DOI:
10.1039/C7RA06425J
(Paper)
RSC Adv., 2017,
7, 36242-36245
Palladium-catalyzed synthesis of quinolines from allyl alcohols and anilines†
Received
8th June 2017
, Accepted 15th July 2017
First published on 21st July 2017
Abstract
A process for quinoline synthesis through palladium-catalyzed oxidative cyclization of aryl allyl alcohols and anilines is described. This process works in the absence of acid, base and any other additive and has a broad substrate scope, tolerating electron-withdrawing groups such as nitryl, trifluoromethyl and so on. A series of quinolines are prepared in satisfactory yields.
Introduction
The quinoline skeleton occurs extensively as an integral part of many natural products, especially in alkaloids, and synthetic pharmacologically active substances due to their biological activities, such as anticancer, antiviral, antibacterial, antifungal, anti-inflammatory and antiplatelet aggregation.1 For example, Camptothecin (A) is a cytotoxic quinoline alkaloid clinically used to treat cancer by inhibiting DNA enzyme topoisomerase I; Quinoline compound (B) has been developed as a corticotropin releasing factor-1 receptor antagonists for the treatment of depression or other stress-related disorders;2 2-phenylquinolinyl moiety is deemed as the critical pharmacophore of Linsitinib (C), a clinical dual inhibitor of insulin-like growth factor-1 receptor (IGF-1R) and insulin receptor (IR) for various cancers treatment (Fig. 1).3
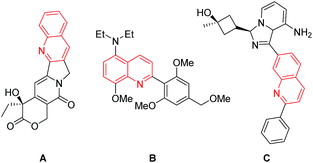 |
| Fig. 1 Examples of bioactive quinolines. | |
In view of the importance of quinolines, great endeavors have been made to develop the procedures for their synthesis over the past few decades.4 Traditionally, the classical strategies, such as Doebner–von Miller reaction, Skraup, reaction, Gould–Jacobs reaction, Combes synthesis, and Friedländer synthesis, employ the condensation reaction of carbonyl substrates and aniline derivatives under harsh conditions, namely strong acidic or strong basic conditions.5 Recently, significant attention has been paid to the development of transition metal-catalyzed protocols for the synthesis of quinoline molecules owing to their advantage of voiding the use of strong acids or strong bases in contrast with traditional methods.6 Among them, the condensation of anilines with alcohols has also been developed.7 For example, Trillo and Pastor reported that quinolines could be prepared from allyl alcohols and anilines under the catalysis of iron(III)-based Lewis acidic ionic liquid,8 and Jiang group disclosed that quinoline derivatives could be obtained from simple aliphatic alcohols and anilines under the Pd(OAc)2/2,4,6-Collidine/Brønsted acid catalytic system.9 More recently, Kapur and his coworkers demonstrated a ruthenium catalyzed [3 + 3] annulation of anilines with allyl alcohols to provide substituted quinolines based on a traceless directing-group strategy.10 Although these elegant developments could provide a range of alternatives, the development of novel and expeditious methods for the synthesis of quinoline is still in demand. Herein, we report a process for the synthesis of quinoline from allyl alcohols and anilines under the catalysis of Pd(OAc)2 without any ligand or additive (Scheme 1).
 |
| Scheme 1 Palladium-catalyzed synthesis of quinolines from allyl alcohols and anilines. | |
Results and discussion
Our studies began by optimizing the reaction conditions for the synthesis of quinolines, choosing aniline (1a) and cinnamic alcohol (2a) as model substrates. The results are collected in Table 1. When this reaction was carried out catalyzed by Pd(OAc)2 in the presence of 20 mol% TsOH in DMSO at 100 °C for 12 h, the expected quinoline 3aa was obtained in 79% GC yield (entry 1). Unexpectedly, the reaction worked well in absence of acid (entry 2). Our experimental results showed that Pd(TFA)2 and PdCl2 could not drive this reaction efficiently (entries 3–4). We then examined other palladium catalysts containing special ligands, such as Pd2(dba)3, Pd(CH3CN)2Cl2, Pd(PPh3)4Cl2, Pd(PPh3)4, and found that they could not promote the transformation preferably either (entries 5–8). The effect of solvents was next investigated. Using dioxane as the reaction medium provided the target product 3aa in 26% yield (entry 9). However, trace amount of product 3aa was detected when the reaction was performed in toluene or amide solvents such as DMF, NMP, and DMAC (entries 10–13). The results of testing reaction temperature revealed that increasing the temperature to 130 °C could improve the yield of quinoline product to 90% while higher temperature gave rise to a lower yield (entries 14–15). On the basis of the experimental results mentioned above, the optimal reaction conditions are listed in entry 14.
Table 1 Optimizing the reaction conditions for palladium-catalyzed synthesis of quinolinesa
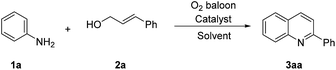
|
Entry |
Catalyst |
Solvent |
Temperature |
Yieldb (%) |
All reactions were performed with 1a (0.5 mmol), 2a (0.5 mmol), catalyst (10 mol%), in the indicated solvent (2.0 mL) under 1 atm oxygen at selected temperature for 12 h. GC yield based on 2a. 20 mol% TsOH was used. Isolated yield. |
1c |
Pd(OAc)2 |
DMSO |
100 |
79 |
2 |
Pd(OAc)2 |
DMSO |
100 |
75 |
3 |
Pd(TFA)2 |
DMSO |
100 |
16 |
4 |
PdCl2 |
DMSO |
100 |
8 |
5 |
Pd2(dba)3 |
DMSO |
100 |
Trace |
6 |
Pd(CH3CN)2Cl2 |
DMSO |
100 |
5 |
7 |
Pd(PPh3)4Cl2 |
DMSO |
100 |
Trace |
8 |
Pd(PPh3)4 |
DMSO |
100 |
Trace |
9 |
Pd(OAc)2 |
Dioxane |
100 |
26 |
10 |
Pd(OAc)2 |
Toluene |
100 |
Trace |
11 |
Pd(OAc)2 |
DMF |
100 |
Trace |
12 |
Pd(OAc)2 |
NMP |
100 |
Trace |
13 |
Pd(OAc)2 |
DMAC |
100 |
Trace |
14 |
Pd(OAc)2 |
DMSO |
130 |
90 (85)d |
15 |
Pd(OAc)2 |
DMSO |
150 |
82 |
The scope of the protocol was further evaluated after the optimal reaction conditions were established. Cinnamic alcohol 2a was reacted with s series of substituted anilines under the best reaction conditions, and the results are listed in Table 2. para-substituted anilines were firstly probed. Generally, moderate to excellent yields could be achieved from both electron-donating groups and electron-withdrawing groups substituted anilines (3ba–3ka). For example, 4-methoxyaniline and 4-(trifluoromethyl)aniline afforded the corresponding quinolines in 80% and 84% yield, respectively (3ba, 3ka). meta-Substituted anilines were then explored. When 3-methylaniline was subjected to the optimized conditions, 7-methyl substituted product 3la and 5-methyl substituted product 3la' were obtained in the ratio of 5
:
1, which suggested that steric hindrance of the substituents had significant influence on the regioselectivity of the oxidative cyclization reaction, and 3,4-disubstituted anilines furnished 6,7-disubstituted quinoline products selectively (3ma–3oa). ortho-Substituted anilines were finally tested. As shown in Table 2, all ortho-substituted anilines went through the transformation smoothly, producing the desired quinoline compounds in good yields (3pa–3sa). Moreover, beta-naphthylamine was also found to be a good partner of cinnamic alcohol under the standard conditions and gave 2-phenylbenzo[g]quinoline in 76% yield (3ta).
Table 2 Scope of anilines for Pd(OAc)2-catalyzed synthesis of quinolinesa,b

|
Reactions were performed with 1a (0.5 mmol), 2 (0.5 mmol), Pd(OAc)2 (10 mol%), in DMSO (2 mL) at 130 °C for 12 h. Isolated yield. |
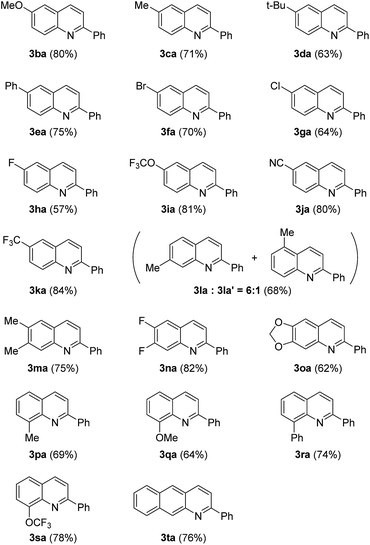 |
A variety of allyl alcohols were treated with aniline 1a. And the results are summarized in Table 3. We found that cinnamic alcohols, whose phenyl rings were substituted by electron-donating groups, reacted smoothly with aniline to provide target quinolines in moderate to good yields (3ab–3ae). Likewise, moderate to good yields could be achieved from cinnamic alcohols whose phenyl rings were substituted by F and NO2 (3ag–3ah). It is noteworthy that this procedure for the preparation of quinoline derivatives tolerates aryl bromide which allows further functionalization (3ah). To our delight, 2,3-disubstituted quinoline could be obtained from α-methyl cinnamic alcohol (3ai). Heteroaryl allyl alcohol was finally assessed and 2-furyl quinoline was successfully isolated in a yield of 65% (3aj).
Table 3 Scope of allyl alcohols for Pd(OAc)2-catalyzed synthesis of quinolinesa,b

|
Reactions were performed with 1 (0.5 mmol), 2a (0.5 mmol), Pd(OAc)2 (10 mol%), in DMSO (2 mL) at 130 °C for 12 h. Isolated yield. |
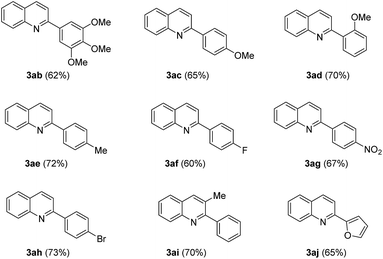 |
In order to gain some insight into the mechanism of the oxidative annulation reaction, some control experiments were carried out. When cinnamaldehyde reacted with aniline 1a under the optimal reaction conditions, quinoline 3aa was detected by in 91% yield (eqn (1)). When N-cinnamylideneaniline was subjected to the standard reaction conditions, product 3aa was given in 93% GC yield (eqn (2)), which revealed that this transformation occurred via aldimine as the key intermediate (Scheme 2).
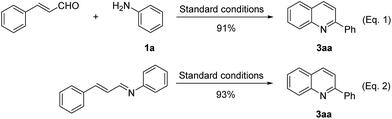 |
| Scheme 2 Control experiments. | |
On the basis of the above mentioned results and relevant reports in the literature,11,6a a reasonable reaction pathway for palladium-catalyzed quinoline synthesis from allyl alcohols with anilines is illustrated in Scheme 3. Firstly, cinnamic alcohol 2a is oxidized by dioxygen under the catalysis of palladium to produce α,β-unsaturated aldehyde A, which goes through a condensation with aniline to give imine B. Dimerization then takes place to generate diazetidine intermediates C with the help of palladium. Its C–N bond cleaves and the following irreversible cyclization forms intermediates E, which isomerizes readily into F driven by the charge, followed by an intramolecular nucleophilic attack along with the elimination of 1 mol of imine, to provide 2-phenyl-1,2-dihydroquinolin G. Palladium-catalyzed dehydro-aromatization subsequently occurs to yield the final product.
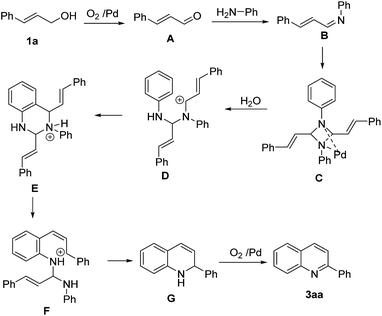 |
| Scheme 3 Possible reaction pathway for palladium-catalyzed synthesis of quinolines from allyl alcohols and anilines. | |
Experimental
General information
All reagents were of analytical grade and obtained from commercial suppliers and used without further purification. 1H and 13C NMR spectra were recorded on a Bruker DRX-400 spectrometer; CDCl3 was used as solvent and TMS as an internal standard.
Typical procedure for palladium-catalyzed synthesis of quinolines from allyl alcohols and anilines
Allyl alcohol (0.5 mmol), aniline (0.5 mmol), Pd(OAc)2 (11.2 mg, 10 mol%) and DMSO (2 mL) were added into a test tube attached to an oxygen balloon (1 atm). The system was stirred magnetically and heated at 130 °C with an oil bath for 12 h. And then the reaction was quenched by the addition of 10 mL water. The aqueous solution was extracted with ethyl acetate (3 × 10 mL) and the combined extract was dried with anhydrous MgSO4. The solvent was vacuumed and the crude product was isolated by TLC (solvent: hexane/ethyl acetate) to give the pure quinoline product.
Conclusions
In conclusion, we have developed a process for quinoline synthesis through palladium-catalyzed oxidative cyclization of aryl allyl alcohols and anilines. This methodology would offer an alternative approach to biologically important quinoline derivatives from commercially available starting materials. More importantly, this process works without acids, bases or any other additives.
Acknowledgements
The authors thank the National Natural Science Foundation of China (21572040) and Guangdong Natural Science Foundation (2015A030310371) for financial support.
Notes and references
-
(a) M. A. Syed, Expert Opin. Ther. Pat., 2016, 26, 1201–12219 CrossRef PubMed;
(b) P. Y. Chung, Z. X. Bian, H. Y. Pun, D. Chan, A. S. C. Chan, C. H. Chui, J. C. O. Tang and K. H. Lam, Future Med. Chem., 2015, 7, 947–967 CrossRef CAS PubMed.
- K. Takeda, T. Terauchi, M. Hashizume, K. Shikata, R. Taguchi, K. Murata-Tai, M. Fujisawa, Y. Takahashi, K. Shin, M. Ino, H. Shibata and M. Yonaga, Bioorg. Med. Chem., 2012, 20, 6559–6578 CrossRef CAS PubMed.
-
(a) M. J. Mulvihill, A. Cooke, M. Rosenfeld-Franklin, E. Buck, K. Foreman, D. Landfair, M. O'Connor, C. Pirritt, Y. Sun, Y. Yao, L. D. Arnold, N. W. Gibson and Q.-S. Ji, Future Med. Chem., 2009, 1, 1153–1171 CrossRef CAS PubMed;
(b) M. J. Mulvihill, Q.-S. Ji, H. R. Coate, A. Cooke, H. Dong, L. Feng, K. Foreman, M. Rosenfeld-Franklin, A. Honda, G. Mak, K. M. Mulvihill, A. I. Nigro, M. O'Connor, C. Pirrit, A. G. Steinig, K. Siu, K. M. Stolz, Y. Sun, P. A. R. Tavares, Y. Yao and N. W. Gibson, Bioorg. Med. Chem., 2008, 16, 1359–1375 CrossRef CAS PubMed.
-
(a) J. B. Bharate, R. A. Vishwakarma and S. B. Bharate, RSC Adv., 2015, 5, 42020–42053 RSC;
(b) R. K. Saunthwal, M. Patel and A. K. Verma, J. Org. Chem., 2016, 81, 6563–6572 CrossRef CAS PubMed;
(c) S. J. Gharpure, S. K. Nanda, P. A. Adate and Y. G. Shelke, J. Org. Chem., 2017, 82, 2067–2080 CrossRef CAS PubMed;
(d) H. Wang, Q. Xu, S. Shen and S. Yu, J. Org. Chem., 2017, 82, 770–775 CrossRef CAS PubMed;
(e) X. Wu, X. Geng, P. Zhao, J. Zhang, X. Gong, Y. Wu and A. Wu, Org. Lett., 2017, 19, 1550–1553 CrossRef CAS PubMed;
(f) R. K. Saunthwal, M. Patel and A. K. Verma, Org. Lett., 2016, 18, 2200–2203 CrossRef CAS PubMed;
(g) R. Wang, H. Fan, W. Zhao and F. Li, Org. Lett., 2016, 18, 3558–3561 CrossRef CAS PubMed.
-
(a) S. M. Prajapati, K. D. Patel, R. H. Vekariya, S. N. Panchal and H. D. Patel, RSC Adv., 2014, 4, 24463–24476 RSC;
(b) G. A. Ramann and B. J. Cowen, Tetrahedron Lett., 2015, 56, 6436–6439 CrossRef CAS.
-
(a) C. Li, J. Li, Y. An, J. Peng, W. Wu and H. Jiang, J. Org. Chem., 2016, 81, 12189–12196 CrossRef CAS PubMed;
(b) M. Zhong, S. Sun, J. Cheng and Y. Shao, J. Org. Chem., 2016, 81, 10825–10831 CrossRef CAS PubMed;
(c) J. Zheng, Z. Li, L. Huang, W. Wu, J. Li and H. Jiang, Org. Lett., 2016, 18, 3514–3517 CrossRef CAS PubMed;
(d) R. Zhu, G. Cheng, C. Jia, L. Xue and X. Cui, J. Org. Chem., 2016, 81, 7539–7544 CrossRef CAS PubMed;
(e) X. Yang, L. Li, Y. Li and Y. Zhang, J. Org. Chem., 2016, 81, 12433–12442 CrossRef CAS PubMed;
(f) M. Mastalir, M. Glatz, E. Pittenauer, G. Allmaier and K. Kirchner, J. Am. Chem. Soc., 2016, 138, 15543–15546 CrossRef CAS PubMed;
(g) M. Zhong, S. Sun, J. Cheng and Y. Shao, J. Org. Chem., 2016, 81, 10825–10831 CrossRef CAS PubMed;
(h) X. Yi and C. Xi, Org. Lett., 2015, 17, 5836–5839 CrossRef CAS PubMed;
(i) R. Yan, X. Liu, C. Pan, X. Zhou, X. Li, X. Kang and G. Huang, Org. Lett., 2013, 15, 4876–4879 CrossRef CAS PubMed;
(j) M. Ni, Y. Zhang, T. Gong and B. Feng, Adv. Synth. Catal., 2017, 359, 824–831 CrossRef CAS.
-
(a) R. N. Monrad and R. Madsen, Org. Biomol. Chem., 2011, 9, 610–615 RSC;
(b) D. K. T. Yadav and B. M. Bhanage, RSC Adv., 2015, 5, 51570–51575 RSC;
(c) R. I. Khusnutdinov, A. R. Bayguzina and R. I. Aminov, Russ. J. Gen. Chem., 2016, 86, 1613–1618 CrossRef CAS;
(d) R. I. Khusnutdinov, A. R. Bayguzina, R. I. Aminov and U. M. Dzhemilev, Russ. J. Org. Chem., 2012, 48, 690–693 CrossRef CAS.
- P. Trillo and I. M. Pastor, Adv. Synth. Catal., 2016, 358, 2929–2939 CrossRef CAS.
- J. Li, J. Zhang, H. Yang and G. Jiang, J. Org. Chem., 2017, 82, 3284–3290 CrossRef CAS PubMed.
- G. S. Kumar, P. Kumar and M. Kapur, Org. Lett., 2017, 19, 2494–2497 CrossRef CAS PubMed.
-
(a) J. J. Eisch and T. Dluzniewski, J. Org. Chem., 1989, 54, 1269–1274 CrossRef CAS;
(b) Y.-C. Wu, L. Liu, H.-J. Li, D. Wang and Y.-J. Chen, J. Org. Chem., 2006, 71, 6592–6595 CrossRef CAS PubMed.
Footnote |
† Electronic supplementary information (ESI) available: NMR of all products. See DOI: 10.1039/c7ra06425j |
|
This journal is © The Royal Society of Chemistry 2017 |
Click here to see how this site uses Cookies. View our privacy policy here.