DOI:
10.1039/C7RA06427F
(Paper)
RSC Adv., 2017,
7, 41929-41935
Preparation and properties of poly (lactic acid)/magnetic Fe3O4 composites and nonwovens
Received
8th June 2017
, Accepted 16th August 2017
First published on 30th August 2017
Abstract
To develop degradable and magnetic PLA-based melt-blown (MB) nonwoven materials for air filtration applications, poly (lactic acid) (PLA)/magnetic Fe3O4 (PLA/Fe3O4) MB nonwovens were obtained by melt blowing using the PLA/Fe3O4 composites with different components prepared by melt-mixing as the masterbatch. The crystallization and melting behavior of the composites were examined with differential scanning calorimetry (DSC). Although the results showed that the Fe3O4 particles obviously hindered the cold crystallization process of PLA and the relative crystallinity degree of the composites decreased due to the addition of Fe3O4; there was no overall change in the crystal structure of PLA according to the wide-angle X-ray diffraction (XRD). Thermogravimetric analysis (TGA) and dynamic rheological measurements demonstrated that the introduction of Fe3O4 reduced the thermal stability of PLA. The effect of Fe3O4 particles on the morphology of the PLA/Fe3O4 composites and MB nonwovens was characterized by scanning electron microscopy (SEM). It revealed that the Fe3O4 particles were dispersed in a PLA mixture with the “sea-island” structure, and the addition of Fe3O4 particles also made the fiber surface rough and the nonwoven web turned fluffy to some extent. The magnetic properties and filtration performance of the MB nonwovens were also investigated in detail.
Introduction
With the increasing attention to air filtration materials, melt-blown (MB) nonwoven has drawn people's interest in recent years owning to its highly effective filtration performance and barrier properties.1,2 Currently, the widely used raw materials for MB nonwovens are polypropylene (PP) and polyethylene terephthalate (PET) but the disposal and subsequent influence on the environment are extremely serious because of their nonbiodegradable nature. Moreover, the eco-friendly bio-based polymers as an answer to the growing sustainability and environmental issues associated with conventional non-biodegradable petroleum-based materials have received increasing interest due to their recyclable, renewable and biodegradable characteristics.3,4 Thus, a new strategy has emerged to develop bio-based polymer materials for MB nonwoven.5
Among many bio-based polymers, poly (lactic acid) (PLA), one of the typical plant-derived biodegradable polymers, can be derived from renewable plant resources (mainly starch and corn or sugar).6 Owing to its excellent biocompatibility, better thermoplastic processability and reasonably good mechanical properties compared with other bio-derived polymers,11,12 it shows a tremendous application and huge commercial market prospect for fibers, film materials, and packaging materials.7–10 Several researchers have focused on the processability and service performance of PLA as a raw material for MB nonwoven;5,13 the structure and properties of PLA chips and PLA-based blends for melt blowing nonwovens were also analyzed in our previous research.14,15 All these studies indicated that PLA showed an explosive application prospect in the melt blown nonwoven material.
Melt-mixing process is a rapid method to obtain composite materials; it has the advantages of high production efficiency, less pollution and simple operation. Composting PLA with functionalized inorganic nanofillers by melt-mixing not only presents a simple but effective way to improve the properties of PLA matrix but also endows some added functionalities, such as impressive electrical conductivity, antimicrobial activity, and gas barrier properties,16–21 which open a promising development and application area of PLA. Ferroferric oxide (Fe3O4) as one of the functional materials has been widely used in microwave absorbing materials, catalyst carriers and biomedical fields due to its superior magnetic responsiveness, low toxicity and biocompatibility.22–26 Interestingly, though there are some studies have demonstrated the PLA based Fe3O4 composite prepared by the solution-mixing method and the usage were almost for biomaterials,27–30 little research has studied the PLA/Fe3O4 composites prepared by melt-mixing and the influence of magnetic Fe3O4 on the filtration property of PLA MB nonwoven materials.
In our study, we have hoped to provide some original data for the development of degradable and functional PLA-based MB nonwoven materials for air filtration application. Therefore, magnetic PLA/Fe3O4 MB nonwoven materials were fabricated by melt-blowing processing using the PLA/Fe3O4 composites as the raw materials that were prepared and pelletized by melt-mixing. Some correlative performances were characterized to evaluate the influence of Fe3O4 particles on the properties of PLA.
Experimental
Materials
A commercial PLA (trade name 6252D, about 6.86% D-isomer lactide) used for MB nonwoven processing was supplied by NatureWorks LLC (USA) and the melt index (MI) was about 120 g min−1 at 210 °C; Fe3O4 particle with a mean particle diameter of about 600 nm was provided by the Hebei Lingshou Mineral Powder Factory (China).
Preparation of PLA/Fe3O4 MB nonwoven
The melt-blowing process was performed in four steps. Prior to the processing, both PLA resins and Fe3O4 particles were dried in a vacuum oven at 80 °C for 12 h. After the mixture of PLA and Fe3O4 was mixed in the mass ratio of 100/0, 100/1, 100/3, and 100/5 by a mechanical mixer (SHR series high speed mixer, Giant company, Nanjing, China, rotate speed 1000 rpm), the PLA/Fe3O4 composites were prepared by feeding the mixture to a twin-screw extruder (TSE-30A, the ratio of length and diameter of the screw is 40
:
1, Nanjing Ruiya Extrusion System Co., Ltd, China) at 180 °C and 300 rpm; the composites with 0, 1, 3, 5 wt% content Fe3O4 were signed as C0, C1, C3, and C5, respectively. Finally, the composites were fed to a 45 cm wide research line with a single coat-hanger die (the diameter of each hole is 0.3 mm and the air-gap distance is 1.5 mm) melt-spun machine (Non-woven Research Laboratory of Zhejiang Sci-Tech University, Hangzhou, Zhejiang, China) to obtain the PLA/Fe3O4 MB nonwovens. The die temperature was in range of 200–230 °C and the temperature of hot air was 270 °C with a drawing air flow press of 0.08 MPa, 10 Hz metering pump frequency and a die to collector distance of 30 cm. The corresponding MB nonwovens were signed as M0, M1, M3, and M5. The preparation process of PLA/Fe3O4 nonwovens is shown in Fig. 1.
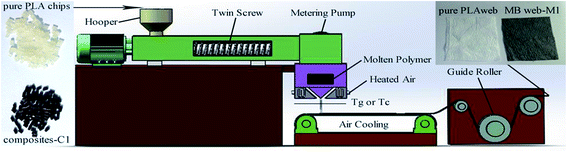 |
| Fig. 1 The preparation process of PLA/Fe3O4 nonwovens. | |
Characterization
Differential scanning calorimetry (DSC). The thermal properties of PLA/Fe3O4 composites were investigated by a DSC (DSC8000, Perkin-Elmer, US). All the samples were heated from 25 to 200 °C at 100 °C min−1 and maintained at 200 °C for 3 min to erase the previous thermal history, and then cooled down to 25 °C at 10°C min−1. Finally, the samples were heated from 25 to 200 °C at 10°C min−1 once more to evaluate the second melting behavior and all experiments were performed under nitrogen atmosphere. It was obviously possible to obtain the glass transition temperature (Tg), the cold crystallization temperature (Tcc), the melt temperature (Tm), the cold crystallization enthalpy (ΔHcc) and the melting enthalpy (ΔHm) from the second heating curves of the samples. The relative degree of crystallinity (Xc) of all the samples was calculated using the equation, Xc = {(ΔHm − ΔHcc) ÷ ΔHo} × 100%, where ΔHm and ΔHcc were the melting enthalpy and the cold crystallization enthalpy of the samples respectively, ΔHo was the fusion enthalpy of the completely crystalline PLA (the value taken as 93 J g−1 from literature),31 and C was the weight fraction of PLA in the composites.
Wide-angle X-ray diffraction (XRD). The crystalline structure of PLA/Fe3O4 composites was evaluated by an X-ray diffractometer (D8 DISCOVERY, Bruker AXS, Geman) using a CuKα1 (=1.5406 Å) radiation source in the diffraction angle (2θ) range of 5–50° by steps of 5° min−1 at 40 kV and 40 mA. All the sample specimen dimensions were made approximately 1 × 40 × 40 mm (thickness–width–length) using the melt calendaring method at 190 °C, 10 MPa. Then, these plastic sheets were placed at room temperature for 48 hours before testing.
Thermogravimetric analysis (TGA). The thermal stability of PLA/Fe3O4 composites was studied on a TGA (TGA-Q50, TA Instruments, US) by scanning from 30 °C to 600 °C at a heating rate of 20°C min−1 under a nitrogen atmosphere.
Dynamic rheological measurements. The dynamic rheological behavior of PLA/Fe3O4 composites was determined by a Anton Paar Physica MCR 301 rheometer at 180 °C in the frequency scan range 0.1–400 rad s−1. The measurements were performed in the linear viscoelastic region with dynamic oscillatory mode and 25 mm parallel cone-plate with gap setting of about 0.4 mm.
Scanning electron microscopy (SEM). The fracture surface morphology of the PLA/Fe3O4 composites and the surface morphology of the corresponding MB nonwovens were analyzed by scanning electron microscopy (SEM, ULTRA55, Carl Zeiss AG, Germany) using a 3 kV accelerating voltage. Prior to the testing, the composite specimens were brittle fractured with liquid nitrogen, and all the composites and MB nonwoven samples were sputtered with gold to provide enhanced conductivity.
Magnetic property testing. A micro-vibration sample magnetometer (VSM 7407, Lakeshore, US) was used to measure the magnetic hysteresis loops of PLA/Fe3O4 MB nonwovens in the solid state at room temperature with an external 2 T magnetic field.
Filtration performance testing. The filtration efficiency of the PLA/Fe3O4 MB nonwovens was measured by an electron-laser particle spectrometer (WPS-1000xp, MSP Corp). The testing aerosol was sodium chloride (NaCl in the range of 0.3–0.5 μm) with 10
000 grains per m3 at 5.3 cm s−1 velocity in air. The mass concentration of NaCl in the upstream volume (Nu) and the downstream volume of the air (Nd) was detected by an electron-laser particle spectrometer. The filtration efficiency (E) was calculated by the equation, E = {(Nu − Nd) ÷ Nu} × 100%. Five replicates were performed on each sample and the average values were recorded.
Result and discussion
Thermal properties of PLA/Fe3O4 composites
The second heating scanning curves of PLA/Fe3O4 composites were detected, as shown in Fig. 2a. Meanwhile, the glass transition temperature (Tg), cold crystallization temperature (Tcc), melt temperature (Tm) and relative crystallinity degree (Xc) of the samples determined from the DSC curves are summarized in Table 1. As seen from Table 1, Tg of pure PLA was about 61.5 °C, and Tg of all its composites were approximately 61 °C, suggesting that the addition of Fe3O4 had no significant change on the Tg of PLA. During the process of continuous heating, all the samples showed an exothermic peak corresponding to the cold crystallization process, which was caused by the mobility due to the rearrangement of PLA macromolecules. The relationship between Tcc and the content of Fe3O4 is plotted in Fig. 2b. Evidently, Tcc of the composites shifted to a higher temperature compared to neat PLA as the Fe3O4 content increased, which indicated that the Fe3O4 particles played an obvious hindrance for cold crystallization process of PLA. Moreover, the crystallinity degree of the composites decreased with the addition of Fe3O4. There were two aspects that might be taken into account to explain this phenomenon. On one side, Fe3O4 particles may disperse into the macromolecular chains, possibly disrupting the regularity of the PLA chain structure and hampering the crystallization process. On the other side, the concentration of Fe3O4 particles could act as blocking sites to hinder the growth of PLA crystallites through strengthening the molecular chain rigidity and decreasing the mobility, thus also resulting in a stronger impediment effect on the crystallization process.32–34 All the samples had two distinct melting peaks; the mechanisms of dual (or multiple) lamellae population or crystal structure and melt-recrystallization could be used to explain the melting behavior of PLA.35 The dual (or multiple) lamellae population or crystal structure mechanism considered the multiple melting with the different morphologies or crystal structures formed during the continue heating process, which may lead to the appearance of multiple melting peaks. In addition, the melt-recrystallization explanation suggests that the two separated peaks in the curves can be attributed to the melting of original crystals and the crystals re-formed through the melt-recrystallization process, and the crystallization of PLA at higher melting peak temperature were more perfect. Although the Tm of all the composites increased about 2 °C compared to neat PLA to some extent, the overall change was not notable and the Tm of all the samples were 164°.
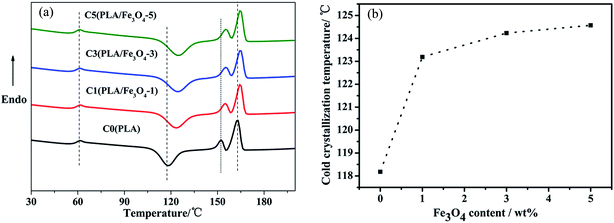 |
| Fig. 2 (a) DSC curves of the second heating for PLA/Fe3O4 composites; (b) the cold crystallization temperature of the composites with different Fe3O4 content. | |
Table 1 The related thermal results of PLA/Fe3O4 compositesa
Sample |
Tg (°C) |
Tcc (°C) |
Tm1 (°C) |
Tm2 (°C) |
Xc (%) |
Data were summarized from the second heating cycles in DSC. |
C0(PLA) |
61.45 |
118.18 |
152.36 |
162.80 |
3.93 |
C1(PLA/Fe3O4-1) |
61.49 |
123.19 |
154.96 |
164.61 |
1.15 |
C3(PLA/Fe3O4-3) |
61.30 |
124.23 |
155.25 |
164.72 |
0.80 |
C5(PLA/Fe3O4-5) |
61.17 |
124.57 |
155.32 |
164.74 |
0.78 |
Effect of Fe3O4 on the crystal structure
The XRD curves of Fe3O4 and PLA/Fe3O4 composites are illustrated in Fig. 3. Some research has been reported that the diffraction peaks of PLA homo-crystallites may exist at 2θ values of 16°, 18.4°, and 21.8°.36 While our samples used for XRD test were obtained by melt calendaring method, it was difficult to form an ordered structure at 190 °C (>Tm). Moreover, the ability of the chain segment rearrangement cooled by air after melting to form crystals was limited due to the slow crystallization rate of PLA. Hence, a small and weak peak of pure PLA appeared at 2θ value of 16.8° corresponding to the (110) phase and the crystallinity was only 0.2%, indicating that neat PLA was almost amorphous. With the addition of Fe3O4, all the composites exhibited a wide angle diffraction peak of PLA, disclosing that the composites were also amorphous. The main peaks at 2θ values of 18.2°, 30.2°, and 35.4°, 43.1° seen from the XRD curve individually correspond to the (110), (220), (311), and (400) planes of Fe3O4, and all these peaks in the XRD pattern were indexed as face-centered cubic particles of Fe3O4 (JCPDS no. 16-0629); the average crystallite size of Fe3O4 particles was 31.1 (±0.9) nm, which was calculated from the Debye-Scherrer equation, D = (Kλ) ÷ {β(2θ) × cos
θ}, where β(2θ) was the full width at half-maximum (FWHM), K was a constant taken as the normal value of 0.9, and θ was the Bragg angle.37 Obviously, the diffraction peak intensity of PLA in the composites did not increase with the increase in the content of Fe3O4; in contrast, all of them became smaller and wider. The reason may be that the addition of Fe3O4 hindered the arrangement of PLA molecular order and inhibited the formation of some PLA crystals, which was consistent with the result of DSC, but the crystalline structure of PLA had no change.
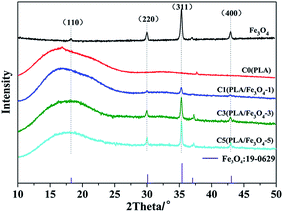 |
| Fig. 3 XRD curves of Fe3O4, PLA and PLA/Fe3O4 composites. | |
Effect of Fe3O4 on the thermal stability and dynamic rheological property
The TGA curves of PLA/Fe3O4 composites are plotted in Fig. 4. The decomposition temperature at 5 wt% and 50% weight loss (recorded as T0.05 and T0.50, respectively), the maximum decomposition temperature (Tmax) and the remnant mass (the content of Fe3O4 can be calculated from the remnant mass) at the end of the curves at 600 °C are listed in Table 2. The weight loss curves of all the samples exhibited one simple thermal decomposition step within the temperature range of 280–390 °C. In fact, it was obviously observed that the temperature at 5 wt% weight loss of the composites, which can be used to evaluate the effect of Fe3O4 particles on the thermal stability, shifted downwards compared to neat PLA. This revealed that the thermal stability became worse with loading Fe3O4. It was possibly related to the adsorbed and absorbed water on the particles' surface; the active sites on their surface also could act as depolymerisation catalysts to accelerate the degradation of PLA.38 All these factors may have caused the decrease in the molecular weight and promoted the degradation of PLA, which would reduce the viscosity of material under processing during a long time effect of heat and oxygen to some extent, it can be confirmed by the dynamic rheological curves shown in Fig. 5. It can be seen that all the samples demonstrated rather similar rheological properties, which exhibited non-newtonian shear thinning behaviors. However, the complex viscosity of all the composites was lower than that of pure PLA, and the phenomenon was more obvious with the addition of Fe3O4. Despite the addition of Fe3O4 accelerating the thermal degradation of PLA, it can still satisfy with the temperature window (185–240 °C) required for melt-blown processing.39
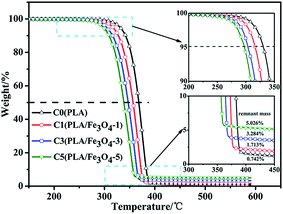 |
| Fig. 4 TGA curves of pure PLA and the composites. | |
Table 2 TGA results of PLA/Fe3O4 composites
Sample |
T0.05 (°C) |
T0.50 (°C) |
Tmax (°C) |
Remnant mass (%) |
C0(PLA) |
331.35 |
367.46 |
392.89 |
0.74 |
C1(PLA/Fe3O4-1) |
316.51 |
357.06 |
381.52 |
1.71 |
C3(PLA/Fe3O4-3) |
303.50 |
346.66 |
370.77 |
3.28 |
C5(PLA/Fe3O4-5) |
297.60 |
338.17 |
363.54 |
5.02 |
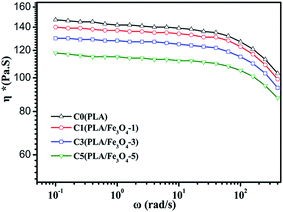 |
| Fig. 5 Complex viscosity of PLA/Fe3O4 composites at 180 °C. | |
Morphology of PLA/Fe3O4 composites and MB nonwovens
The SEM micrographs of the fracture surface of PLA/Fe3O4 composites are shown in Fig. 6A and the morphology of PLA/Fe3O4 MB nonwovens in Fig. 6B. In fact, during the process, partly agglomerated particles could be effectively separated if they withstood a strong shear force during the mixture and polymer processing through the screw,32 which could improve the dispersion of particles in the PLA phase to some extent. Hence, it can be seen that the particles were distributed homogeneously within the PLA matrix in the form of “sea-island” structure. Moreover, with the content of fillers increasing, the “sea-island” structure between Fe3O4 particles and PLA was clear, which showed the poor adhesion ability between PLA phase and Fe3O4 particles, leading to some big particles falling off from the polymer after been brittle fractured with liquid nitrogen and forming some holes. This phenomenon could be obviously observed with the high loading content of Fe3O4 particles (3 wt% and 5 wt%) according to Fig. 6A(c) and (d). While the fiber of pure PLA MB nonwoven prepared by melt-blowing processing using the PLA extrusion strip as the master batch was relatively uniform with a mean diameter of 0.6–1.5 μm, as shown in Fig. 6B(e), the fiber surfaces were sooth and the melt-blown web was dense. However, the fiber diameter of MB nonwovens became non-uniform and the web turned fluffy on loading Fe3O4 particles. Some Fe3O4 particles with the small size wrapped in the fibers during the melt blowing process; on the other hand, the larger particles were exposed on the surface of the fibers, which made the fiber surface become rough.
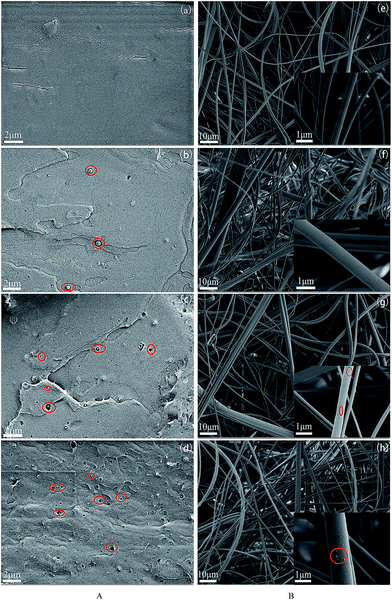 |
| Fig. 6 (A) The fracture surface of PLA/Fe3O4 composites: (a) C0 (Pure PLA), (b) C1 (PLA/Fe3O4-1), (c) C3 (PLA/Fe3O4-3), (d) C5 (PLA/Fe3O4-5); (B) the morphology of the PLA/Fe3O4 MB nonwovens: (e) M0 (pure PLA), (f) M1 (PLA/Fe3O4-1), (g) M3 (PLA/Fe3O4-3), (h) M5 (PLA/Fe3O4-5). | |
Magnetic property analysis of PLA/Fe3O4 MB nonwovens
The magnetization hysteresis loops of PLA/Fe3O4 MB nonwovens are measured and shown in Fig. 7 to reveal their magnetic characterization. All the PLA/Fe3O4 MB nonwovens achieved their saturation magnetization (Ms), which was the maximum magnetization intensity corresponding to the value of the atom magnetic moment completely displayed in the same direction. With the addition of Fe3O4 particles increasing from 1 wt% to 5 wt%, the Ms of the MB nonwovens increased from 0.056 to 0.221 emu/g, while the Ms of pure PLA MB nonwoven was maintained at 0 because it had no magnetic response. The hysteresis curves showed that the PLA/Fe3O4 MB nonwovens had certain remanence (Mr) and coercivity (Hc). These values were small, which indicated that all the prepared PLA/Fe3O4 MB nonwovens were paramagnetic. It can be seen that the PLA/Fe3O4-1 MB nonwoven strip exhibited good magnetic response under the action of magnetic attraction; in contrast, there was no response of the pure PLA MB nonwoven strip. The MB nonwovens still owed certain magnetic response after the melt-blending and melt-blowing processing.
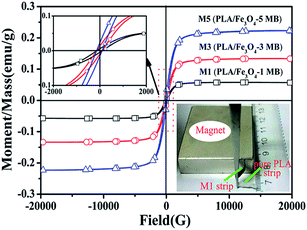 |
| Fig. 7 The magnetization hysteresis loops of PLA/Fe3O4 MB nonwovens. | |
Filtration property of PLA/Fe3O4 MB nonwovens
Fig. 8 describes the filtration property of the pure PLA and the PLA/Fe3O4 MB nonwovens. Compared to the pure PLA MB nonwovens, the filtration efficiency of all the composite MB nonwovens increased from 42% to 68% as the content of Fe3O4 particles added increased from 0% to 5%. The morphology of PLA/Fe3O4 MB nonwovens indicated that the web turned fluffy and the fiber surface became rough on loading Fe3O4 powder, which could result in the testing particles getting impacted and captured more easily by the combined effects of sieving, diffusion, inertia and interception.40 Moreover, the addition of Fe3O4 particles also affected the electrostatic and magnetic adsorption on some charged particles in air, which can also improve the filtration efficiency of the MB nonwovens.
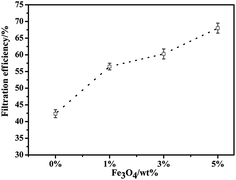 |
| Fig. 8 The filtration efficiency curve of PLA/Fe3O4 MB nonwovens. | |
Conclusion
In this study, PLA/Fe3O4 composites with different contents of magnetic Fe3O4 particles were prepared by melt-mixing process using a twin-screw extruder. Then, the MB nonwovens were obtained by melt blowing. Some measurements were conducted to evaluate the effect of magnetite on the composites and melt-blown materials in detail. The DSC results showed that Fe3O4 particles had an inhibition for the cold crystallization process of PLA, but the crystalline structure of PLA was unchanged. Though TGA and dynamic rheological measurements indicated the introduction of Fe3O4 reduced the thermal stability of the PLA, the decomposition temperature of the composites decreased as the content of Fe3O4 increases, it still could be satisfied with the temperature window required for melt-blowing process. According to the SEM observation, the Fe3O4 particles were uniformly dispersed in the PLA mixture in the form of the “sea-island” structure, and the particles also made the fiber surface become rough and the nonwoven web turned fluffy to some extent. Magnetic property and filtration performance testing on the MB nonwovens showed that the Fe3O4 particles not only endowed the PLA/Fe3O4 MB nonwovens with certain magnetic response after the melt-blending and melt-blowing processing a but also improved the filtration efficiency of PLA nonwovens, which showed a potential usage in the field of filter materials. This provided a method to develop functional PLA based composites, which can be applied in MB nonwoven materials for air filtration application. However, to obtain the PLA/Fe3O4 MB nonwovens with excellent performance, there were still some factors to be considered in the next works, such as the dispersion of Fe3O4, the compatibility between PLA matrix and Fe3O4 particles, and the different melt-blowing process condition.
Conflicts of interest
There are no conflicts to declare.
Acknowledgements
This work was financially supported by the Sci-Tech Plan Project of Zhejiang Province [Project No.: 2017C33077], the National Natural Science Foundation of China [grant number: 51203141] and 521 Talent Project of ZSTU.
References
- M. A. Hassan, N. Anantharamaiah, S. A. Khan and B. Pourdeyhimi, Ind. Eng. Chem. Res., 2016, 55, 2049–2058 CrossRef CAS.
- C. J. Ellison, A. Phatak, D. W. Giles, C. W. Macosko and F. S. Bates, Polymer, 2007, 48, 3306–3316 CrossRef CAS.
- A. K. Mohanty, M. Misra and L. T. Drzal, J. Polym. Environ., 2002, 10, 19–26 CrossRef CAS.
- T. Iwata, Angew. Chem., Int. Ed., 2015, 54, 3120–3215 CrossRef PubMed.
- D. H. Muller and A. Krobjilowski, Int. Nonwovens J., 2001, 11 Search PubMed.
- C. Liu, K. W. Chen, J. Shen, H. M. Wong, K. W. K. Yeung and S. C. Tjong, RSC Adv., 2015, 5, 72288–72299 RSC.
- L. T. Lim, R. Auras and M. Rubino, Prog. Polym. Sci., 2008, 33, 820–852 CrossRef CAS.
- M. Jamshidian, E. A. Tehrany, M. Imran, M. Jacquot and S. Desobry, Compr. Rev. Food Sci. Food Saf., 2010, 9, 552–571 CrossRef CAS.
- B. Mallet, K. Lamnawar and A. Maazouz, Polym. Eng. Sci., 2014, 54, 840–857 CAS.
- Y. Chen, L. M. Geever, J. A. Killion, J. G. Lyons, C. L. Higginbotham and D. M. Devine, Polym.-Plast. Technol. Eng., 2016, 55, 1057–1075 CrossRef CAS.
- V. H. Sangeetha, H. Deka, T. O. Varghese and S. K. Nayak, Polym. Compos., 2016 DOI:10.1002/pc.23906.
- A. N. Frone, D. M. Panaitescu, I. Chiulan, C. A. Nicola, Z. Vuluga, C. Vitelaru and C. M. Damian, J. Mater. Sci., 2016, 51, 9771–9791 CrossRef CAS.
- Y. Liu, B. Cheng and G. Cheng, Text. Res. J., 2010, 80, 771–778 CrossRef CAS.
- B. Yu, J. Han, H. Sun, F. Zhu, Q. Zhang and J. Kong, Polym. Compos., 2014, 36, 264–271 CrossRef.
- B. Yu, H. Sun, Y. Cao, J. Han, J. Kong, P. Wang and F. Zhu, Polym.-Plast. Technol. Eng., 2014, 53, 1788–1793 CrossRef CAS.
- I. H. Kim and Y. G. Jeong, J. Polym. Sci., Part B: Polym. Phys., 2010, 48, 850–858 CrossRef CAS.
- H. Liu, D. Bai, H. Bai, Q. Zhang and Q. Fu, J. Mater. Chem. A, 2015, 3, 13835–13847 CAS.
- C. Huang, H. Bai, H. Xiu, Q. Zhang and Q. Fu, Compos. Sci. Technol., 2014, 102, 20–27 CrossRef CAS.
- S. Davoodi, E. Oliaei, S. M. Davachi, I. Hejazi, J. Seyfi, B. S. Heidari and H. Ebrahimi, RSC Adv., 2016, 6, 39870–39882 RSC.
- R. Scaffaro, L. Botta, A. Maio, M. C. Mistretta and F. P. L. Mantia, Materials, 2016, 9, 351 CrossRef PubMed.
- E. Picard, E. Espuche and R. Fulchiron, Appl. Clay Sci., 2011, 53, 58–65 CrossRef CAS.
- X. Jian, B. Wu, Y. Wei, S. Dou, X. Wang, W. He and N. Mahmood, ACS Appl. Mater. Interfaces, 2016, 8, 6101–6109 CAS.
- S. Ni, X. Sun, X. Wang, G. Zhou, F. Yang, J. Wang and D. He, Mater. Chem. Phys., 2016, 124, 353–358 CrossRef.
- S. W. Bian, S. Liu and L. Chang, J. Mater. Sci., 2016, 51, 3643–3649 CrossRef CAS.
- L. He, L. Yao, F. Liu, B. Qin, R. Song and W. Huang, J. Nanosci. Nanotechnol., 2010, 10, 6348–6355 CrossRef CAS PubMed.
- J. Yang, P. Zou, L. Yang, J. Cao, Y. Sun, D. Han, S. Yang, Z. Wang, G. Chen, B. Wang and X. Kong, Appl. Surf. Sci., 2014, 303, 425–432 CrossRef CAS.
- N. Mhlanga, S. S. Ray, Y. Lemmer and J. Wesleysmith, ACS Appl. Mater. Interfaces, 2015, 7, 22692–22701 CAS.
- L. Hosseimi, K. Mahboobnia and M. Irani, Int. J. Polym. Mater., 2016, 65, 176–182 CrossRef.
- H. Deng and Z. Lei, Composites, Part B, 2013, 54, 194–199 CrossRef CAS.
- G. Lv, F. He, X. Wang, F. Gao, G. Zhang, T. Wang, H. Jiang, C. Wu, D. Guo, X. Li, B. Chen and Z. Gu, Langmuir, 2008, 24, 2151–2156 CrossRef CAS PubMed.
- E. Fortunati, I. Armentano, Q. Zhou, D. Puglia, A. Terenzi, L. A. Berglund and J. M. Kenny, Polym. Degrad. Stab., 2012, 97, 2027–2036 CrossRef CAS.
- H. Zhang, J. Huang, L. Yang, R. Chen, W. Zou, X. Lin and J. Qu, RSC Adv., 2015, 5, 4639–4647 RSC.
- Y. Sun and C. He, ACS Macro Lett., 2012, 1, 709–713 CrossRef CAS.
- D. Wu, Y. Cheng, S. Feng, Z. Yao and M. Zhang, Ind. Eng. Chem. Res., 2013, 52, 6731–6739 CrossRef CAS.
- P. Pan, W. Kai, B. Zhu, T. Dong and Y. Inoue, Macromolecules, 2007, 40, 6898–6905 CrossRef CAS.
- X. Wei, R. Bao, Z. Cao, W. Yang, B. Xie and M. Yang, Macromolecules, 2014, 47, 1439–1448 CrossRef CAS.
- D. Zhang, A. B. Karki, D. Rutman, D. Young, A. Wang, D. Cocke, T. H. Ho and Z. Guo, Polymer, 2009, 17, 4189–4198 CrossRef.
- C. Cifuentes, M. Lieblich, F. A. Lópeza, R. Benaventec and J. L. González-Carrascoa, Mater. Sci. Eng., C, 2017, 72, 18–25 CrossRef PubMed.
- J. M. Ferri, M. D. Samper, D. García-Sanoguera, M. J. Reig, O. Fenollar and R. Balart, J. Mater. Sci., 2015, 51, 5356–5366 CrossRef.
- I. M. Hutten, Handbook of Nonwoven Filter Media, Elsevier, New York, 2007 Search PubMed.
|
This journal is © The Royal Society of Chemistry 2017 |
Click here to see how this site uses Cookies. View our privacy policy here.