be seen from Pd/OMS-2-300 and Pd/OMS-2-400, indicating the occupation of K+ in the 2 × 2 microchannels of the OMS-2 structure. However, Pd/OMS-2-500, Pd/OMS-2-600 showed a sudden emergence of surface potassium (K+). In addition, surface atomic ratios of palladium increased with the elevation of the thermal-treatment temperature above 400 °C. There was a potential spill-over effect of K+ from the 2 × 2 microchannels and/or decomposition of strong metal–support interacted palladium (PdSMSI) during the thermal treatment process at 500 to 600 °C. Above all, it could be concluded that an increase in thermal treatment temperature from 300 to 500 °C is preferred to improve surface palladium and potassium species, while the catalytic activity of CO oxidation also increased with the elevation of the calcination temperature from 300 to 500 °C.
Table 2 Surface atomic ratios of Mn2+/Mntotal, Mn3+/Mntotal, Mn4+/Mntotal, Oadsorbed/Olattice and surface atomic concentrations (%) of various samples
Samples |
Surface atomic concentration (%) |

|

|

|

|
Pd |
O |
Mn |
K |
Pd/OMS-2-300 |
0.34 |
77.40 |
22.26 |
0.00 |
43.38 |
30.89 |
34.09 |
35.02 |
Pd/OMS-2-400 |
0.50 |
66.00 |
33.50 |
0.00 |
40.07 |
29.68 |
35.23 |
35.08 |
Pd/OMS-2-500 |
0.59 |
66.15 |
30.38 |
2.88 |
35.53 |
30.73 |
34.99 |
34.28 |
Pd/OMS-2-600 |
0.91 |
69.41 |
26.86 |
2.82 |
47.95 |
31.04 |
34.44 |
34.52 |
It could be observed from Table 2 that Pd/OMS-2-400 and Pd/OMS-2-500 show approximately 2
:
1 surface O/Mn atomic ratios, while the surface O/Mn atomic ratios Pd/OMS-2-300 and Pd/OMS-2-600 are 3.48 and 2.58, respectively, which are obviously higher than the stoichiometric ratio of K2−xMn8O16. Is known that a majority of surface hydroxyl groups (−OH) may contribute to an increase in surface oxygen of the OMS-2 structure. While it is also known that a higher thermal treatment temperature may lead to a decrease in surface hydroxyl groups (−OH) of metal oxide.22 Therefore, it could be speculated that surface –OH contributed to the nonstoichiometric O/Mn atomic ratios of Pd/OMS-2-300. It should be noted that our XRD analysis (Fig. 2) indicates that Pd/OMS-2-600 exhibits two major crystalline phases of Mn2O3 and K2−xMn8O16, which indicate a total stoichiometric O/Mn ratio of less than 2.0. However, as a matter of fact, Pd/OMS-2-600 shows a surface O/Mn ratio of 2.58. Therefore, further analysis of structural and chemical data (Raman and H2-TPR. etc.) are expected. Although it is too early to conclude a defined correlation of catalytic activity between Oadsorbed/Olattice, O/Mn atomic ratios and catalytic activity of CO oxidation, to our great interest, Pd/OMS-2-400 and Pd/OMS-2-500 exhibit approximately 2
:
1 surface O/Mn atomic ratios of OMS-2 and an obviously higher catalytic activity than Pd/OMS-2-300 or Pd/OMS-2-600. Further exploration of the redox properties (H2-TPR) and laser Raman are expected.
3.6 H2 temperature programmed reduction analysis (H2-TPR)
Low-temperature programmed reduction (low-temperature H2-TPR) has been carried out to explore the redox properties of surface palladium species under elevated temperatures in H2/Ar. It can be seen from Fig. 8 that all samples show obvious peaks of H2 consumption at 65 to 75 °C, indicating the reduction of PdO and the Pd-assisted reduction of surface MnOx-interacted Pd(II).11,23 Peaks of reduction temperature (Fig. 8) shifted in the order Pd/OMS-2-600 (72.8 °C) > Pd/OMS-2-300 (67.5 °C) > Pd/OMS-2-400 (62.6 °C) > Pd/OMS-2-500 (58.9 °C). No significant differences in peak areas could be observed from any of the samples, indicating the same amount of H2 consumed by Pd(II) and Pd(II) interacted surface MnOx species (for example, Pd–O–Mn structure) (Table 3). Pd/OMS-2-300 (67.5 °C), Pd/OMS-2-400 and Pd/OMS-2-500 show relatively flat peaks with a wide full width at half maximum (FWHMs), while Pd/OMS-2-600 shows a sharp increase in peak height and a sharp decrease in full wave at half maximum (FWHM). Above all, compared with other samples, profiles of Pd/OMS-2-600 imply an abrupt alteration in the Pd–support interaction. It is reported that a relatively high calcination temperature may lead to a decrease in the strong palladium–support interaction or the transformation of PdSMSI into dispersed surface PdO and Pd0,12 which could enhance the low-temperature catalytic activity of CO oxidation.24,25 Reduction of the co-existing surface PdSMSI and PdO could be regarded as a potential reason for the broader FWHMs26 over Pd/OMS-2-300, Pd/OMS-2-400 and Pd/OMS-2-500. Combined with our XRD (Fig. 2) and HR-TEM analysis (Fig. 4), no obvious sintering of the palladium particles could be observed from the Pd/OMS-2-600, due to the low content of palladium. However, sintering of the OMS-2 structure, phase transformation and changes in surface chemical properties (for example, the spill-over of Pd from the 2 × 2 tunnels to the surface) at 600 °C are also potential reasons for the deactivation of Pd/OMS-2-600. In conclusion, an elevated calcination temperature from 300 to 500 °C is preferred to enhance the redox activity of palladium species, while a higher calcination temperature above 600 °C may weaken the palladium–support interaction. While it is hard to determine the key factors for the enhanced activity of Pd/OMS-2-500 and deactivation of Pd/OMS-2-600 from H2-TPR and XPS analysis.
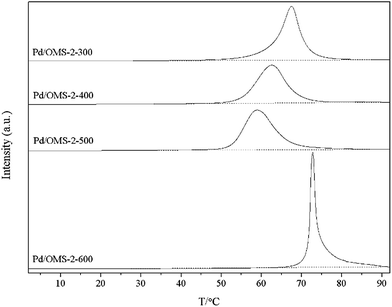 |
| Fig. 8 Low-temperature H2-TPR profiles of the various samples. | |
Table 3 Peak positions and areas of H2 consumption over various samples
Samples |
Peak position (°C) |
Area |
Pd/OMS-2-300 |
67.5 |
1081 |
Pd/OMS-2-400 |
62.6 |
1074 |
Pd/OMS-2-500 |
58.9 |
1075 |
Pd/OMS-2-600 |
72.8 |
999 |
3.7 Raman analysis
Confocal micro-spectroscopy Raman spectra are employed to provide complementary structural information of XRD analysis over various Pd/OMS-2 catalysts. Optical microscope images of various samples are shown in Fig. S7(a), S8(a), S9(a), S10(a) (ESI†). Raman spectra of selected spots1 (Fig. S7(a), S8(a), S9(a), S10(a) (ESI†)) exhibit well-crystalline structure. Distinct peaks of all samples at 636 to 656 cm−1 assignable to the symmetric vibrations of the A1g stretching mode of the lattice Mn–O, indicate the existence of an octahedral MnO6 structure with 2 × 2 tunnels.14,15 It is hard to give qualitative and quantitative analysis results of surface PdO from the present Raman analysis as the influence of the Mn–O A1g mode at 636 to 656 cm−1 approaches the distinctive Raman mode of PdO.27,32 Bands in the region 455–569 cm−1 could be attributed to the asymmetric stretching of bridge oxygen species (Mn–O–Mn), indicating the existence of surface amorphous or semi-crystalline MnxOy species (where 1 < x < 2, 1 < y < 3 and 1 < y/x < 1.5).28 It can be seen from Fig. 9(a) that the peak of Pd/OMS-2-600 at 348 cm−1 is the out-of-plane bending mode of Mn2O3, indicating the presence of Mn2O3.29 Bands of Pd/OMS-2-300 and Pd/OMS-2-400 at 182 cm−1 indicate the presence of an Mn–O–M metal–oxygen chain interacting with the occupation of K+ in the 2 × 2 tunnels.30,31
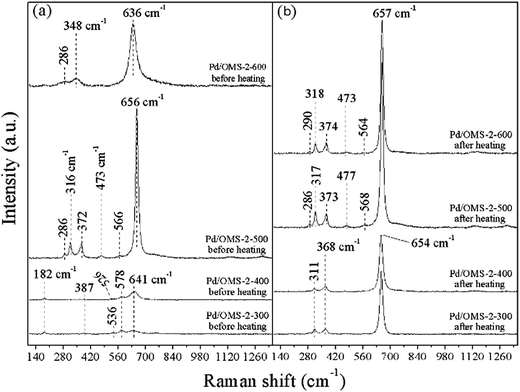 |
| Fig. 9 Raman spectroscopy of various samples (a) before heating (room temperature), (b) cooled to room temperature after being heated at 450 °C (testing conditions: in Ar atmosphere). | |
Shoulders at 578 cm−1 corresponding to the symmetric vibrations of the F2g mode of the lattice Mn–O could be observed from Pd/OMS-2-300 and Pd/OMS-2-400 (Fig. 9(a)),14 indicating the existence of the well-developed tetragonal structure of MnO6 octahedral chains with K+ occupied inside the 2 × 2 tunnels.32,33 This slight F2g mode of the lattice Mn–O peak could not be observed from the spectra of Pd/OMS-2-500 or Pd/OMS-2-600. Moreover, bands of Pd/OMS-2-300 and Pd/OMS-2-400 at 182 cm−1 and 578 cm−1 corresponding to the K+ interacted Mn–O species could not be observed from Pd/OMS-2-300 or Pd/OMS-2-400 after heating. Combined with our XPS analysis (Table 2), it is also inferred that Pd/OMS-2-500 and Pd/OMS-2-600 exhibit an abrupt increase in surface potassium and palladium, i.e. a spill-over of palladium and K+ from the octahedral MnO6-interacted 2 × 2 tunnels, when Pd/OMS-2 was calcined above 400 °C. It should be noted that Pd/OMS-2-500 exhibits no obvious shifts and the peaks disappear before and after being heated at 450 °C in Ar. In other words, compared with Pd/OMS-2-300 and Pd/OMS-2-400, Pd/OMS-2-500 exhibits a higher thermal stability.
4. Discussion
The gradual evolution of surface species and metal–support interactions with the different thermal-treatment temperatures have been characterized by XPS H2-TPR and Raman analysis. Reduction peaks of H2-TPR over the samples shifted to a lower temperature as the thermal-treatment temperature increased from 300 to 500 °C. An elevated calcination temperature up to 500 °C is the potential influencing factor for decreasing palladium (or potassium)–support interaction. Raman analysis indicated the decrease (or disappearance) of bands at 182 cm−1 and 578 cm−1 corresponding to the occupation of K+ in the 2 × 2 tunnels. XPS indicated no obvious proofs of PdSMSI and the differences in surface palladium (and potassium) atomic ratios over all the samples. However, all the samples exhibit the same peak areas of H2-TPR, indicating that all samples have the same content of H2-activated Pd(II) (Table 3). Above all, it could be speculated that the spill-over of potassium and palladium from the tunnels to the outer surface happened with the elevation in calcination temperature from 300 to 500 °C. Despite the same content of total palladium and H2-activated Pd(II), the increase in surface Pd species (proved by XPS analysis) is generally regarded as a key factor in improving CO catalytic oxidation.12,24,25 Combined with the XPS, XRD and H2-TPR analysis, an abrupt increase in surface oxygen and palladium, the phase transformation of K2−xMn8O16 → Mn2O3, as well as an alteration in palladium–support interactions happened when the calcination temperature shifted from 500 to 600 °C. Despite the increase in surface palladium at a thermal-treatment temperature of 600 °C, the sintering effect plays a key role in the deactivation over Pd/OMS-2-600. But it is beyond the scope of the present research to probe the detailed sintering effects on the deactivation of Pd/OMS-2-600 catalysts. Previous research on the catalytic oxidation of CO over manganese oxides and manganese oxides supported noble-metal catalysts has been listed in Table S1 in ESI.†
5. Conclusions
The present research has explored a facile thermal treating process on the catalytic activity of CO oxidation over OMS-2 supported palladium catalysts. A relatively high calcination temperature up to 500 °C is preferred to improve surface palladium atomic ratios and catalytic activity of the Pd/OMS-2 catalyst. In addition, an elevated calcination temperature up to 500 °C led to a decrease in surface Oadsorbed and palladium (or potassium)-support interaction. Spill-over of potassium and palladium happened when the calcination temperature shifted from 400 to 500 °C. A higher calcination temperature above 500 °C may lead to the abrupt alteration of palladium–support interactions and a decrease in the catalytic activity. Phase transformation of K2−xMn8O16 → Mn2O3 happened as well. The present research indicates that an optimal thermal-treatment temperature (500 °C) existed which was attributed to the optimal surface physiochemical properties of Pd/OMS-2-500 and complete CO conversion at 35 °C.
Conflicts of interest
There are no conflicts to declare.
Acknowledgements
This work is supported by the National Natural Science Foundation of China (No. 21666014); The Analysis and Testing Foundation of Kunming University of Science and Technology (No. 2016P201501107004).
References
- R. Prasad and P. Singh, Catal. Rev., 2012, 54(2), 224–279 CAS.
- B. Min and C. FrLiuiend, Chem. Rev., 2007, 107(6), 2709–2724 CrossRef CAS PubMed.
- T. Takei, T. Akita, I. Nakamura, T. Fujitani, M. Okumura, K. Okazaki, J. Huang, T. Ishida and M. Haruta, Adv. Catal., 2012, 55, 1–126 CAS.
- X. Zhang, Z. Qu, F. Yu and Y. Wang, Chin. J. Catal., 2013, 34(7), 1277–1290 CrossRef CAS.
- T. Nguyen, F. Morfin, M. Aouine, F. Bosselet, J. Rousset and L. Piccolo, Catal. Today, 2015, 253, 106–114 CrossRef CAS.
- C. Wang, A. J. Binder, T. J. Toops, J. Lauterbach and E. Sasmaz, Emiss. Control Sci. Technol., 2017, 3, 37–46 CrossRef CAS.
- S. Kato, R. Fujimaki, M. Ogasawara, T. Wakabayashi, Y. Nakahara and S. Nakata, Appl. Catal., B, 2009, 89, 183–188 CrossRef CAS.
- S. Wang, N. Li, L. Luo, W. Huang, Z. Pu, Y. Wang, G. Hu, M. Luo and J. Lu, Appl. Catal., B, 2014, 144, 325–332 CrossRef CAS.
- C. Zhang, C. Wang, W. Zhan, Y. Guo, Y. Guo, G. Lu, A. Baylet and A. Giroir-Fendler, Appl. Catal., B, 2013, 129, 509–516 CrossRef CAS.
- X. Chen, Y. Shen, S. Suib and C. Young, Chem. Mater., 2002, 14, 940–948 CrossRef CAS.
- Q. Zhang, Q. Liu, P. Ning, X. Liu, L. Xu, Z. Song, Y. Duan and T. Zhang, Res. Chem. Intermed., 2017, 43(4), 2017–2032 CrossRef CAS.
- S. Hinokuma, H. Fujii, M. Okamoto, K. Ikeue and M. Machida, Chem. Mater., 2010, 22, 6183–6190 CrossRef CAS.
- S. Hinokuma, H. Fujii, Y. Katsuhara, K. Ikeue and M. Machida, Catal. Sci. Technol., 2014, 4, 2990–2996 CAS.
- C. Calvert, R. Joesten, K. Ngala, J. Villegas, A. Morey, X. Shen and S. L. Suib, Chem. Mater., 2008, 20, 6382–6388 CrossRef CAS.
- S. Dharmarathna, C. K. King’ondu, W. Pedrick, L. Pahalagedara and S. L. Suib, Chem. Mater., 2012, 24(4), 705–712 CrossRef CAS.
- J. Hou, Y. Li, L. Liu, L. Ren and X. Zhao, J. Mate. Chem. A, 2013, 1(23), 6736–6741 RSC.
- K. Priolkar, P. Bera, P. Sarode, M. Hegde, S. Emura, R. Kumashiro and N. P. Lalla, Chem. Mater., 2002, 14, 2120–2128 CrossRef CAS.
- S. Sharma, B. Devu Mukri and M. S. Hegde, Dalton Trans., 2011, 40, 11480–11489 RSC.
- Y. Zhou, X. Liu, Q. Zhang, Q. Liu, Z. Song and P. Ning, Res. Chem. Intermed., 2017, 43(22), 1–19 Search PubMed.
- X. Meng, J. Zhang, B. Chen, Z. Jing and P. Zhao, Catal. Sci. Technol., 2016, 6, 890–896 CAS.
- E. S. Ilton, J. E. Post, P. J. Heaney, F. T. Ling and S. N. Kerisit, Appl. Surf. Sci., 2016, 366, 475–485 CrossRef CAS.
- Q. Zhang, X. Liu, P. Ning, Z. Song, H. Li and J. Gu, Catal. Sci. Technol., 2015, 5, 2260–2269 CAS.
- X. Liu, P. Ning, L. Xu, Q. Liu, Z. Song and Q. Zhang, RSC Adv., 2016, 6, 41181–41188 RSC.
- A. I. Boronin, E. M. Slavinskaya, I. G. Danilova, R. V. Gulyaev, Y. I. Amosov, P. A. Kuznetsov, I. A. Polukhina, S. V. Koscheev, V. I. Zaikovskii and A. S. Noskov, Catal. Today, 2009, 144, 201–211 CrossRef CAS.
- E. M. Slavinskaya, R. V. Gulyaev, A. V. Zadesenets, O. A. Stonkus, V. I. Zaikovskii, Y. V. Shubin, S. V. Korenev and A. I. Boronin, Appl. Catal., B, 2015, 91, 166–167 Search PubMed.
- L. Meng, A. P. Jia, J. Q. Lu, L. F. Luo, W. X. Huang and M. F. Luo, J. Phys. Chem. C, 2011, 115(40), 19789–19796 CAS.
- A. Baylet, P. Marécot, D. Duprez, P. Castellazzi, G. Groppi and P. Forzatti, Phys. Chem. Chem. Phys., 2011, 13, 4607–4613 RSC.
- J. Xu, Y. Deng, X. Zhang, Y. Luo, W. Mao, X. Yang, L. Ouyang, P. Tian and Y. Han, ACS Catal., 2014, 4, 4106–4115 CrossRef CAS.
- J. Xu, Y. Deng, Y. Luo, W. Mao, X. Yang and Y. Han, J. Catal., 2013, 300, 225–234 CrossRef CAS.
- C. Wang, J. Ma, F. Liu, H. He and R. Zhang, J. Phys. Chem. C, 2015, 119, 23119–23126 CAS.
- S. Cheng, L. Yang, D. Chen, X. Ji, Z. Jiang, D. Ding and M. Liu, Nano Energy, 2014, 9, 161–167 CrossRef CAS.
- L. Liu, Y. Song, Z. Fu, Q. Ye, S. Cheng, T. Kang and H. Dai, Appl. Surf. Sci., 2017, 396, 599–608 CrossRef CAS.
- R. Wang and J. Li, Environ. Sci. Technol., 2010, 44, 4282–4287 CrossRef CAS PubMed.
Footnote |
† Electronic supplementary information (ESI) available. See DOI: 10.1039/c7ra07814e |
|
This journal is © The Royal Society of Chemistry 2017 |
Click here to see how this site uses Cookies. View our privacy policy here.