DOI:
10.1039/C7RA06310E
(Paper)
RSC Adv., 2017,
7, 36895-36901
Syntheses, crystal structures, and magnetic properties of cyclic dimer Ln2L2 complexes constructed from (3-pyridinylmethoxy)phenyl-substituted nitronyl nitroxide ligands†
Received
6th June 2017
, Accepted 10th July 2017
First published on 25th July 2017
Abstract
Herein, five lanthanide-radical cyclic dimer complexes [Ln(hfac)3(NIT-3PyPh)]2 derived from (3-pyridinylmethoxy)phenyl-substituted nitronyl nitroxide ligands, 2-(4-(3-pyridinylmethoxy)phenyl)-4,4,5,5-tetramethyl-imidazolyl-1-oxyl-3-oxide (NIT-3PyPh), and Ln(hfac)3 (LnIII = Gd (1), Tb (2), Dy (3), Ho (4), and Er (5); hfac = hexafluoroacetylacetonato), were synthesized as well as structurally and magnetically characterized. The single-crystal structures show that these complexes are isostructural, in which the NIT-3PyPh molecule acts as a bridging ligand linking two LnIII ions through the oxygen atom of the N–O group and nitrogen atom from the pyridine ring to form a four-spin system. Tb and Dy complexes exhibit a frequency-dependence of ac magnetic susceptibilities, slowing the relaxation of the magnetization.
Introduction
Research on single-molecule magnets (SMMs) has been a very active field and rapidly expanding for over two decades since the first magnetic dynamics of Mn12Ac was discovered in the early 1990s.1 These materials have potential applications in high-density data storage, quantum information processing systems, and spintronic devices.2–4 Recently, lanthanide-based systems, especially heavy lanthanide ions, have become increasingly interesting due to their large intrinsic magnetic anisotropy from the large unquenched orbital moment.5 This strategy has achieved tremendous success in the production of novel SMMs mainly comprising mononuclear and polynuclear pure 4f6,7 and mixed 3d–4f8 systems. However, the main disadvantage of these compounds is the internal character of their 4f unpaired electrons, which results in weak magnetic interactions between spin carriers.9 Nitronyl nitroxide radicals (NITs) and other organic radicals, such as verdazyl, TEMPO, TTF, and so on, as spin carriers are fascinating building blocks and bridged ligands not only for stabilization under ambient conditions but also for directed coordination to transfer effective magnetic interactions. The organic radical has proven to be an attractive route to obtain magnetically coupled 2p–4f heterospin systems.10 Contrary to 3d–4f and pure 4f metal SMMs, to date, 2p–4f SMMs are relatively rare, and only some examples including mononuclear lanthanide–nitronyl nitroxide radical complexes,11 lanthanide–nitronyl nitroxide dimers,12 and lanthanide-biradical compounds13 have been reported. As is known, discrete complexes with definite geometry resulting from paramagnetic metal ions and organic radicals are good candidates for the fundamental studies of magneto-structural correlations, in particular for determining how the structural factors affect the interaction between metals and nitronyl nitroxide and how to improve magnetic relaxation.14 Moreover, for nitronyl nitroxide–Ln complexes, several investigations have shown that the substituents of the nitronyl nitroxide radicals play a crucial role in modulating the dynamics of magnetization.15 Herein, a (3-pyridinylmethoxy)phenyl-substituted nitroxide radical, NIT-3PyPh (NIT-3PyPh = 2-(4-(3-pyridinylmethoxy)phenyl)-4,4,5,5-tetramethyl-imidazolyl-1-oxyl-3-oxide, Scheme 1), is chosen to assemble a series of 2p–4f compounds. This radical ligand not only has a functional coordinated atom but also shows a long and flexible substituted group relativity as compared to the pyridine or benzene-substituted radical, which may construct the variety structures of complexes. In this study, the complexes are isostructural, exhibiting cyclic nitronyl nitroxide–Ln dimer units in which two NIT-3PyPh radicals act as bridging ligands to link two Ln(hfac)3 units through the oxygen atoms of the nitroxide groups and nitrogen atoms of the pyridine ring, namely [Ln(hfac)3(NIT-3PyPh)]2(LnIII = Gd (1), Tb (2), Dy (3), Ho (4), and Er (5); hfac = hexafluoroacetylacetonato). Magnetic studies show that Tb and Dy complexes exhibit slow magnetic relaxation, the typical characteristic of SMMs.
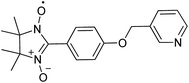 |
| Scheme 1 NIT-3PyPh ligand. | |
Experimental
Materials and physical measurements
All the reagents and chemicals used in the syntheses were of analytical grade. Ln(hfac)3·2H2O and the radical ligand NIT-3PyPh were prepared according to the literature methods.16 Elemental analyses for carbon, hydrogen, and nitrogen were carried out using a Perkin-Elmer 240 elemental analyzer. Infrared spectra were obtained from KBr pellets in the 4000–400 cm−1 region via a Bruker Tensor 27FTIR spectrophotometer. Magnetic measurements were conducted using a Quantum Design VSM SQUID magnetometer. Diamagnetic corrections were made with Pascal's constants for all of the constituent atoms and sample holders.
Synthesis
Compounds 1–5 [Ln(hfac)3(NIT-3PyPh)]2(LnIII = Gd (1), Tb (2), Dy (3), Ho (4), and Er (5)) were obtained via the same procedure. A suspension of 0.02 mmol Ln(hfac)3·2H2O in 20 mL n-heptane was heated to reflux for about 2 hours. Then, the solution was subsequently cooled to 90 °C; after this, 0.02 mmol NIT-3PyPh in 10 mL dichloromethane was added under stirring. The mixture was refluxed for another 1 hour and then cooled to room temperature. The filtrate was allowed to stay at room temperature for slow evaporation. After two days, dark-blue block crystals suitable for single-crystal X-ray analysis were obtained.
[Gd(hfac)3(NIT-3PyPh)]2 (1). Yield: 76%. Anal. calc. for C68H50F36Gd2N6O18: C, 36.50, H, 2.25, N, 3.76; found: C, 36.66, H, 2.42, N, 3.93%. IR (KBr, cm−1): 3432 (m), 1654 (s), 1510 (m), 1260 (s), 1208 (s), 1147 (s), 799 (m), 662 (m).
[Tb(hfac)3(NIT-3PyPh)]2 (2). Yield: 72%. Anal. calc. for C68H50F36Tb2N6O18: C, 36.45, H, 2.25, N, 3.75; found: C, 36.51, H, 2.32, N, 4.14%. IR (KBr, cm−1): 3433 (m), 1655 (s), 1512 (m), 1259 (s), 1205 (s), 1146 (s), 798 (m), 661 (m).
[Dy(hfac)3(NIT-3PyPh)]2 (3). Yield: 74%. Anal. calc. for C68H50F36Dy2N6O18: C, 36.33, H, 2.24, N, 3.74; found: C, 36.21, H, 2.12, N, 3.95%. IR (KBr, cm−1): 3433 (m), 1658 (s), 1505 (m), 1259 (s), 1208 (s), 1148 (s), 800 (m), 661 (m).
[Ho(hfac)3(NIT-3PyPh)]2 (4). Yield: 71%. Anal. calc. for C68H50F36Dy2N6O18: C, 36.25, H, 2.24, N, 3.73; found: C, 36.31, H, 2.29, N, 3.67%. IR (KBr, cm−1): 3432 (m), 1658 (s), 1505 (m), 1259 (s), 1208 (s), 1148 (s), 800 (m), 661 (m).
[Er(hfac)3(NIT-3PyPh)]2 (5). Yield: 79%. Anal. calc. for C68H50F36Er2N6O18: C, 36.18, H, 2.23, N, 3.72; found: C, 36.29, H, 2.45, N, 3.93%. IR (KBr, cm−1): 3432 (m), 1655 (s), 1512 (m), 1259 (s), 1205 (s), 1146 (s), 798 (m), 661 (m).
X-ray data collection and structure refinement
All crystallographic data were obtained using a Rigaku CCD diffractometer with graphite monochromated Mo Kα radiation (λ = 0.71073 Å) at 113 K. Structures were solved via direct methods using the SHELXS-2014 program and refined by a full-matrix least-squares techniques against F2 with the SHELXTL-2014 program package.17 Some restraints are applied, such as ISOR, DFIX, to solve the disorder of the F atoms. Besides fluorine atoms, all other non-hydrogen atoms were anisotropically refined, and hydrogen atoms were fixed at the calculated positions, which were refined using a riding model. The pertinent crystallographic data and structure refinement parameters for complexes 1–5 are listed in Table 1. The important bond lengths and angles for 1–5 are listed in Tables 2 and S1–S5.† CCDC 1538537–1538541.†
Table 1 Crystal data and structure refinement for complexes 1–5
|
1 Gd |
2 Tb |
3 Dy |
4 Ho |
5 Er |
Formula |
C68H50F36Ln2N6O18 |
Mr |
2237.63 |
2241.98 |
2248.12 |
2252.99 |
2257.64 |
T, K |
113(2) |
293(2) |
113(2) |
113(2) |
113(2) |
λ (Mo Kα), Å |
0.71073 |
0.71073 |
0.71073 |
0.71073 |
0.71073 |
Crystal system |
Monoclinic |
Monoclinic |
Monoclinic |
Monoclinic |
Monoclinic |
Space group |
P21/n |
P21/c |
P21/c |
P21/c |
P21/c |
a, Å |
13.433(3) |
13.504(3) |
13.394(3) |
13.372(3) |
13.387(3) |
b, Å |
17.289(4) |
17.675(3) |
17.257(3) |
17.214(3) |
17.241(3) |
c, Å |
18.334(4) |
22.376(7) |
22.104(7) |
22.022(7) |
22.063(7) |
α, deg |
90 |
90 |
90 |
90 |
90 |
β, deg |
93.07(3) |
124.48(2) |
124.21(2) |
124.08(2) |
124.00(2) |
γ, deg |
90 |
90 |
90 |
90 |
90 |
V, Å3 |
4252.0(15) |
4402.5(18) |
4225.2(18) |
4198.5(18) |
4221.7(18) |
Z |
2 |
2 |
2 |
2 |
2 |
Dc, g cm−3 |
1.748 |
1.690 |
1.767 |
1.782 |
1.776 |
μ, mm−1 |
1.692 |
1.734 |
1.902 |
2.018 |
2.121 |
θ range, deg |
1.62–25.01 |
1.60–25.01 |
1.62–25.01 |
1.63–27.97 |
1.62–25.01 |
Unique reflns/Rint |
7492/0.0463 |
7726/0.0409 |
7439/0.0491 |
10 025/0.0546 |
7426/0.0445 |
GOF |
1.05 |
1.069 |
1.038 |
1.023 |
1.070 |
R1 [I > 2σ(I)] |
0.0603 |
0.0719 |
0.0577 |
0.0642 |
0.0571 |
wR2 [I > 2σ(I)] |
0.1687 |
0.1925 |
0.1648 |
0.1791 |
0.1663 |
Table 2 The important bond distances (Å) and angles (°) for complexes 1–5
Complexes |
Bond lengths Ln–O(rad) |
Bond lengths Ln–N(py) |
Angle of O(rad)–Ln–N(py) |
Dihedral angle between the phenyl plane and the nitroxide plane |
Dihedral angle between the phenyl plane and the pyridine plane |
1 |
2.320(5) |
2.579(5) |
71.91(17) |
36.46 |
48.74 |
2 |
2.317(6) |
2.585(6) |
72.0(2) |
37.10 |
48.96 |
3 |
2.294(5) |
2.560(5) |
71.74(17) |
36.39 |
48.96 |
4 |
2.282(4) |
2.547(5) |
71.74(16) |
35.97 |
49.01 |
5 |
2.281(5) |
2.534(6) |
71.75(18) |
36.07 |
49.02 |
Results and discussion
Crystal structure
Single-crystal X-ray diffraction analyses reveal that complexes 1–5 are isomorphous; therefore, only the structure of complex 1 has been described in detail. Complex 1 crystallizes in the monoclinic space group P21/n with the central symmetric structure. Moreover, two NIT-3PyPh paramagnetic ligands are linked through coordination bonds with two molecules of Gd(hfac)3 to generate a four-spin cyclic dimer complex (Fig. 1). GdIII is coordinated with six oxygen atoms from three hfac ligands and one oxygen atom and one nitrogen atom from two different nitronyl nitroxide units to form a distorted square antiprism geometry. As is known, eight coordinate geometries refer to some polyhedra, including D2d dodecahedron (DD), D4d square antiprism (SAPR), C2v bicapped trigonal prism (TPRS), and so on. Continuous shape measures have been performed with SHAPE to evaluate the actual shape of the coordination spheres of the Ln atoms for complexes 1–518(Table 3), indicating that all of them are located in distorted square antiprism environments. The NIT-3PyPh molecule acts as a bidentate bridging ligand to link two GdIII ions by the oxygen atom of the nitroxide group and the nitrogen atom of the pyridine ring. Within a dimer unit, the phenyl ring of one NIT-3PyPh ligand is almost parallel to the pyridine group of the other NIT-3PyPh molecule; this may due to the π–π stacking interaction between the phenyl ring and the pyridine ring. The bond lengths of GdIII–O (nitroxide) and GdIII–N are 2.320(5) and 2.579(5) Å, respectively, which are comparable to those reported for other lanthanide–pyridine-substituted radical complexes.12a,b In the dimer units, the distance of two metal ions is 11.675 Å. The shortest intermolecular GdIII–GdIII distance is 9.272 Å, whereas the nearest contact between the uncoordinated N–O groups is 3.546 Å (Fig. 2), which implies that there is non-negligible magnetic coupling between uncoordinated NO groups. The crystal structures and packing arrangements for the complexes 2–5 are shown in Fig. S1–S4 and S5–S8,† respectively.
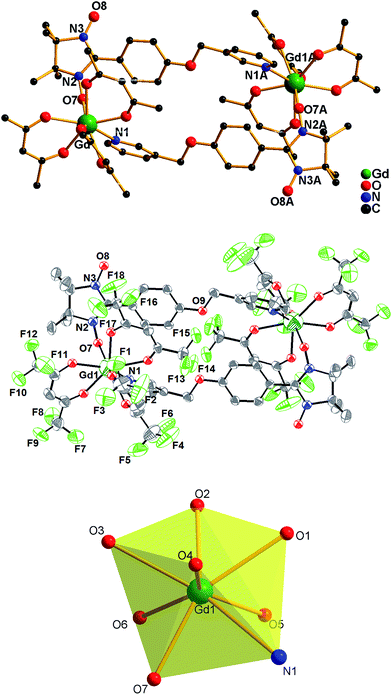 |
| Fig. 1 (Top) Crystal structure of complex 1, (H atoms and F atoms are omitted for clarity and symmetry transformations used to generate equivalent atoms A: 2 − x, 2 − y, −z); (middle) ORTEP view of complex 1 at the 50% probability level. (Bottom) The coordination polyhedron of Gd(III) ion in 1. | |
Table 3 Lanthanide geometry analysis using the Shape software for complexes 1–5
Complex |
Ln |
D4d SAPR |
D2d DD |
C2v TPRS |
1 |
Gd |
0.789 |
1.877 |
1.833 |
2 |
Tb |
0.792 |
2.025 |
1.798 |
3 |
Dy |
0.777 |
1.901 |
1.808 |
4 |
Ho |
0.750 |
1.908 |
1.782 |
5 |
Er |
0.739 |
1.907 |
1.764 |
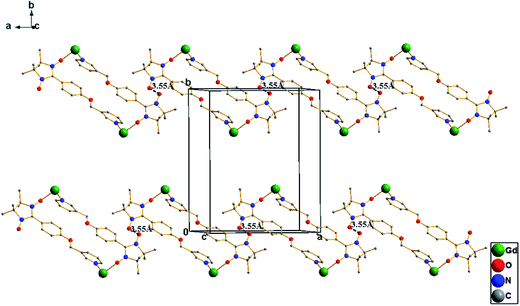 |
| Fig. 2 Packing diagram of complex 1, hfac ligands and hydrogen and fluorine atoms are not shown for clarity. | |
Magnetic properties
The temperature-dependent magnetic susceptibility data of complexes 1–5 were measured in the range of 2–300 K under an external magnetic field of 1 kOe (Fig. 3). At room temperature, the χMT values are 16.84, 24.99, 29.02, 29.68, and 25.04 cm3 K mol−1 for compounds 1, 2, 3, 4, and 5, respectively, which are close to the expected values of 16.51, 24.39, 29.09, 28.89, and 23.71 cm3 K mol−1 for the uncoupled two LnIII ions (GdIII: 8S7/2, S = 7/2, L = 0, J = 7/2, g = 2, C = 7.88 cm3 K mol−1; TbIII: 7F6, S = 3, L = 3, J = 6, g = 3/2, C = 11.82 cm3 K mol−1; DyIII: 6H15/2, S = 5/2, L = 5, J = 15/2, g = 4/3, C = 14.17 cm3 K mol−1; HoIII: 5I8, S = 2, L = 6, J = 8, g = 5/4, C = 14.08 cm3 K mol−1; and ErIII: 4H15/2, S = 3/2, L = 6, J = 15/2, g = 6/5, C = 11.48 cm3 K mol−1) plus two organic radicals NIT-3PyPh (S = 1/2). For the complex of 1, upon cooling, the χMT value remains almost constant until 74 K and then decreases more and more dramatically to reach a value of 13.62 cm3 K mol−1 at 2 K. As is known, there are mainly three kinds of magnetic interactions based on the present magnetic system: (i) GdIII ion interacting with the directly coordinated NO group (J1); (ii) the magnetic coupling between two uncoordinated NO groups through space (J2); and (iii) GdIII ion interacting with the NO group through the pyridine and benzene ring. However, the last interaction should be weak19 and could be neglected. Hence, the magnetic behavior of 1 can be interpreted as that of one linear Gd–rad–rad–Gd magnetic unit (Scheme 2). According to the structural data, the torsion angle for Gd–O–N–C is 77.5°, and the value of J1 should be positive.20 Therefore, the observed antiferromagnetic coupling in complex 1 may be due to the strong magnetic coupling between two uncoordinated NO groups, which overwhelm the ferromagnetic interaction between Gd and the directed coordinated NO group.21 MAGPACK22 software was used to simulate the magnetic susceptibilities based on the Hamiltonian equation: Ĥ = −2J1(ŜRad1ŜGd1 + ŜRad2ŜGd2)−2J2ŜRad1ŜRad2 and the best agreement between calculated and experimental behaviors was obtained for J1 = 1.89 cm−1, J2 = −18.39 cm−1, and g = 2.01. The positive J1 is in agreement with a weak ferromagnetic interaction between Gd(III) and the NO group, as was already observed in other gadolinium nitronyl nitroxide compounds.23 The large negative J2 indicates that there exists important antiferromagnetic interaction between uncoordinated NO groups. For complexes 2, 3, 4, and 5, the χMT values continuously decrease with the decreasing temperature and reach minimum values of 14.10, 18.66, 13.02, and 8.69 cm3 K mol−1 at 2 K, respectively. The overall behaviors of compounds 2–5 can be ascribed to the depopulation of Ln(III) ion Stark sublevels and magnetic couple interaction within the complexes.
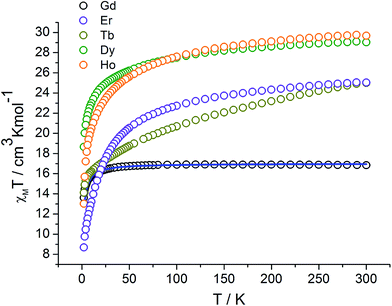 |
| Fig. 3 χMT versus T plots of complexes 1–5; the solid line represents the best-fitted simulation curve using MAGPACK. | |
 |
| Scheme 2 The magnetic exchange pathways in complex 1. | |
The field-dependent magnetizations of 1–5 at 2.0 K are shown in Fig. 4 and S9.† For complex 1, upon increasing the applied field, M increases up to 14.34 Nβ at 70 kOe. For any value of the field, the experimental magnetization value is lower than the magnetization calculated by the Brillouin function for non-coupled two S = 7/2 and two S = 1/2 spins (g = 2.0 and T = 2 K), further supporting the antiferromagnetic interactions in this compound. For complexes 2, 3, 4, and 5, the magnetization reveals a rapid increase at low field and reaches 8.53, 12.03, 11.99, and 10.43 Nβ at 70 kOe, respectively. All the M values did not reach the expected saturation values; this suggested the presence of magnetic anisotropy and/or low-lying excited states in the systems.
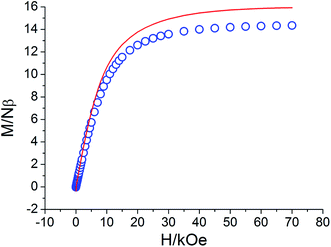 |
| Fig. 4 M versus H plot at 2 K for complex 1. The solid line represents the Brillouin function for non-coupled two S = 7/2 and two S = 1/2 spin centers (g = 2.0, T = 2 K). | |
AC magnetic susceptibility measurements were carried out for 2 and 3 to probe dynamic magnetic behaviors. For compound 2, as shown in Fig. 5, in a zero dc field with an ac field of 2 Oe, both the in-phase (χ′) and out-of phase (χ′′) components of ac susceptibility showed frequency dependence; this suggested the presence of slow magnetic relaxation at low temperatures. According to the magnetic couplings, the quantum tunneling of the magnetization (QTM) is reduced when the unit is exchange coupled with neighboring magnetic centers, which allows the observation of the magnetic relaxation behavior even without an applied field. The peaks of the out-of-phase signals are visible and follow the Arrhenius law τ = τ0
exp(Δ/kBT) with the pre exponential factor τ0 = 4.04 × 10−7 s and the effective anisotropy barrier Δ/kB = 21.6 K. Compared with those of the reported cyclic Tb-radical complexes,12 the value of this barrier is almost high, especially in the zero dc field. (Fig. S10†).
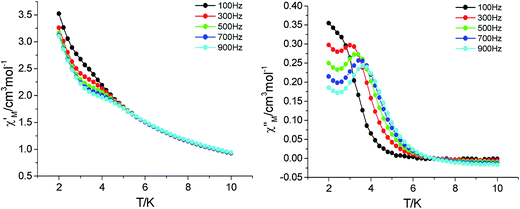 |
| Fig. 5 Temperature dependence of the in-phase (left) and out-of-phase (right) plots for 2 at frequencies 100–900 Hz with Hdc = 0 and Hac = 2 Oe. | |
Complex 3 shows frequency-dependent out-of-phase signals in a zero dc field with an ac field of 2 Oe (Fig. 6); however, there is no maxima visible down to 2.0 K. This behavior may result from the relaxation mechanism such as quantum tunneling of the magnetization (QTM). Since the tunneling mechanism might be suppressed via application of a static magnetic field,24 we obtained ac susceptibility vs. temperature in 5 kOe dc field. As expected, both in-phase (χ′) and out-of-phase (χ′′) signals are more visible and show frequency dependent peaks, indicating similar SMMs behavior. Fitting the data to the Arrhenius law (Fig. S11†) gives the pre-exponential factor τ0 = 3.31 × 10−9 s and the effective anisotropy barrier Δ/kB = 35.47 K.
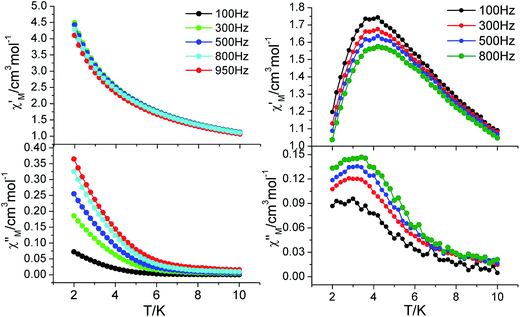 |
| Fig. 6 Temperature dependence of the in-phase and out-of-phase components of the ac magnetic susceptibility for 3 in zero (left) and 5 kOe (right) dc fields with an oscillation of 2 Oe. | |
Conclusion
In conclusion, five new cyclic lanthanide-radical compounds [Ln(hfac)3(NIT-3PyPh)]2 have been synthesized. The investigation of the dynamics of the magnetization for complexes 2 and 3 shows that they exhibit SMMs behavior; especially, the peaks of the out-of-phase signals are visible in a zero dc field for the Tb compound. Contrary to the other Ln-based SMMs, reports on Tb-based SMMs are rare because the bistability is not guaranteed for non-Kramer Tb(III) ions. The results demonstrated that the substituents of the radicals play a crucial role in the magnetic behaviors, and minor structural changes can induce large differences for magnetic relaxation. This strategy is promising to design new lanthanide-radical SMM molecular magnetic materials.
Acknowledgements
This work was supported by the National Natural Science Foundation of China (No. 21602202), the Natural Science Foundation of Zhejiang Province (No. LQ17B010005), and the Science Foundation of Zhejiang Sci-Tech University (No. 15062094-Y). We thank Professor Licun Li of Nankai University for guidance.
References
-
(a) R. Sessoli, D. Gatteschi, A. Caneschi and M. A. Novak, Nature, 1993, 365, 141 CrossRef CAS;
(b) R. Sessoli, H. L. Tsai, A. R. Schake, S. Wang, J. B. Vincent, K. Folting, D. Gatteschi, G. Christou and D. N. Hendrickson, J. Am. Chem. Soc., 1993, 115, 1804 CrossRef CAS.
- M. Mannini, F. Pineider, P. Sainctavit, C. Danieli, E. Otero, C. Sciancalepore, A. M. Talarico, M. A. Arrio, A. Cornia, D. Gatteschi and R. Sessoli, Nat. Mater., 2009, 8, 194 CrossRef CAS PubMed.
-
(a) M. N. Leuenbergger and D. Loss, Nature, 2001, 410, 789 CrossRef PubMed;
(b) A. Ardavan, O. Rival, J. J. L. Morton, S. J. Blundell, A. M. Tyryshkin, G. A. Timco and R. E. P. Winpenny, Phys. Rev. Lett., 2007, 98, 057201 CrossRef PubMed.
-
(a) L. Bogani and W. Wernsdorfer, Nat. Mater., 2008, 7, 179 CrossRef CAS PubMed;
(b) M. J. Graham, J. M. Zadrozny, M. Shiddiq, J. S. Anderson, M. S. Fataftah, S. Hill and D. E. Freedman, J. Am. Chem. Soc., 2014, 136, 7623 CrossRef CAS PubMed.
-
(a) R. Sessoli and A. K. Powell, Coord. Chem. Rev., 2009, 253, 2328 CrossRef CAS;
(b) D. N. Woodruff, R. E. P. Winpenny and R. A. Layfield, Chem. Rev., 2013, 113, 5110 CrossRef CAS PubMed.
-
(a) N. Ishikawa, M. Sugita, T. Ishikawa, S. Koshihara and Y. Kaizu, J. Am. Chem. Soc., 2003, 125, 8694 CrossRef CAS PubMed;
(b) S. D. Jiang, B. W. Wang, H. L. Sun and S. Gao, J. Am. Chem. Soc., 2011, 133, 4730 CrossRef CAS PubMed;
(c) S. Cardona-Serra, J. M. Clemente-Juan, E. Coronado, A. Gaita-Ariño, A. Camón, M. Evangelisti, F. Luis, M. J. Martífnez-Pérez and J. Sesé, J. Am. Chem. Soc., 2012, 134, 14982 CrossRef CAS PubMed;
(d) J. Liu, Y. C. Chen, J. L. Liu, V. Vieru, L. Ungur, J. H. Jia, L. F. Chibotaru, Y. Lan, W. Wernsdorfer, S. Gao, X. M. Chen and M. L. Tong, J. Am. Chem. Soc., 2016, 138, 5441 CrossRef CAS PubMed.
-
(a) R. J. Blagg, C. A. Muryn, E. J. L. Mclnnes, F. Tuna and R. E. P. Winpenny, Angew. Chem., Int. Ed., 2011, 50, 6530 CrossRef CAS PubMed;
(b) Y. F. Bi, X. T. Wang, W. P. Liao, X. W. Wang, R. P. Deng, H. J. Zhang and S. Gao, Inorg. Chem., 2009, 48, 11743 CrossRef CAS PubMed;
(c) X. J. Zhang, V. Vieru, X. W. Feng, J. L. Liu, Z. J. Zhang, B. Na, W. Shi, B. W. Wang, A. K. Powell, L. F. Chibotaru, S. Gao, P. Cheng and R. Jeffrey, Angew. Chem., Int. Ed., 2015, 54, 9861 CrossRef CAS PubMed;
(d) R. J. Blagg, L. Ungur, F. Tuna, J. Speak, P. Comar, D. Collison, W. Wernsdorfer, E. J. L. Mclnnes, L. F. Chibotaru and R. E. P. Winpenny, Nat. Chem., 2013, 5, 673 CrossRef CAS PubMed.
-
(a) G. Novitchi, W. Wemsdorfer, L. F. Chibotaru, J. P. Costes, C. E. Anson and A. K. Powell, Angew. Chem., Int. Ed., 2009, 48, 1614 CrossRef CAS PubMed;
(b) T. C. Stamatatos, S. J. Teat, W. Wernsdorfer and G. Christou, Angew. Chem., Int. Ed., 2009, 48, 521 CrossRef CAS PubMed;
(c) V. M. Mereacre, A. M. Ako, R. Clérac, W. Wernsdorfer, G. Filoti, J. Bartolomé, C. E. Anson and A. K. Powell, J. Am. Chem. Soc., 2007, 129, 9248 CrossRef CAS PubMed;
(d) M. Andruh, J.-P. Costes, C. Diaz and S. Gao, Inorg. Chem., 2009, 48, 3342 CrossRef CAS PubMed;
(e) J. L. Liu, J. Y. Wu, Y. C. Chen, V. Mereacre, A. K. Powell, L. Ungur, L. F. Chibotaru, X. M. Chen and M. L. Tong, Angew. Chem., Int. Ed., 2014, 53, 12966 CrossRef CAS PubMed;
(f) T. Pugh, N. F. Chilton and R. A. Layfield, Angew. Chem., Int. Ed., 2016, 55, 11082 CrossRef CAS PubMed.
-
(a) M. Andruh, I. Ramade, E. Codjovi, O. Guillou, O. Kahn and J. C. Trombe, J. Am. Chem. Soc., 1993, 115, 1822 CrossRef CAS;
(b) J.-P. Costes, F. Dahan, A. Dupuis and J.-P. Laurent, Chem.–Eur. J., 1998, 4, 1616 CrossRef CAS;
(c) F. Habib and M. Murugesu, Chem. Soc. Rev., 2013, 42, 3278 RSC.
-
(a) J. D. Rinehart, M. Fang, W. J. Evans and J. R. Long, J. Am. Chem. Soc., 2011, 133, 14236 CrossRef CAS PubMed;
(b) G. Poneti, K. Bernot, L. Bogani, A. Caneschi, R. Sessoli, W. Wernsdorfer and D. Gatteschi, Chem. Commun., 2007, 1807 RSC;
(c) K. Bernot, L. Bogani, A. Caneschi, D. Gatteschi and R. Sessoli, J. Am. Chem. Soc., 2006, 128, 7947 CrossRef CAS PubMed;
(d) F. Pointillart, B. L. Guennic, S. Golhen, O. Cador and L. Ouahab, Chem. Commun., 2013, 49, 11632 RSC;
(e) E. M. Fatila, M. Rouzières, M. C. Jennings, A. J. Lough, R. Clérac and K. E. Preuss, J. Am. Chem. Soc., 2013, 135, 9596 CrossRef CAS PubMed;
(f) S. Demir, J. M. Zadrozny, M. Nippe and J. R. Long, J. Am. Chem. Soc., 2012, 134, 18546 CrossRef CAS PubMed;
(g) S. G. Reis, M. Briganti, S. Soriano, G. P. Guedes, S. Calancea, C. Tiseanu, M. A. Novak, M. A. del Águila-Sánchez, F. Totti, F. Lopez-Ortiz, M. Andruh and M. G. F. Vaz, Inorg. Chem., 2016, 55, 11676 CrossRef CAS PubMed;
(h) F. Pointillart, J. Jung, R. Berraud-Pache, B. L. Guennic, V. Dorcet, S. Golhen, O. Cador, O. Maury, Y. Guyot, S. Decurtins, S.-X. Liu and L. Ouahab, Inorg. Chem., 2015, 54, 5384 CrossRef CAS PubMed.
-
(a) N. Zhou, Y. Ma, C. Wang, G. F. Xu, J. K. Tang, J. X. Xu, S. P. Yan, P. Cheng, L. C. Li and D. Z. Liao, Dalton Trans., 2009, 8489 RSC;
(b) A. Lannes, M. Intissar, Y. Suffren, C. Reber and D. Luneau, Inorg. Chem., 2014, 53, 9548 CrossRef CAS PubMed;
(c) X. L. Wang, L. C. Li and D. Z. Liao, Inorg. Chem., 2010, 49, 4735 CrossRef CAS PubMed;
(d) A. Lannes, M. Intissar, Y. Suffren, C. Reber and D. Luneau, Inorg. Chem., 2014, 53, 9548 CrossRef CAS PubMed.
-
(a) J. X. Xu, Y. Ma, D. Z. Liao, G. F. Xu, J. K. Tang, C. Wang, N. Zhou, S. P. Yan, P. Cheng and L. C. Li, Inorg. Chem., 2009, 48, 8890 CrossRef CAS PubMed;
(b) X. L. Mei, R. N. Liu, C. Wang, P. P. Yang, L. C. Li and D. Z. Liao, Dalton Trans., 2012, 2904 RSC;
(c) F. Pointillart, K. Bernot, G. Poneti and R. Sessoli, Inorg. Chem., 2012, 51, 12218 CrossRef CAS PubMed;
(d) R. N. Liu, C. M. Zhang, L. C. Li, D. Z. Liao and J.-P. Sutter, Dalton Trans., 2012, 12139 RSC;
(e) H. X. Tian, R. N. Liu, X. L. Wang, P. P. Yang, Z. X. Li, L. C. Li and D. Z. Liao, Eur. J. Inorg. Chem., 2009, 4498 CrossRef CAS.
-
(a) K. Bernot, F. Pointillart, P. Rosa, M. Etienne, R. Sessoli and D. Gatteschi, Chem. Commun., 2010, 46, 6458 RSC;
(b) S. G. Reis, M. Briganti, D. O. T. A. Martins, H. Akpinar, S. Calancea, G. P. Guedes, S. Soriano, M. Andruh, R. A. A. Cassaro, P. M. Lahti, F. Totti and M. G. F. Vaz, Dalton Trans., 2016, 2936 RSC;
(c) X. Li, T. Li, L. Tian, Z. Y. Liu and X. G. Wang, RSC Adv., 2015, 5, 74864 RSC.
-
(a) K. E. Vostrikova, D. Luneau, W. Wernsdorfer, P. Rey and M. Verdaguer, J. Am. Chem. Soc., 2000, 122, 718 CrossRef CAS;
(b) F. L. de Panthou, E. Belorizky, R. Calemczuk, D. Luneau, C. Marcenat, E. Ressouche, P. Turek and P. Rey, J. Am. Chem. Soc., 1995, 117, 11247 CrossRef.
-
(a) L. Bogani, C. Sangregorio, R. Sessoli and D. Gatteschi, Angew. Chem., Int. Ed., 2005, 44, 5817 CrossRef CAS PubMed;
(b) R. N. Liu, Y. Ma, P. P. Yang, X. Y. Song, G. F. Xu, J. K. Tang, L. C. Li, D. Z. Liao and S. P. Yan, Dalton Trans., 2010, 3321 RSC.
- M. Zhu, M. Yang, J. J. Wang, H. D. Li and L. C. Li, Chem.–Asian J., 2016, 11, 1900 CrossRef CAS PubMed.
-
(a) G. M. Sheldrick, SHELXL-2014, Program for Structures Solution, University of Göttingen, Germany, 1997 Search PubMed;
(b) G. M. Sheldrick, SHELXS-2014, Program for Structures Refinement, University of Göttingen, Germany, 1997 Search PubMed.
-
(a) D. Casanova, M. Llunell, P. Alemany and S. Alvarez, Chem.–Eur. J., 2005, 11, 1479 CrossRef CAS PubMed;
(b) M. Llunell, D. Casanova, J. Cirera, P. Alemany and S. Alvarez, SHAPE, version 2.1, University of Barcelona, Barcelona, 2013 Search PubMed.
- C. Benelli, A. Caneschi, D. Gatteschi and L. Pardi, Inorg. Chem., 1992, 31, 741 CrossRef CAS.
- T. Kanetomo and T. Ishida, Inorg. Chem., 2014, 53, 10794 CrossRef CAS PubMed.
-
(a) C. Benelli, A. Caneschi, D. Gatteschi, L. Pardi, P. Rey, D. P. Shum and R. L. Carlin, Inorg. Chem., 1989, 28, 272 CrossRef;
(b) R. N. Liu, L. C. Li, X. L. Wang, P. P. Yang, C. Wang, D. Z. Liao and J.-P. Sutter, Chem. Commun., 2010, 46, 2566 RSC;
(c) J.-P. Sutter, M. L. Kahn, S. Golhen, L. Ouahab and O. Kahn, Chem.–Eur. J., 1998, 4, 571 CrossRef CAS;
(d) M. Yang, H. D. Li and L. C. Li, Inorg. Chem. Commun., 2017, 76, 59 CrossRef CAS.
-
(a) J. J. Borrás-Almenar, J. M. Clemente-Juan, E. Coronado and B. S. Tsukerblat, Comput. Chem., 2001, 22, 985 CrossRef;
(b) J. J. Borrás-Almenar, J. M. Clemente-Juan, E. Coronado and B. S. Tsukerblat, Inorg. Chem., 1999, 38, 6081 CrossRef.
-
(a) C. Benelli, A. Caneschi, D. Gatteschi, J. Laugier and P. Rey, Angew. Chem., Int. Ed., 1987, 26, 913 CrossRef;
(b) C. Lescop, D. Luneau, P. Rey, G. Bussière and C. Reber, Inorg. Chem., 2002, 41, 5566 CrossRef CAS PubMed.
- L. Thomas, F. Lionti, R. Ballou, D. Gatteschi, R. Sessoli and B. Barbara, Nature, 1996, 383, 145 CrossRef CAS.
Footnote |
† Electronic supplementary information (ESI) available. CCDC 1538537–1538541. For ESI and crystallographic data in CIF or other electronic format see DOI: 10.1039/c7ra06310e |
|
This journal is © The Royal Society of Chemistry 2017 |