DOI:
10.1039/C7RA05994A
(Paper)
RSC Adv., 2017,
7, 46164-46170
Phloroglucinol accelerates the regeneration of liver damaged by H2O2 or MNZ treatment in zebrafish
Received
29th May 2017
, Accepted 14th September 2017
First published on 28th September 2017
Abstract
The liver is a vital organ in vertebrates and is vulnerable to oxidative stress that initiates a cascade of intracellular toxic events that lead to activation of the redox system and subsequent cell death, causing chronic liver diseases. Herein, we investigate the protective effect of phloroglucinol (PG), which is a mono-unit of phlorotannins isolated from the brown marine alga Ecklonia cava, on oxidative stress-induced damage in zebrafish. PG is found to reduce H2O2-induced toxicity in addition to H2O2-induced oxidative stress damage. Consequently, PG reduces H2O2-induced hepatocyte death. Moreover, PG accelerates liver regeneration after metronidazole (MNZ)-induced apoptosis. These results clearly indicate that PG possesses prominent antioxidant activity in vivo. Therefore, it could be a potential therapeutic agent for the prevention or treatment of liver diseases associated with oxidative stress.
1. Introduction
Oxidative stress arises due to an imbalance between the production of reactive oxygen species (ROS) and antioxidant scavenging activity. ROSs can cause oxidative damage to biological macromolecules such as DNA, lipids and proteins. In addition, oxidative stress can induce a variety of cytokines such as TNF-α, which might increase inflammation and apoptosis.1,2 In particular, liver is a vital organ in vertebrates and easily attacked by ROS.3 Oxidative stress is a major pathogenetic event occurring in several liver disorders4 due to its redox control. Chronic liver diseases are characterized by increased oxidative stress, regardless of the cause of the liver disorder.
On the other hand, antibiotics used as therapeutic agents are most often associated with hepatotoxicity due to the widespread prescription of these drugs.5 Since the use of antibiotics for disease treatment is indispensable, there is a need for preventive and/or therapeutic agents that can protect and/or regenerate the liver. To date, several natural products have been reported to mitigate drug-induced toxicity.6 The dietary nature and less adverse effects of natural products give them advantages over other candidates for supplementary medication.
Marine algae are composed of a variety of bioactive substances such as polysaccharides, pigments, minerals, peptides and polyphenols with valuable pharmaceutical and biomedical potential.7 In particular, brown marine algae contain various biological benefits.8–12 The biological properties of the brown marine alga Ecklonia cava are attributed to biologically-active secondary metabolites such as phlorotannins including phloroglucinol (PG).13–15 Phlorotannins exhibit a variety of biological properties, including antioxidative,16,17 anti-allergenic,18 neuroprotective,19 anti-inflammatory,20 and memory enhancing effects.21 PG is a structurally powerful radical scavenger and the most abundant compound in phlorotannin extracts from brown marine algae.16–22
The zebrafish (Danio rerio) has been widely used as an alternative animal model to study drug discovery and toxicology,23,24 particularly, liver injury or disease models.25,26 The advantages of the zebrafish include its small size, fecundity, large clutches, low cost, physiological similarity to mammals, and rapid embryonic development, which facilitates morphological monitoring. In particular, its embryos and juveniles are useful for imaging studies because their transparency allows visualization of specific cells, tissues, and organs.27,28 In addition, liver transgenic models are available that can be used to assess efficacy for MNZ-induced liver damage.29,52,53
In this study, we examine the liver protective effect of PG isolated from the brown marine alga E. cava on oxidative stress-induced toxicity caused by H2O2 or MNZ in zebrafish.
2. Materials and methods
2.1. Preparation of PG from E. cava
The marine brown alga E. cava was collected along the coast of Jeju Island, Korea, between October 2013 and March 2014. The sample was washed three times with tap water to remove the salt, epiphytes, and sand attached to its surface. Subsequently, it was carefully rinsed with fresh water, and maintained in a medical refrigerator at −20 °C. Thereafter, the frozen samples were lyophilized and homogenized with a grinder prior to extraction. PG was isolated as previously described9,30 and its chemical structure is presented in Fig. 1A.
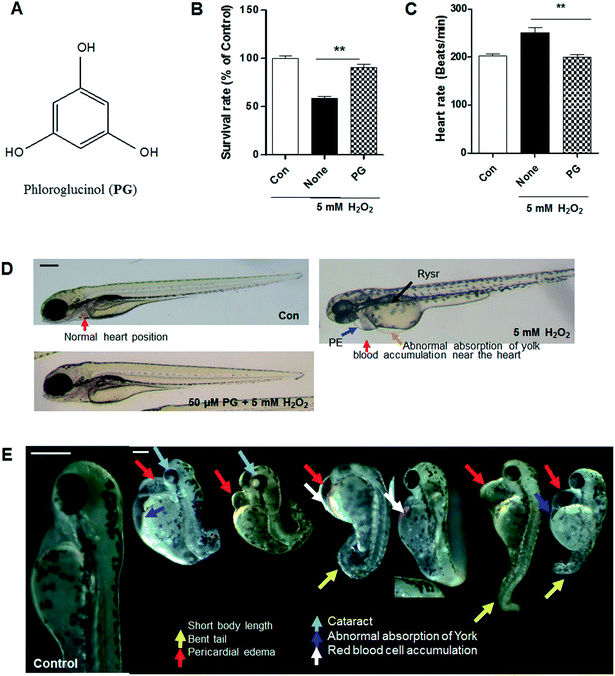 |
| Fig. 1 Phloroglucinol attenuation of H2O2-induced toxicity in zebrafish. (A) Chemical structure of phloroglucinol. (B) Survival rate of zebrafish after treatment with 5 mM H2O2 and pretreatment with 50 μM phloroglucinol. (C) Heart rate of zebrafish after treatment with H2O2 and pretreatment with phloroglucinol. Heart rate was measured at 48 h post-fertilization (hpf), the number of heart rates in 3 min was counted, and the results are expressed as beats per min. (D) Photographs of zebrafish embryos after treatment with H2O2 or pretreatment with phloroglucinol. The embryos were treated with and pretreated with phloroglucinol. (E) Morphological defects from treatment with H2O2. Scale bar: 100 μm. Experiments were performed in triplicate. p values were determined using one-way ANOVA. **p < 0.01. | |
2.2. Zebrafish maintenance
Adult zebrafish were obtained from Korean zebrafish bank (ZOMB) and 20–25 fish were kept in a 3 L acrylic tank under the following conditions: 28.5 °C, with a 14/10 h light/dark cycle. The zebrafish were fed three times a day, 6 d per week, with Tetramin flake food supplemented with live brine shrimps (Artemia salina). Embryos were obtained from natural spawning, which was induced in the morning by turning on the light. Collection of the embryos was completed within 30 min. Zebrafish (Danio rerio) embryos were obtained and maintained according to standard procedures. The wild type and transgenic zebrafish Tg(fabp10:dsRed, ela3l:GFP)gz12
53 was used for protective effect experiments and transgenic zebrafish Tg(fabp10a:CFP-NTR)gt1
53 was used in the experiment for liver regeneration. The Tg(fabp10:dsRed, ela3l:GFP)gz12 transgenic line was generated with the fluorescent protein DsRed and Tg(fabp10a:CFP-NTR)gt1 was generated with the fluorescent protein Cyan fused to NTR and driven by the hepatocyte-specific promoter lfabp.31 The transgenic line was adopted to confirm the PG effect of liver regeneration on antibiotics. The adult zebrafish procedures used in the present study were conducted according to the guidelines established by the Jeju National University Ethics Review Committee for Animal Experiments and approved by the Ethical Committee (IEC) of the Jeju National University.
2.3. Treatment of PG, H2O2, and MNZ to zebrafish embryos
Approximately 7–9 h post-fertilization (hpf), embryos (n = 10–13) were transferred to a 12-well plate and maintained in 1 mL of embryo medium. In the H2O2 treatment experiments, embryos were incubated in the presence of PG prior to the addition of H2O2 (5 mM) up to 120 h post-fertilization (120 hpf). In addition, the embryos were rinsed in embryo medium and anaesthetized before experiments. To ablate the hepatocytes from the embryos, they were treated with freshly prepared 15 mM MNZ at 3 day in the dark. After 24 h, the embryos were rinsed in embryo medium and anaesthetized to observe phase and fluorescence images (Leica, Germany). For confocal microscopy, the embryos were fixed in 4% paraformaldehyde overnight at 4 °C and washed with PBS for 5 min at room temperature. After washing several times with PBS, the whole liver was isolated from the embryos, and subsequently mounted with Vectashield (Vector Laboratories, Burlingame, CA), and observed using a confocal microscope (Zeiss, Germany).
2.4. Measurement of heart rates
The heart rates of both atrium and ventricle were measured at 35 hpf of the experiment to determine sample toxicity.32 Counting and recording of atrial and ventricular contractions were performed for 3 min under a microscope, and results are presented as the average heart rate per min.
2.5. Estimation of intracellular ROS, lipid peroxidation and cell death
Intracellular ROS production using DCFH-DA probe, lipid peroxidation using DPPP probe and cell death using acridine orange staining on zebrafish were examined as previously described.16 The embryos were anaesthetized and individual embryo fluorescence images were observed using a fluorescence microscope equipped with a color digital camera (Zeiss, Germany). The images were analyzed using the AxioVision Microscopy Software (Zeiss, Germany).
2.6. Statistical analysis
All measurements were made in triplicate and all values are presented as mean ± S.E. The results were subjected to an analysis of variance using the Tukey test to analyse differences. Values of p < 0.05 were considered significant.
3. Results
3.1. PG attenuates H2O2-induced toxicity in zebrafish
It was determined that PG exhibits a protective effect against H2O2-induced toxicity in zebrafish. A significantly lower survival rate was observed in the zebrafish treated with H2O2 (around 60% survival), whereas PG pretreatment increased the survival rate to approximately 90% (Fig. 1B). PG alone did not show any toxicity in zebrafish. Heart rate is another indicator of toxicity in the test. A marked increase in heart rate was recorded for the H2O2-treated zebrafish. On the other hand, the PG pretreatment did not generate any heat rate disturbance (Fig. 1C). In the morphological evaluations, the H2O2-treated zebrafish showed several typical morphological defects such as short body lengths, bent tails, pericardial edema, cataracts, abnormal absorption of yolk and red blood cell accumulation (Fig. 1D and E); however, the PG pretreatment prevented conspicuous adverse effects (Fig. 1D), which suggests that PG has protective effects against H2O2-induced oxidative toxicity in zebrafish.
3.2. PG reduced H2O2-induced ROS production, lipid peroxidation, and cell death in zebrafish
PG has been known to be an excellent radical scavenger.9 Therefore, we tested whether PG scavenges H2O2-induced ROS produced in zebrafish. A significantly higher ROS level was observed in the zebrafish treated with H2O2, whereas ROS production was inhibited by the PG pretreatment (Fig. 2A). Lipid peroxidation is also used as an indicator of oxidative stress in cells and tissues. The generation of DPPP oxide was inhibited in zebrafish by the PG pretreatment compared with the control group without PG pretreatment (Fig. 2B). To evaluate whether PG protects against H2O2-induced cell death, acridine orange staining was adopted in the zebrafish. The H2O2 treatment caused a significant increase in acridine orange stained cells, whereas the PG pretreatment reduced the amount of H2O2-induced acridine orange stained cells (Fig. 2C). These results suggest that PG pretreatment inhibits ROS production, lipid peroxidation and cell death, which shows protective properties against H2O2-induced oxidative toxicity in zebrafish.
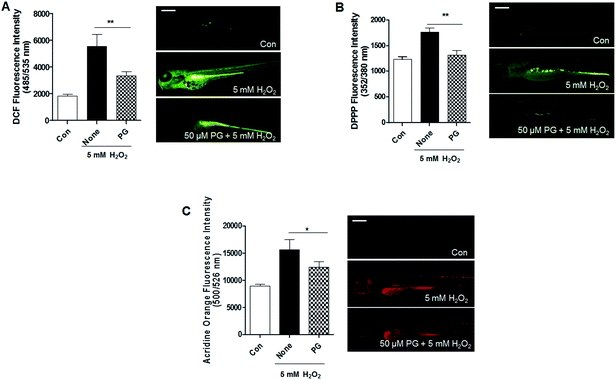 |
| Fig. 2 Phloroglucinol reduces H2O2-induced oxidative stress in zebrafish. (A) Inhibitory effect of phloroglucinol on H2O2-induced ROS generation in zebrafish embryos. (B) Inhibitory effect of phloroglucinol on H2O2-induced lipid peroxidation in zebrafish embryos. (C) Protective effects of phloroglucinol on H2O2-induced cell death and tissue damage in zebrafish embryos. The embryos were treated with 5 mM H2O2 and pretreated with 50 μM phloroglucinol. Scale bar: 100 μm. Experiments were performed in triplicate. p values were determined using one-way ANOVA. **p < 0.01, *p < 0.05. | |
3.3. PG reduced H2O2-induced liver toxicity in zebrafish
The liver is a vital organ in vertebrates and is vulnerable to oxidative stress, which consequently can cause severe hepatic injury. Therefore, we evaluated whether PG attenuates H2O2-induced liver toxicity in transgenic Tg(lfabp-DsRed) zebrafish. As expected, the liver tissues of the normal group showed normal architecture hepatocytes. In the H2O2 treatment group, the liver showed a distorted architecture with cellular necrosis. The group treated with PG and H2O2 displayed a less distorted architecture than the H2O2 treatment group (Fig. 3), which suggests that PG exhibits liver protective effects against H2O2-induced oxidative toxicity in zebrafish.
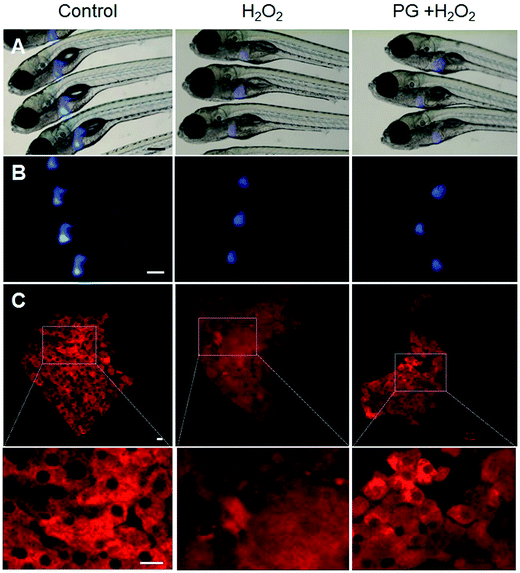 |
| Fig. 3 Phloroglucinol protects from H2O2-induced liver damage in zebrafish. (A) Phase contrast and (B) fluorescence images observed using a fluorescence microscope (Leica, Germany). (C) Images of zebrafish liver observed with a confocal microscope (Zeiss, Germany). The embryos were treated with 5 mM H2O2 and pretreated with 50 μM phloroglucinol. (A and B) Scale bar: 100 μm. (C) Scale bar: 10 μm. | |
3.4. PG accelerates regeneration of liver ablated by MNZ in zebrafish
The hepatocytes of zebrafish are specifically damaged by bacterial nitroreductase (Ntr), which is damaged by MNZ and starts to recover after the removal of MNZ.29 To determine whether PG can regenerate the liver after it is ablated by MNZ, the transgenic Tg(lfabp-cfp-Ntr) line was used. The liver tissues of the normal group showed normal architecture hepatocytes. In the MNZ treatment group, the liver showed a distorted architecture with a lower number of hepatocytes, whereas, in the post-treatment PG group, the liver architecture was mostly similar to that of the control (Fig. 4), which suggests that PG accelerates the regeneration of the zebrafish liver.
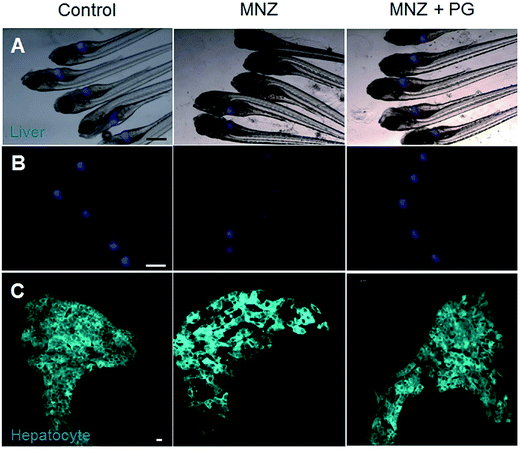 |
| Fig. 4 Phloroglucinol accelerates liver regeneration in MNZ-induced cell death and tissue damaged zebrafish. (A) Phase contrast and (B) fluorescence images observed using a fluorescence microscope (Leica, Germany). (C) Images of zebrafish liver observed with a confocal microscope (Zeiss, Germany). The embryos were treated with 15 mM MNZ and post-treated with 400 μM phloroglucinol. (A and B) Scale bar: 100 μm. (C) Scale bar: 10 μm. | |
4. Discussion
In the present study, we provide compelling evidence of PG as a new therapeutic drug or supplement model by showing the liver protective and regenerative effects of PG on typical pathological features of oxidative damage in zebrafish.
First, we demonstrated that PG prevents H2O2-induced oxidative toxicity by scavenging free radicals in zebrafish. Oxidative stresses induce cellular damage by distorting biochemical components such as enzymes, lipids, proteins and DNA.33 High levels of ROS increase the pathogenesis of a wide variety of diseases.34–38 Cells are protected from ROS-induced damage by a variety of endogenous ROS-scavenging enzymes, chemical compounds, and natural products. Recently, increasing interest has been focused on plants as natural therapeutic agents due to their high antioxidant content.
The physiological benefits of phlorotannins are generally thought to be due to their antioxidant and free radical scavenging properties, even though phlorotannins display other biological activities.14,39,40 Particularly, phlorotannins increase the levels of antioxidant enzymes, including superoxide dismutase, catalase, and glutathione peroxidase,41 and reduce the levels of pro-inflammatory enzymes, including nitric oxide synthase and cyclooxygenase-2 (COX-2),42 thus inhibiting ROS formation. Several ROS-induced pathways blocked by phlorotannins have also been identified; these include ROS-induced apoptotic pathways, the mitochondrial apoptotic pathway, the c-Jun N-terminal kinase (JNK) pathway, and pathways involving NF-kB, caspase-3, and Bax.42–45 In addition, the antioxidant effect of PG has been reported in in vitro systems.46,47 Therefore, the antioxidant activities of PG in assays led us to further investigate its effects in oxidative stress in an in vivo model.
Second, the liver is the organ responsible for the metabolism and detoxification of xenobiotics, and is also the main target for antioxidants. Therefore, we demonstrate that PG prevents and recovers oxidative stress-induced liver toxicity. Antibiotics are hepatotoxic,48 and therefore protection or regeneration of hepatocytes is important in the use of antibiotics. Our results prove that the PG pretreatment significantly decreases oxidative stress-induced liver toxicity as well as PG post-treatment promotes liver regeneration, which may be due to the antioxidant effect of PG. Interestingly, several natural phenolic compounds, such as resveratrol, show protection from hepatocyte toxicity and the molecular mechanisms underlying this action are antioxidant effects.49 Pomegranate, which contains phenolic constituents, has also been reported to reduce hepatotoxicity,50 and pretreatment of resveratrol effectively reversed liver toxicity and resulted in a significant improvement in hepatic function in cat models.51 Accordingly, PG may reduce liver toxicity via the same mechanisms as antioxidant effects.
In conclusion, the results obtained in the present study show that PG isolated from E. cava can effectively protect the liver from damage induced by H2O2. Moreover, PG exhibits liver regenerative effects. These results reveal that PG can be used as an ingredient for nutra/pharmaceutical agents related to liver diseases.
Conflicts of interest
The authors declare that there are no conflicts of interest.
Acknowledgements
This research was supported by the Basic Science Research Program through the Basic Science Research Program through the National Research Foundation of Korea (NRF), funded by the Ministry of Science, ICT and future Planning (2014R1A1A3050501).
References
- A. Voigt, A. Rahnefeld, P. M. Kloetzel and E. Kruger, Front. Physiol., 2013, 4, 42 CrossRef PubMed
. - J. B. Hoek and J. G. Pastorino, Alcohol, 2002, 27, 63–68 CrossRef CAS PubMed
. - V. Sanchez-Valle, N. C. Chavez-Tapia, M. Uribe and N. Mendez-Sanchez, Curr. Med. Chem., 2012, 19, 4850–4860 CrossRef CAS PubMed
. - G. Tell, C. Vascotto and C. Tiribelli, J. Hepatol., 2013, 58, 365–374 CrossRef PubMed
. - M. Robles, E. Toscano, J. Cotta, M. I. Lucena and R. J. Andrade, Curr. Drug Saf., 2010, 5, 212–222 CrossRef CAS PubMed
. - D. Singh, W. C. Cho and G. Upadhyay, Front. Physiol., 2015, 6, 363 Search PubMed
. - T. Kuda, M. Tsunekawa, H. Goto and Y. Araki, J. Food Compos. Anal., 2005, 18, 625–633 CrossRef CAS
. - Y. Athukorala and Y.-J. Jeon, J. Food Sci. Nutr., 2005, 10, 134–139 Search PubMed
. - G.-N. Ahn, K.-N. Kim, S.-H. Cha, C.-B. Song, J. Lee, M.-S. Heo, I.-K. Yeo, N.-H. Lee, Y.-H. Jee and J.-S. Kim, Eur. Food Res. Technol., 2007, 226, 71–79 CrossRef CAS
. - T. Shibata, K. Ishimaru, S. Kawaguchi, H. Yoshikawa and Y. Hama, J. Appl. Phycol., 2008, 20, 705–711 CrossRef CAS
. - K. Kang, J. H. Hye, H. H. Dong, Y. Park, H. K. Seong, H. L. Bong and H. C. Shin, Res. Commun. Mol. Pathol. Pharmacol., 2004, 115–116, 77–95 CAS
. - K. Nagayama, Y. Iwamura, T. Shibata, I. Hirayama and T. Nakamura, J. Antimicrob. Chemother., 2002, 50, 889–893 CrossRef CAS PubMed
. - S. J. Heo, E. J. Park, K. W. Lee and Y. J. Jeon, Bioresour. Technol., 2005, 96, 1613–1623 CrossRef CAS PubMed
. - K. N. Kim, S. J. Heo, C. B. Song, J. Lee, M. S. Heo, I. K. Yeo, K. A. Kang, J. W. Hyun and Y. J. Jeon, Process Biochem., 2006, 41, 2393–2401 CrossRef CAS
. - S. J. Heo, S. C. Ko, S. H. Cha, D. H. Kang, H. S. Park, Y. U. Choi, D. Kim, W. K. Jung and Y. J. Jeon, Toxicol. in Vitro, 2009, 23, 1123–1130 CrossRef CAS PubMed
. - M. C. Kang, S. H. Cha, W. A. Wijesinghe, S. M. Kang, S. H. Lee, E. A. Kim, C. B. Song and Y. J. Jeon, Food Chem., 2013, 138, 950–955 CrossRef CAS PubMed
. - S. J. Heo, S. H. Cha, K. N. Kim, S. H. Lee, G. Ahn, D. H. Kang, C. Oh, Y. U. Choi, A. Affan, D. Kim and Y. J. Jeon, Appl. Biochem. Biotechnol., 2012, 166, 1520–1532 CrossRef CAS PubMed
. - S. Y. Shim, L. Quang-To, S. H. Lee and S. K. Kim, Food Chem. Toxicol., 2009, 47, 555–560 CrossRef CAS PubMed
. - N. Y. Yoon, H. Y. Chung, H. R. Kim and A. J. S. Cho, Fish. Sci., 2008, 74, 200–207 CrossRef CAS
. - H. A. Jung, S. E. Jin, B. R. Ahn, C. M. Lee and J. S. Choi, Food Chem. Toxicol., 2013, 59, 199–206 CrossRef CAS PubMed
. - C. S. Myung, H. C. Shin, H. Y. Bao, S. J. Yeo, B. H. Lee and J. S. Kang, Arch. Pharmacal Res., 2005, 28, 691–698 CrossRef CAS
. - H. Y. Suengmok Cho, Y.-J. Jeon, C. Justin Lee, Y.-H. Jin, N.-I. Baek, S.-M. K. Dongsoo Kim, M. Yoon, H. Yong, M. Shimizu and D. Han, Food Chem., 2012, 132, 1133–1142 CrossRef
. - H. S. Nam and K. S. Hwang, BioMed Res. Int., 2016, 2016, 1473578 Search PubMed
. - L. C. Leung and P. Mourrain, Nat. Chem. Biol., 2016, 12, 468–469 CrossRef CAS PubMed
. - A. D. Vliegenthart, C. S. Tucker, J. Del Pozo and J. W. Dear, Br. J. Clin. Pharmacol., 2014, 78, 1217–1227 CrossRef CAS PubMed
. - W. Goessling and K. C. Sadler, Gastroenterology, 2015, 149, 1361–1377 CrossRef PubMed
. - M. Vittori, B. Breznik, T. Gredar, K. Hrovat, L. Bizjak Mali and T. T. Lah, Radiol. Oncol., 2016, 50, 159–167 Search PubMed
. - A. Vasilyev and I. A. Drummond, Methods in molecular biology, Clifton, N.J., 2012, vol. 886, pp. 55–70 Search PubMed
. - S. Curado, D. Y. Stainier and R. M. Anderson, Nat. Protoc., 2008, 3, 948–954 CrossRef CAS PubMed
. - E.-A. Kim, S.-H. Lee, J.-H. Lee, N. Kang, J.-Y. Oh, S.-h. Cha, G. Ahn, S.-C. Ko, I. P. S. F. Fernando, S.-Y. Kim, S. J. Park, Y.-T. Kim and Y.-J. Jeon, RSC Adv., 2016, 6, 78570–78575 RSC
. - G. M. Her, C. C. Chiang, W. Y. Chen and J. L. Wu, FEBS Lett., 2003, 538, 125–133 CrossRef CAS PubMed
. - T. Y. Choi, J. H. Kim, D. H. Ko, C. H. Kim, J. S. Hwang, S. Ahn, S. Y. Kim, C. D. Kim, J. H. Lee and T. J. Yoon, Pigm. Cell Res., 2007, 20, 120–127 CrossRef CAS PubMed
. - P. Sharma, A. B. Jha, R. S. Dubey and M. Pessarakli, J. Bot., 2012, 2012, 26 Search PubMed
. - J. H. Wu, C. Xu, C. Y. Shan and R. X. Tan, Life Sci., 2006, 78, 622–630 CrossRef CAS PubMed
. - N. V. Goncharov, P. V. Avdonin, A. D. Nadeev, I. L. Zharkikh and R. O. Jenkins, Curr. Pharm. Des., 2015, 21, 1134–1146 CrossRef CAS PubMed
. - K. Matsubara, Y. Matsubara, S. Hyodo, T. Katayama and M. Ito, J. Obstet. Gynaecol. Res., 2010, 36, 239–247 CrossRef CAS PubMed
. - A. Bhattacharyya, R. Chattopadhyay, S. Mitra and S. E. Crowe, Physiol. Rev., 2014, 94, 329–354 CrossRef CAS PubMed
. - T. Nishikawa, M. Brownlee and E. Araki, J. Diabetes Invest., 2015, 6, 137–139 CrossRef CAS PubMed
. - K. A. Kang, K. H. Lee, S. Chae, R. Zhang, M. S. Jung, Y. M. Ham, J. S. Baik, N. H. Lee and J. W. Hyun, J. Cell. Biochem., 2006, 97, 609–620 CrossRef CAS PubMed
. - C.-S. Kong, J.-A. Kim, N.-Y. Yoon and S.-K. Kim, Food Chem. Toxicol., 2009, 47, 1653–1658 CrossRef CAS PubMed
. - M.-C. Kang, S.-M. Kang, G. Ahn, K.-N. Kim, N. Kang, K. W. Samarakoon, M.-C. Oh, J.-S. Lee and Y.-J. Jeon, Environ. Toxicol. Pharmacol., 2013, 35, 517–523 CrossRef CAS PubMed
. - Y. I. Yang, J. H. Woo, Y. J. Seo, K. T. Lee, Y. Lim and J. H. Choi, J. Agric. Food Chem., 2016, 64, 570–578 CrossRef CAS PubMed
. - J. H. Ahn, Y. I. Yang, K. T. Lee and J. H. Choi, J. Cancer Res. Clin. Oncol., 2015, 141, 255–268 CrossRef CAS PubMed
. - Y. J. Jeon, H. S. Kim, K. S. Song, H. J. Han, S. H. Park, W. Chang and M. Y. Lee, Drug Chem. Toxicol., 2015, 38, 180–187 CrossRef CAS PubMed
. - S. Y. Lee, J. Lee, H. Lee, B. Kim, J. Lew, N. Baek and S. H. Kim, J. Agric. Food Chem., 2016, 64, 5508–5514 CrossRef CAS PubMed
. - K. A. Kang, K. H. Lee, S. Chae, R. Zhang, M. S. Jung, Y. M. Ham, J. S. Baik, N. H. Lee and J. W. Hyun, J. Cell. Biochem., 2006, 97, 609–620 CrossRef CAS PubMed
. - B. Queguineur, L. Goya, S. Ramos, M. A. Martin, R. Mateos and L. Bravo, Food Chem. Toxicol., 2012, 50, 2886–2893 CrossRef CAS PubMed
. - J. M. Leitner, W. Graninger and F. Thalhammer, Infection, 2010, 38, 3–11 CrossRef CAS PubMed
. - S. H. Seif El-Din, N. M. El-Lakkany, M. B. Salem, O. A. Hammam, S. Saleh and S. S. Botros, J. Adv. Pharm. Technol. Res., 2016, 7, 99–104 CrossRef PubMed
. - S. Mukherjee, S. Ghosh, S. Choudhury, A. Adhikary, K. Manna, S. Dey, G. Sa, T. Das and S. Chattopadhyay, J. Nutr. Biochem., 2013, 24, 2040–2050 CrossRef CAS PubMed
. - Z. Zhang, L. Gao, Y. Cheng, J. Jiang, Y. Chen, H. Jiang, H. Yu, A. Shan and B. Cheng, BioMed Res. Int., 2014, 2014, 617202 Search PubMed
. - T. Y. Choi, N. Ninov, D. Y. Stainier and D. Shin, Gastroenterology, 2014, 146(3), 776–788 CrossRef CAS PubMed
. - M. Huang, A. Chang, M. Choi, D. Zhou, F. A. Anania and C. H. Shin, Hepatology, 2014, 60(5), 1753–1766 CrossRef CAS PubMed
.
Footnote |
† The authors are contributing equally. |
|
This journal is © The Royal Society of Chemistry 2017 |