DOI:
10.1039/C7RA06781J
(Paper)
RSC Adv., 2017,
7, 37412-37418
Direct synthesis of thiol-terminated poly(ε-caprolactone): a study on polymerization kinetics, mechanism and rare earth phenolates' structure–activity relationship†
Received
18th June 2017
, Accepted 17th July 2017
First published on 28th July 2017
Abstract
Herein, chemoselective ring-opening polymerization kinetics, mechanism and rare earth phenolates' structure–activity relationship were investigated for direct synthesis of thiol-terminated poly(ε-caprolactone). Polymerization kinetics was measured using lanthanide tris(2,6-di-tert-butyl-4-methylphenolate) and unprotected 6-mercapto-1-hexanol as model catalyst/initiator. The fraction of thiol-terminated poly(ε-caprolactone) in the product (thiol fidelity) first decreased with the increasing consumption of monomers and then increased afterwards. The degree of polymerization and molecular weight distribution increased continuously with the reaction time. The proposed polymerization mechanism is presented herein. Active site of O–La bond was generated via chemoselective ligand exchange between phenol and hydroxyl groups of the initiator, leading to designable polymers. A small amount of thiolester intermediates was yielded by monomer insertion into the S–La bond and disappeared through transesterification attacked by the O–La bond, which was confirmed by NMR, MALDI-TOF MS and SEC. The structure–activity relationship was investigated by employing 14 catalysts with varied substituted ligands and center metals. All catalysts exhibited good activities to achieve over 75% thiol fidelities and controlled molecular weights and distributions. 2,6-Di-tert-butyl-4-methylphenol and lanthanide showed relatively higher chemoselectivity. Well-defined thiol-terminated poly(ε-caprolactone) with a molar mass up to 20
000 g mol−1 was prepared under mild conditions. This study provides deep insights into chemoselective polymerization and rare earth phenolate catalysis.
Introduction
By virtue of excellent biodegradability, biocompatibility and special properties of the end group, thiol-terminated polyesters have broad applications in biotechnology, microelectronics, material science, etc.1–7 Owing to the active chemical and physical properties of thiol, it was challenging to directly produce polyesters containing thiol functionality via ring-opening polymerization (ROP) of cyclic monomers in the presence of mercapto alcohol used as a multifunctional initiator.8,9 Indirect synthetic methods were used to yield target products by employing tedious protecting/deprotecting steps.10–12
Since the discovery of Candida antarctica lipase B (CALB), a direct strategy for synthesis of thiol-terminated polyesters was established without any protecting group.13 To date, chemoselective catalysts of Sn(OTf)2,14 rare earth phenolates15,16 and lipase from Candida sp. 99–125,17 as well as microflow technology,18 were developed to promote protecting-group-free approach. However, there were still some drawbacks, including poor control of thiol fidelity, molecular weight and distribution.
Previously, we have reported rare earth phenolates as highly chemoselective and low-cost catalysts for unprotected mercapto alcohol-initiated polymerization of ε-caprolactone (CL) to linear and branched thiol-functionalized poly(ε-caprolactone).15,16 In the absence of the thiol group, experimental and computational studies have strongly proved the living/controlled nature of ring-opening polymerization through coordination–insertion mechanism.19–27 For the system containing the thiol group, the polymerization kinetics, mechanism and catalyst's structure–activity relationship were unknown, which were critical for obtaining more understanding of the catalyst design.
In this contribution, chemoselective ring-opening polymerization kinetics was carefully measured and the proposed polymerization mechanism is presented with the support of NMR, MALDI-TOF MS and SEC. The structure–activity relationship study revealed that 2,6-di-tert-butyl-4-methylphenol and lanthanide exhibited relatively higher chemoselectivity. Moreover, well-defined thiol-terminated poly(ε-caprolactone) (PCLSH) with a molar mass up to 20
000 g mol−1 was obtained under optimized mild conditions. This study provides deep insights into chemoselective polymerization and rare earth phenolate catalysis.
Experimental
Materials
CL (J&K, 99%) was dried over CaH2 overnight, vacuum-distilled and stored at room temperature. Toluene (Sinopharm chemical Reagent, 99.5%) was refluxed over sodium under an argon atmosphere. 6-Mercapto-1-hexanol (MH) (TCI, 98%) and other chemicals were purchased and used without purification. The rare earth phenolates were synthesized according to the literature.21
General polymerization procedures
Polymerization reactions were performed in 20 mL flame-dried ampoules interfaced to the dual-manifold Schlenk line. As an example (Table 1, entry 1), MH (0.0111 g, 0.0824 mmol), catalyst 1 (0.4 mL, 0.0412 mol L−1) and 1.7 mL toluene were transferred into the previously flamed and argon-purged ampoules. The reaction proceeded at 30 °C for 1 h after addition of CL (0.3762 g, 3.296 mmol). After predetermined time, a portion of the polymerization mixtures, quenched by addition of a small amount of benzoic acid/CHCl3 (10 mg mL−1), was taken out for the detection of monomer conversion by 1H NMR measurement. An excess of 1 mol L−1 HCl solution was added to neutralize the catalyst, and the polymer was precipitated by cold methanol and then was filtered and dried under high vacuum to a constant weight.
Table 1 Results of MH-initiated chemoselective ROP of CL catalyzed by varied rare earth phenolatesa
Entry |
Catalyst |
[CL]/[MH]/[Cat] |
Temperature °C |
Time h |
Thiol fidelityb % |
Mn,NMRb g mol−1 |
ĐMc |
All polymerization reactions ([CL] = 1.7 mol L−1) were conducted in toluene to achieve over 99% conversion. Determined by the integration comparison according to a previous report.15,18 Determined by SEC. |
1 |
1 |
40 : 1 : 0.2 |
30 |
1 |
75 |
3550 |
1.05 |
2 |
2 |
40 : 1 : 0.2 |
30 |
1 |
83 |
5090 |
1.10 |
3 |
3 |
40 : 1 : 0.2 |
30 |
1 |
85 |
3900 |
1.06 |
4 |
4 |
40 : 1 : 0.2 |
30 |
1 |
79 |
3860 |
1.06 |
5 |
5 |
40 : 1 : 0.2 |
30 |
1 |
77 |
4470 |
1.08 |
6 |
6 |
40 : 1 : 0.2 |
30 |
1 |
88 |
4900 |
1.11 |
7 |
7 |
40 : 1 : 0.2 |
30 |
1 |
86 |
5160 |
1.24 |
8 |
8 |
40 : 1 : 0.2 |
30 |
1 |
91 |
6870 |
1.27 |
9 |
9 |
40 : 1 : 0.2 |
60 |
1 |
75 |
5600 |
1.25 |
10 |
10 |
40 : 1 : 0.2 |
30 |
1 |
84 |
7180 |
1.22 |
11 |
11 |
40 : 1 : 0.2 |
30 |
1 |
77 |
5100 |
1.23 |
12 |
12 |
40 : 1 : 0.2 |
60 |
1 |
81 |
5740 |
1.29 |
13 |
13 |
40 : 1 : 0.2 |
60 |
1 |
84 |
5800 |
1.32 |
14 |
14 |
40 : 1 : 0.2 |
60 |
1 |
76 |
5540 |
1.26 |
Table 2 Direct synthesis of thiol-terminated poly(ε-caprolactone) with a wide range of molecular weightsa
Entry |
[CL]/[MH]/[8] |
Temperature °C |
Time h |
Thiol fidelityb % |
Mn,theoc g mol−1 |
Mn,NMRb g mol−1 |
ĐMd |
All polymerization reactions ([CL] = 1.7 mol L−1) were conducted in toluene to achieve over 99% conversion. Determined by comparing the integration according to a previous report.15,18 Calculated by a combination of conversion and feed ratio. Determined by SEC. |
1 |
40 : 1 : 0.2 |
30 |
1 |
91 |
4700 |
6870 |
1.27 |
2 |
80 : 1 : 0.2 |
30 |
1 |
76 |
9270 |
10 410 |
1.34 |
3 |
100 : 1 : 0.2 |
30 |
1.5 |
72 |
11 550 |
12 120 |
1.35 |
4 |
150 : 1 : 0.2 |
60 |
1 |
73 |
17 260 |
15 540 |
1.36 |
5 |
200 : 1 : 0.2 |
60 |
2 |
75 |
22 960 |
20 450 |
1.37 |
Characterizations
Nuclear magnetic resonance (NMR) spectra were recorded on the Bruker-400 spectrometer in CDCl3 with tetramethylsilane (TMS) as the internal reference. Molecular weight distributions of obtained polymers were determined by size exclusion chromatography (SEC). The SEC was performed at 35 °C with tetrahydrofuran (THF) as the eluent at a flow rate of 0.70 mL min−1, using the SSI 1500 pump equipped with the Wyatt Optilab rEX differential refractive index (DRI) detector with a 658 nm light source, Wyatt DAWN HELEOS-II multiangle laser light scattering (MALLS) detector (laser at λ = 658 nm) and Waters Styragel HR4E THF (5 μm, 300 mm × 7.8 mm) column. Matrix-assisted laser desorption/ionization time-of-flight mass spectra (MALDI-TOF MS) were obtained using a mass spectrometer (Ultraflextreme; Bruker Co.) with the Smartbeam/Smartbeam II modified Nd:YGA laser. Mass spectra of five hundred shots were accumulated for the spectra at a 25 kV acceleration voltage. The polymer sample was dissolved in CHCl3 at a concentration of 10.0 mg mL−1, while the matrix 2,5-dihydroxybenzoic acid (2,5-DHB) was dissolved in a solution of trifluoroacetic acid and acetonitrile with a volume ratio of 70
:
30 in 10 μL of water (1%). Samples for MALDI-TOF MS were prepared by mixing the matrix and polymer solutions with a volume ratio of 1
:
1. The MALDI target was spotted with 1.0 μL of solution and allowed to air-dry.
Results and discussion
Rare earth phenolates have been demonstrated to be the chemoselective catalysts for mercapto alcohol-initiated ring-opening polymerization reactions to thiol-functionalized poly(ε-caprolactone) (PCL).15,16 To obtain deep insights into this chemoselective polymerization system, polymerization kinetics was carefully measured using easily available lanthanum (La) tris(2,6-di-tert-butyl-4-methylphenolate)s (8) and 6-mercapto-1-hexanol (MH) as the model catalyst and initiator. Fig. 1 displays the dependence of monomer conversion, thiol fidelity, degree of polymerization (DPNMR) and molecular weight distribution (ĐM) on the reaction time. The conversion of CL reached 99% in a remarkably short time of 3.5 min under mild conditions ([CL]/[MH]/[La] = 40/1/0.2, [CL] = 1.0 mol L−1, 30 °C). It was interesting that DPNMR (21) at full monomer conversion was much lower than the theoretical value (40). The fraction of thiol-terminated PCL in the product (thiol fidelity) decreased slightly with the increasing consumption of monomers, which could be generally calculated by integration comparison in 1H NMR according to a previous report.15,17,18 About 35% thiol group (Hy/(Hy + Hw) in Fig. 2) took part in the initiation of monomers, resulting in dihydroxyl-terminated PCL. After depletion of monomers, thiol fidelity, DPNMR and ĐM continuously increased with the reaction time.
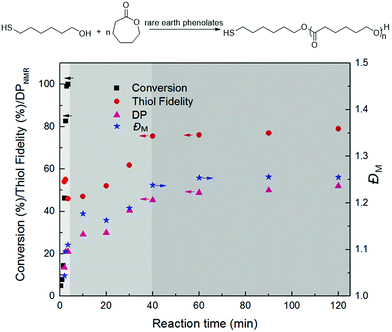 |
| Fig. 1 Dependence of monomer conversion, thiol fidelity, degree of polymerization (DPNMR) and molecular weight distribution (ĐM) and on the reaction time ([CL]/[MH]/[8] = 40/1/0.2, [CL] = 1.0 mol L−1, 30 °C). | |
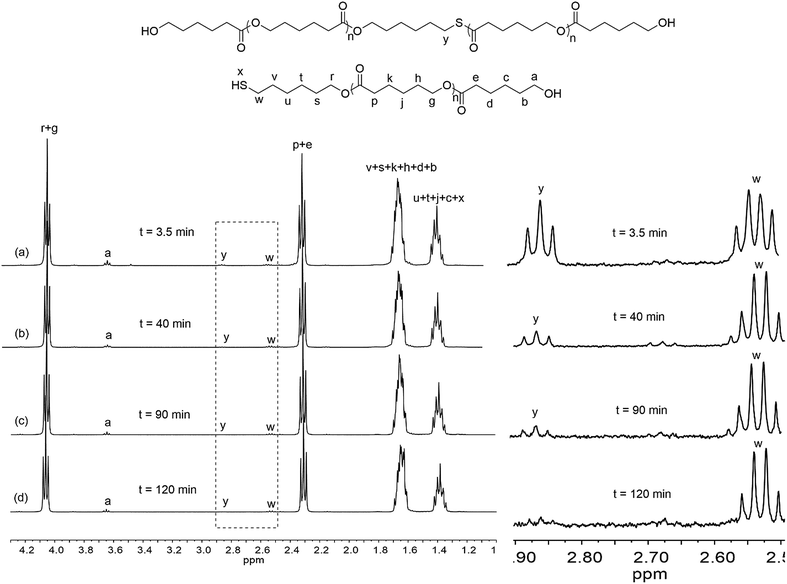 |
| Fig. 2 1H NMR of products at different reaction times ([CL]/[MH]/[8] = 40/1/0.2, [CL] = 1.0 mol L−1, 30 °C), reaction time: (a) 3.5 min; (b) 40 min; (c) 90 min and (d) 120 min. | |
Based on kinetics study, the proposed polymerization mechanism is presented in Scheme 1. The chemoselective ligand exchange between rare earth phenolates and hydroxyl groups in MH played a dominant role in the formation of O–La bond (i), which acted as an active site, followed by monomer “coordination–insertion” (iii) to designable thiol-terminated PCL. Moreover, a small amount of thiol group competed with the hydroxyl group to form S–La bond (ii), resulting in the dihydroxyl-terminated PCL containing thiolester (viii). The “extra” hydroxyl resulted in reduced thiol fidelity, and DPNMR. Proton signals (Hy) at 2.86 ppm confirmed the presence of thiolester structure (Fig. 2a). The decreased intensity of Hy with reaction time, shown in Fig. 2b–d, was attributed to the transesterification attacked by O–La bond, thus releasing the thiol group again (ix). Moreover, transesterifications (ix and xi) were responsible for the increase in molecular weight and distribution.28 This could be proved by SEC traces, which shifted toward regions of higher molecular weight and became broader with the increasing reaction time (Fig. 3).
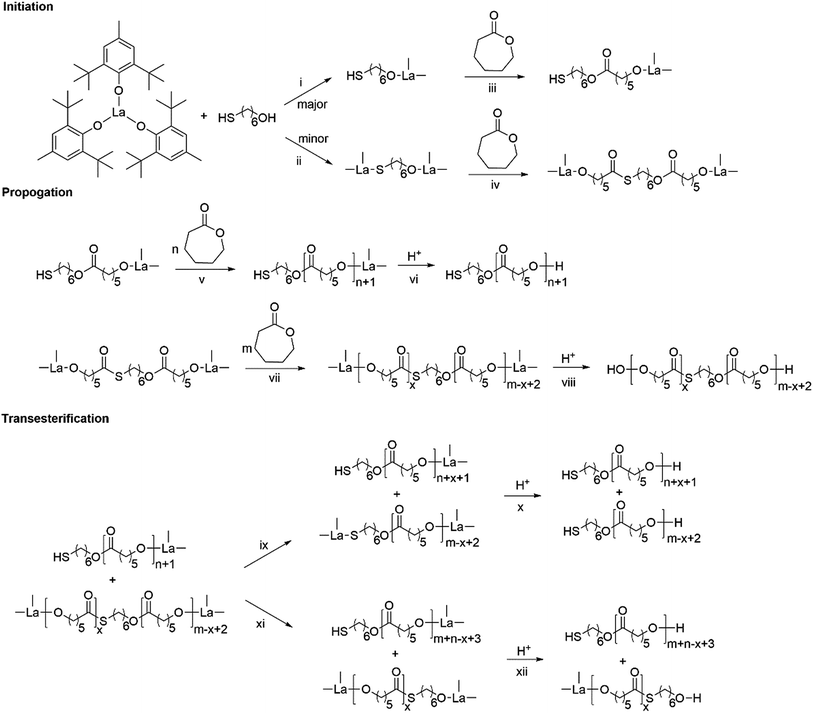 |
| Scheme 1 Proposed mechanism for MH initiated chemoselective ROP of CL catalyzed by [8]. | |
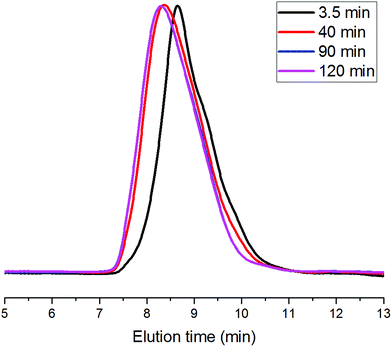 |
| Fig. 3 SEC traces of products at different reaction times ([CL]/[MH]/[8] = 40/1/0.2, [CL] = 1.0 mol L−1, 30 °C). | |
To explore the structure–activity relationship, 1–14 rare earth phenolates were prepared through varied substituted phenol ligands and rare earth metals (Scheme 2). The monomer concentration was elevated to 1.7 mol L−1 to reduce the reaction time. The results are summarized in Table 1. In general, all rare earth phenolates (1–14) showed good activity in the unprotected MH-initiated ROP system. The resultant polymers exhibited thiol fidelity over 75% (up to 91%) with narrow molecular weight distributions (ĐM < 1.4). 2,6-Di-tert-butyl-4-methylphenol and the lanthanide showed relative higher chemoselectivity, which might have resulted from substituent's electronic/steric effect and metal characteristic.29,30
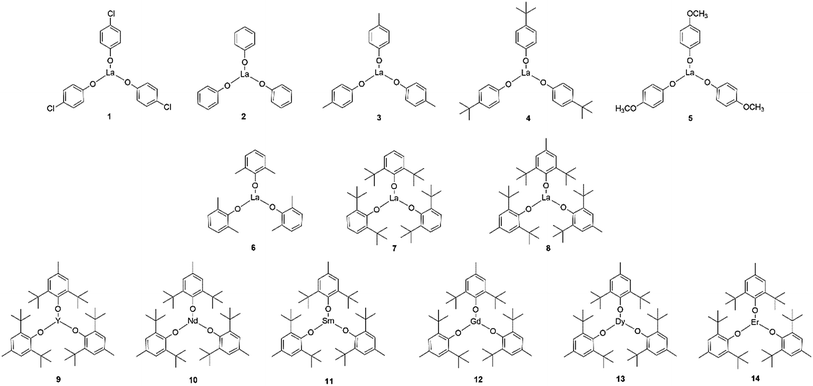 |
| Scheme 2 Structures of rare earth phenolates. | |
To demonstrate the versatility of rare earth catalysts, polymerization reactions with different monomer feed ratios were carried out to obtain varied molecular weights of PCLSH using 8 as the catalyst. On increasing [CL]/[MH] from 40 to 200, a wide range of molecular weights from 6870 to 20
450 g mol−1 were achieved within 2 h, which were in good accordance with the Mn,theo values. Fig. 5 shows the monomodal SEC traces of the resulting PCLSH with controlled molecular weight distributions (ĐM < 1.4). The chemical structure of the resultant PCLSH was characterized by 1H NMR (Fig. S1†), 1H–1H COSY (Fig. S2†) and 13C NMR (Fig. S3†). All signals were well assigned and the presence of thiol group as the end group was validated, which was consistent with a previous report.15,17,18 MALDI-TOF MS was used to provide more information about the polymer structure. In Fig. 4, two series of main peaks cationized by Na+ and K+ fairly agreed with the theoretical values of PCLSH. The interval of 114 represented the molecular weight of the CL unit. No disulfide byproducts were detected. Next, the monomer scope should be expanded to prepare varied thiol-terminated polyesters. The applications of the resultant polymers with thiol functionality would also be explored in the future.
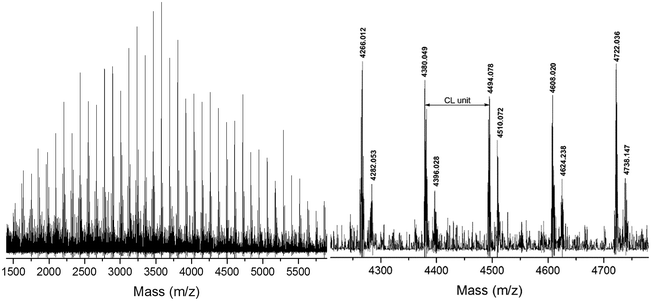 |
| Fig. 4 MALDI-TOF MS of thiol-terminated poly(ε-caprolactone) ([CL]/[MH]/[8] = 40/1/0.2, [CL] = 1.7 mol L−1, 1 h, 30 °C). | |
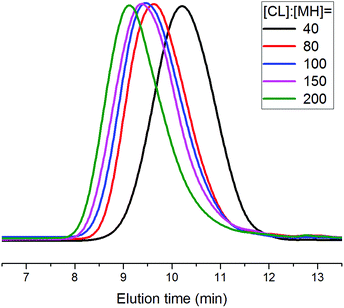 |
| Fig. 5 SEC traces of the obtained thiol-terminated poly(ε-caprolactone) with various [CL]/[MH] feed ratios (Table 2). | |
Conclusions
In this study, unprotected 6-mercapto-1-hexanol-initiated ring-opening polymerization kinetics was carefully investigated in the presence of rare earth phenolates used as catalysts. Thiol fidelity decreased as the monomers were consumed and subsequently increased, accompanied by a continuous increase in the degree of polymerization and molecular weight distribution. The proposed polymerization mechanism is presented, indicating that besides chemoselective ring-opening polymerization to design products, thiolester intermediates were generated via initiation of S–La bond and disappeared through transesterification attacked by O–La bond. The structure–activity relationship study indicated that all 14 rare earth phenolates exhibited good activities in control of thiol fidelities, molecular weights and distributions. Furthermore, 2,6-di-tert-butyl-4-methylphenol and lanthanide were found to be the relative best ligand and metal. Moreover, well-defined high-molecular-weight thiol-terminated poly(ε-caprolactone) with high thiol fidelities and narrow distributions were successfully prepared under optimized mild conditions. Further research might be carried out on monomer scope expansion and post modification of thiol groups. We believe that this article provides deep insights into chemoselective polymerization and rare earth phenolate catalysis.
Acknowledgements
This study was supported by the National Natural Science Foundation of China (21504039) and the Jiangsu National Synergetic Innovation Centre for Advance Materials (SICAM).
Notes and references
- N. G. Moon, R. J. Mondschein and T. E. Long, Polym. Chem., 2017, 8, 2598–2608 RSC.
- G. Wang, M. Jiang, Q. Zhang, R. Wang and G. Zhou, RSC Adv., 2017, 7, 13798–13807 RSC.
- T. Ware, A. R. Jennings, Z. S. Bassampour, D. Simon, D. Y. Son and W. Voit, RSC Adv., 2014, 4, 39991 RSC.
- S. De and A. Khan, Chem. Commun., 2012, 48, 3130–3132 RSC.
- S. Binder, I. Gadwal, A. Bielmann and A. Khan, J. Polym. Sci., Part A: Polym. Chem., 2014, 52, 2040–2046 CrossRef CAS.
- I. Gadwal, J. Rao, J. Baettig and A. Khan, Macromolecules, 2014, 47, 35–40 CrossRef CAS.
- I. Gadwal, M. C. Stuparu and A. Khan, Polym. Chem., 2015, 6, 1393–1404 RSC.
- M. Tohyumu, A. Hiruo and S. Nukuhumu, Macromol. Chem. Phys., 1996, 197, 3135–3148 CrossRef.
- M. Trollsås, C. J. Hawker and J. L. Hedrick, Macromolecules, 1998, 31, 5960–5963 CrossRef.
- G. Carrot, J. G. Hilborn, M. Trollsås and J. L. Hedrick, Macromolecules, 1999, 32, 5264–5269 CrossRef CAS.
- G. Carrot, J. Hilborn, J. L. Hedrick and M. Trollsås, Macromolecules, 1999, 32, 5171–5173 CrossRef CAS.
- N. C. Kalarickal, S. Rimmer, P. Sarker and J.-C. Leroux, Macromolecules, 2007, 40, 1874–1880 CrossRef CAS.
- C. Hedfors, E. Östmark, E. MalmstrÖm, K. Hult and M. Martinelle, Macromolecules, 2005, 38, 647–649 CrossRef CAS.
- N. Xu, R. Wang and Z. L. F. Du, Chem. J. Chin. Univ., 2008, 28, 1791–1795 Search PubMed.
- N. Zhu, J. Ling, Y. Zhu, W. Sun and Z. Shen, J. Polym. Sci., Part A: Polym. Chem., 2010, 48, 4366–4369 CrossRef CAS.
- N. Zhu, W. Feng, Z. Zhang, Z. Fang, Z. Li and K. Guo, Polymer, 2015, 80, 88–94 CrossRef CAS.
- N. Zhu, Z. Zhang, W. He, X. Geng, Z. Fang, X. Li, Z. Li and K. Guo, Chin. Chem. Lett., 2015, 26, 361–364 CrossRef CAS.
- N. Zhu, Y. Liu, W. Feng, W. Huang, Z. Zhang, X. Hu, Z. Fang, Z. Li and K. Guo, Eur. Polym. J., 2016, 80, 234–239 CrossRef CAS.
- C. Yu, L. Zhang and Z. Shen, Eur. Polym. J., 2003, 39, 2035–2039 CrossRef CAS.
- J. Ling, W. Chen and Z. Shen, J. Polym. Sci., Part A: Polym. Chem., 2005, 43, 1787–1796 CrossRef CAS.
- J. Ling, Z. Shen and Q. Huang, Macromolecules, 2001, 34, 7613–7616 CrossRef CAS.
- W. M. Stevels, M. J. K. Ankoné, P. J. Dijkstra and J. Feijen, Macromolecules, 1996, 29, 3332–3333 CrossRef CAS.
- J. Liu, J. Ling, X. Li and Z. Shen, J. Mol. Catal. A: Chem., 2009, 300, 59–64 CrossRef CAS.
- Y. D. J. Ling, Y. Zhu, W. Sun and Z. Shen, J. Polym. Sci., Part A: Polym. Chem., 2010, 48, 3807–3815 CrossRef.
- J. Ling, J. Liu, Z. Shen and T. E. Hogen-Esch, J. Polym. Sci., Part A: Polym. Chem., 2011, 49, 2081–2089 CrossRef CAS.
- X. Li, Y. Zhu, J. Ling and Z. Shen, Macromol. Rapid Commun., 2012, 33, 1008–1013 CrossRef CAS PubMed.
- J. Lin, W. Chen, Z. Shen and J. Ling, Macromolecules, 2013, 46, 7769–7776 CrossRef CAS.
- X. L. Li, J. Liu, J. Z. Tian, J. C. Sun and Z. Shen, Chem. J. Chin. Univ., 2010, 31, 2293–2297 CAS.
- F. Peng, J. Ling, Z. Shen and W. Zhu, J. Mol. Catal. A: Chem., 2005, 230, 135–141 CrossRef CAS.
- F. Peng and Z. Shen, J. Appl. Polym. Sci., 2007, 106, 1828–1835 CrossRef CAS.
Footnote |
† Electronic supplementary information (ESI) available: Details of 1H NMR, 13C NMR and 1H–1H COSY of products. See DOI: 10.1039/c7ra06781j |
|
This journal is © The Royal Society of Chemistry 2017 |
Click here to see how this site uses Cookies. View our privacy policy here.