DOI:
10.1039/C7RA05670B
(Paper)
RSC Adv., 2017,
7, 41033-41042
Changes in the heavy metal distributions in whole soil and aggregates affected by the application of alkaline materials and phytoremediation
Received
19th May 2017
, Accepted 9th August 2017
First published on 22nd August 2017
Abstract
To explore the combined remedy effect of alkaline materials and plants on the distribution of heavy metals in whole soil and aggregates, a 3 year in situ experiment was carried out in a dual copper (Cu)/cadmium (Cd)-contaminated farmland in this study. Treatment was applied by the addition of soda residue (11.2 t ha−1), apatite (22.3 t ha−1), or lime (4.45 t ha−1), respectively, into the heavy metals-contaminated soil as a single application; Elsholtzia splendens was continually planted for 3 years in all the experimental plots. The total metals amounts and distributions of Cu and Cd in the whole soil and aggregates were then investigated. Our results showed that (1) although the total concentrations of Cu and Cd were slightly increased in the whole soil, their exchangeable concentrations were significantly reduced in both abovementioned treatments; (2) all the abovementioned 3 treatments significantly increased the stability of the dry and wet aggregates when compared with the control treatment; (3) similar to the whole soil, combined remediation groups slightly increased the concentrations of Cu and Cd in the aggregates, but decreased their available and exchangeable concentrations observably. Specifically, the highest concentrations of Cu and Cd were found in the aggregates sized <0.053 mm; however, mass loadings of Cu and Cd were observed in the 0.053–0.25 mm and 0.25–2 mm sized fractions, respectively; moreover, the treatments increased the mass loading of Cu and Cd in the aggregates sized >0.25 mm. In total, the combined remediation adopted in our study dramatically decreased the available concentrations of both Cu and Cd in the whole soil and aggregates. The distribution variations of Cu and Cd caused by passivator-plant combined remediation in the whole soil might be because more metal ions have been transformed into less mobile fractions, whereas the heavy metal distribution differences in the aggregates might be not only correlated with the size of the soil wet aggregates, but also possibly controlled by the soil organic carbon.
Introduction
In most countries, heavy metal contaminated soil is a major concern. Owing to human activities, the issue of contaminated soils in agricultural, industrial, and urban areas has become increasingly acute in recent decades.1–3 To solve this environmental issue, various remediation technologies, such as thermal treatment, flotation, immobilization, and phytoremediation, have been adopted to remediate heavy metal-contaminated soil.4–6 Generally, some of these technologies alone or combined have been proven to be effective to reduce the availability of heavy metals in soil.7,8 However, some technologies are not suitable for practical application due to limitations, such as being time-consuming and costly, or involvement of intensive labor needs; the use of plant-based and chemical additives combined in situ remediation technology has been proven to be one of the cheapest and most effective methods for remediating soils contaminated with heavy metals.9–12
Various soil factors, such as the soil pH, content of organic matter, heavy metal availability, and biological properties, have been used to evaluate the stabilization effect of the various immobilization technologies.13 However, the physical properties of the soil have often been ignored in remediation research. In fact, soil aggregates and bulk density can also play key roles in the soil components and particle composition14 and should thus be taken into consideration. As the basic unit in the soil system, soil aggregates are a product of the interaction of soil and environmental factors. These aggregates have an effect on the adsorption and distribution of plant nutrients and the binding capacity of heavy metals and other organic toxicants in the soil.15,16 A growing body of research has shown that the migration and availability of heavy metals mainly depend on the soil particle size.17 The concentration of heavy metals gradually decreases with the increase in soil particle size, with also a big difference in the bioavailability among different fractions.18,19 Furthermore, soil aggregates comprise a set of soil particles with different sizes combined with each other in an inorganic and organic composition, where the stability of the aggregates has an important influence on the soil quality and on crop growth.20 Heavy metal contamination in soil has intensified the situation of arable soil tension in China to the point where soil remediation is needed to meet the demand for soil reuse for grain production.21 So it is very important to study the composition and stability of soil aggregates and the distribution of heavy metals in soil aggregates after the in situ remediation of soil.
Therefore, the main aims of this study were: (1) to evaluate the effects of three kinds of cheap chemical materials (soda residue, apatite, and lime) on the availability and distribution of Cu and Cd in whole soil, (2) to explore the effects of combined remediation approaches on the distributions of Cu and Cd in soil aggregates, and (3) to explore the possible mechanisms contributing to variations in the distribution of Cu and Cd.
Materials and methods
Experiment design
The experimental site is located in Guixi, Jiangxi Province, China. A subtropical monsoon climate dominates this area, with an annual average precipitation of 1808 mm. Owing to farmers using wastewater containing heavy metals discharged by a local copper smelter for irrigation and due to atmospheric metal depositions and waste residue accumulation, more than 130 hm2 of surrounding farmland is suffering from issues with heavy metal pollution (mainly Cu and Cd), resulting in Cd levels in rice exceeding the acceptable level.22 The concentration of lead (Pb), zinc (Zn), and arsenic (As) were also determined before the start of the field experiment, and it was found that the concentrations of all of these were below the second grade of soil environmental quality standards in China (GB 15618-1995).
Field experiments were conducted in triplicate, and were designed with the land split in to randomized blocks or plots. Each plot was 6 m2 (3 m × 2 m) and the plots were separated by plastic plates. The treatments applied were: (1) 0.5% soda residue (w/w according to the mass of surface 17 cm soil, 11.1 t ha−1, the same below), (2) 1% apatite (22.3 t ha−1), (3) 0.2% lime (4.45 t ha−1), and (4) the control. The choice of the doses in the experiments was based on our previous experimental results, and the main properties of the materials are listed in Table 1. The soil and the materials were fully mixed with an agricultural harrow, and then irrigated with tap water after the application of the materials (500 t ha−1) on December 26, 2012. Elsholtzia splendens were planted with a spacing of 30 cm × 30 cm (70 plants per plot) in every plot on April 26 in 2013, 2014, and 2015, with a compound fertilizer (N
:
P2O5
:
K2O = 15
:
15
:
15) first applied before the plants were planted at a spreading of 0.833 t ha−1. During the study period 2012–2015, these materials were only applied at the start in 2012, while the test crop Elsholtzia splendens was harvested at the beginning of December each year.
Table 1 The physicochemical properties of the tested soila
pH |
SOC (g kg−1) |
Total N (g kg−1) |
Total P (g kg−1) |
Total K (g kg−1) |
Available N (mg kg−1) |
Available P (mg kg−1) |
Available K (mg kg−1) |
CEC (cmol kg−1) |
Total Cu (mg kg−1) |
Total Cd (mg kg−1) |
SOC – soil organic carbon, CEC – cation exchange capacity. |
4.63 |
16.3 |
1.33 |
0.261 |
2.38 |
67.1 |
186 |
54.8 |
8.45 |
517 |
0.410 |
Soil and amendments
Field immobilization remediation was performed throughout the 3 years, whereby soil samples were collected from each plot from an area of 20 cm × 20 cm × 17 cm, with three samples taken from each plot and then mixed together to form a mixed sample. These samples were air dried and sieved using a 5 mm sieve, and the resulting samples were used for the analysis of soil aggregates. Surface soil (0–17 cm) was collected and passed through a 10-mesh sieve for determination of the basic properties and to assess the Cu and Cd concentrations (Table 1).
The Cu and Cd concentrations in the soda residue (particle size 0.25 mm, Sinopec group, Lianyungang soda plant, Jiangsu, China) were 0.412 and 0.0304 mg kg−1, respectively; while those in the apatite (particle size 0.25 mm, Nanzhang Lihua mineral powder factory, Hubei, China) were 9.54 and 1.18 mg kg−1, respectively; and those in the lime (particle size 0.25 mm, building materials market, Jiangxi, China) were 1.36 and 0.87 mg kg−1, respectively; the pH values of the soda residue, apatite, and lime were 10.1, 8.40, and 12.2, respectively.
Aggregates analysis
The dry and wet sieving results were used to measure the soil aggregates according to the methods of Zhang23 and Elliott.24 The dry aggregates were divided into aggregates sized >5 mm, 2–5 mm, 1–2 mm, 0.5–1 mm, 0.25–0.5 mm, and <0.25 mm, while the wet aggregates were divided into aggregates sized >2 mm, 0.25–2 mm, 0.053–0.25 mm, and <0.053 mm. On this basis, the contents of >0.25 mm mechanically stable aggregates (DR0.25) and >0.25 mm water-stable aggregates (WR0.25) were calculated.25
Chemical analysis
Soil chemical properties. The soil pH was measured with a glass electrode at a water
:
soil ratio of 2.5
:
1 (PHS-2CW-CN, Bante, Shanghai, China). The soil organic carbon (SOC) and total nitrogen were determined according to Walkley's method.26 The soil available phosphate (P) and nitrogen (N) contents were measured in accordance with Bingham's method.27 The ammonium acetate method was used to measure the soil cation exchange capacity (CEC).28 The total Cu and Cd contents in the whole soil and aggregates were digested with nitric acid (HNO3), hydrofluoric acid (HF), and perchloric acid (HClO4) (5
:
10
:
5 mL) on an electric heating plate, then the solution content was determined using a flame or graphite furnace atomic absorption spectrophotometer (Model 240AA, Varian, State of California, America). In order to ensure the accuracy of the analysis results, replicate samples, blanks, and a certified reference material (GBW07401, provided by the Institute of Geophysical and Geochemical Exploration, Langfang, Hebei province, China) were included in all the analyses. The available heavy metals in soils were extracted with 0.01 mol L−1 CaCl2 and measured according to Walker's method.29
Sequential extraction of Cu and Cd. Air-dried whole soil was used for the extraction using the modified Tessier sequential extraction procedure following Cui's method,7 where the heavy metals were divided into five operationally defined fractions in the following steps:
(1) Exchangeable fraction. 16 mL of 1 mol L−1 MgCl2 (pH 7.0) was added to a 50 mL centrifuge tube containing 2 ± 0.0001g of soil. The extraction experiment was carried out under stirring at 120 rpm for 2 h at 25 ± 1 °C, then the suspension was centrifuged for 10 min at 4000 rpm, and finally the supernatant was filtered with a 0.45 µm membrane and transferred into a 15 mL centrifuge tube and stored in refrigerator at 4 °C.
(2) Carbonate-bound fraction. 16 mL of 1 mol L−1 CH3COONa (adjusted to pH 5.0 with CH3COOH) was added to the residue soil from (1) and shaken in a reciprocating oscillating machine for 3 h at 25 ± 1 °C.
(3) Fe–Mn oxides-bound fraction. The residue from (2) was shaken with 40 mL of 0.04 mol L−1 NH2OH HCl in 25% (v/v) CH3COOH, followed with occasional agitation for 6 h at 96 ± 3 °C.
(4) Organic matter-bound fraction. 6 mL of 0.02 mol L −1 HNO3 and 10 mL of 30% H2O2 (adjusted to pH 2.0 with HNO3) were added to the residue from (3), and the mixture was heated at 85 ± 3 °C for 2 h in a water bath. Then, 6 mL of 30% H2O2 (adjusted to pH 2.0 with HNO3) was added to the mixture, and the mixture was heated again at 85 ± 3 °C for 3 h. After cooling, 10 mL of 3.2 mol L−1 CH3COONH4 in 20% (v/v) HNO3 was added and the mixture was continuously agitated for 30 min at 25 ± 1 °C.
(5) Residual fraction. The residue from (4) was digested with nitric acid (HNO3), hydrofluoric acid (HF), and perchloric acid (HClO4) (5
:
10
:
5 mL) on an electric heating plate.
Statistical analysis
All the treatments were carried out in triplicate. The means and standard deviations of each treatment were calculated and are presented herein. Differences between the means of the treatments were estimated using one-way ANOVA at a significance level of 0.05 using SPSS 20.0 (IBM SPSS, Somers, NY, USA) when necessary. All the graphics were using Sigmaplot 12.5.
Results and discussion
Soil characteristics
After the application of the soil amendments and with the continuous growth of Elsholtzia splendens, the soil pH became significantly elevated, while the available Cu and Cd (CaCl2–Cu and –Cd) concentrations were significantly decreased (Table 2). Considering the vital role of the soil pH in controlling the soil solubility, mobility, and bioavailability of heavy metals,30–32 the above co-remediation with chemical additives and plants might, through strengthening the soil pH, lead to a lowering of the heavy metal availability. After three years remediation over the whole study period, the SOC contents were significantly increased by the application of these three amendments, respectively. The highest SOC content was observed in the soda residue treatment group, followed by apatite and lime treatment. Also, the SOC concentrations of the different experimental treatments were closely correlated with their corresponding plant biomass (Table 3). The growth of plants might, through increasing the amount of litter and fine roots and through changing the structure of soil aggregates, further lead to an increase in the SOC content.33–35 This means that the plant growth could effectively increase the nutrient supply for microbial activities. No significant changes in the total N and K were observed between different treatments, but the application of apatite could significantly improve the concentration of the total phosphorus in the soil. This might mainly be due to the application of apatite, which adds a large amount of phosphorus to the soil. Also, given that the test area was in a typical acid rain area,36 acid rain may have facilitated the dissolution of apatite.37,38 Thus the available phosphorus content in the soil could be improved, which would ultimately lead to promoting phosphorus absorption in plants.
Table 2 Soil properties after remediation for three yearsa
Treatment |
pH |
SOC, g kg−1 |
Total N, g kg−1 |
Total P, g kg−1 |
Total K, g kg−1 |
CaCl2–Cu, mg kg−1 |
CaCl2–Cd, mg kg−1 |
Total Cu, mg kg−1 |
Total Cd, mg kg−1 |
The different lowercase letters indicate significant differences between treatments in the same year (n = 3, P < 0.05). |
Control |
4.59 ± 0.30b |
16.4 ± 0.13b |
1.34 ± 0.13a |
0.26 ± 0.03b |
2.38 ± 0.36a |
48.7 ± 5.35a |
1.08 × 10−1 ± 2.01 × 10−2a |
517 ± 61.7a |
0.401 ± 0.015a |
Soda residue |
5.27 ± 0.21a |
21.8 ± 0.30a |
1.47 ± 0.01a |
0.21 ± 0.01b |
2.29 ± 0.21a |
36.0 ± 6.88ab |
6.29 × 10−2 ± 8.41 × 10−3b |
561 ± 69.0a |
0.404 ± 0.069a |
Apatite |
5.49 ± 0.06a |
18.6 ± 0.15ab |
1.37 ± 0.21a |
0.40 ± 0.03a |
2.42 ± 0.05a |
14.1 ± 4.43c |
5.89 × 10−2 ± 1.15 × 10−2b |
592 ± 55.1a |
0.419 ± 0.015a |
Lime |
5.27 ± 0.35a |
18.0 ± 0.17ab |
1.57 ± 0.15a |
0.21 ± 0.01b |
2.51 ± 0.21a |
31.5 ± 6.27b |
7.45 × 10−2 ± 1.12 × 10−2ab |
554 ± 43.0a |
0.426 ± 0.029a |
Table 3 Shoot biomass of Elsholtzia splendens from each treatment over the 3 year study perioda
Treatment |
Shoot biomass (kg dry weight per plot per year) |
2013 |
2014 |
2015 |
Total shoot biomass |
The different lowercase letters indicate significant differences between treatments in the same year (n = 3, P < 0.05); — indicates no plant growth. |
Control |
— |
— |
— |
— |
Soda residue |
7.29 ± 0.904ab |
9.53 ± 0.822a |
7.31 ± 0.945a |
24.1 ± 0.265a |
Apatite |
4.90 ± 1.04b |
8.24 ± 1.39a |
7.20 ± 0.291a |
21.2 ± 1.34b |
Lime |
7.79 ± 1.58a |
7.10 ± 1.04a |
6.28 ± 1.41a |
20.3 ± 1.15b |
In addition, the total Cu and Cd concentrations were slightly increased in the combined repaired soils (Table 2). These results appeared to deviate from the remediation objective of soil remediation, which is to remove heavy metals. Previous studies mostly reported that the total heavy metals concentration showed no significant differences or only a slight decrease relative to that of the control.39 This was mainly because of the severe atmospheric deposition in this area, whereby heavy metals could continually enter the soil,40 while the surface runoff and leaching amount varied greatly among the different treatments. This could lead to an increased adsorption and retention capacity of Cu and Cd, and a strengthened immobilization efficiency of Cu and Cd as well in the treated soil as compared with those in untreated soil.41,42
Chemical fractions of Cd and Cu
The soil heavy metal forms can be classified into solid and solution phases, where heavy metals are generally more abundant in the solid phase.43 Solid-phase heavy metals can be further divided into different fractions, whose solubility, mobility, bioavailability, and potential environmental toxicity cannot be fully reflected by any single-step extraction method.44 In the present study, a sequential extraction procedure was used to solve the problem. The different fractions of Cu and Cd in the soil were listed in Tables 4 and 5, respectively. The percentage distributions of the Cu and Cd fractions in the soil are shown in Fig. 1a and b, respectively.
Table 4 Effects of soda residue, apatite, and lime application on Cu fractions. EXC = exchangeable fraction, CA = carbonate-bound fraction, Fe–Mn = Fe–Mn oxides-bound fraction, OM = organic matter-bound fraction, RES = residual fraction. Different lowercase letters indicate significant differences between treatments after three years (n = 3, P < 0.05)
Treatment |
EXC, mg kg−1 |
CA, mg kg−1 |
Fe–Mn, mg kg−1 |
OM, mg kg−1 |
RES, mg kg−1 |
Control |
71.4 ± 6.66a |
36.2 ± 1.94b |
74.2 ± 5.20a |
100 ± 4.88a |
111 ± 8.92b |
Soda residue |
56.3 ± 11.0ab |
59.2 ± 10.8ab |
121 ± 28.0a |
88.8 ± 9.68ab |
137 ± 25.0ab |
Apatite |
25.2 ± 11.3c |
81.9 ± 21.9a |
133 ± 39.6a |
94.7 ± 15.3a |
127 ± 17.3ab |
Lime |
41.2 ± 12.6bc |
68.7 ± 4.77ab |
113 ± 2.67a |
68.3 ± 3.27b |
162 ± 5.78a |
Table 5 Effects of soda residue, apatite, and lime application on Cd fractions. EXC = exchangeable fraction, CA = carbonate-bound fraction, Fe–Mn = Fe–Mn oxides-bound fraction, OM = organic matter-bound fraction, RES = residual fraction. Different lowercase letters indicate significant differences between treatments after three years (n = 3, P < 0.05)
Treatment |
EXC, µg kg−1 |
CA, µg kg−1 |
Fe–Mn, µg kg−1 |
OM, µg kg−1 |
RES, µg kg−1 |
Control |
158 ± 29.4a |
14.9 ± 3.85a |
30.2 ± 8.10b |
38.8 ± 15.3a |
162 ± 46.5c |
Soda residue |
91.8 ± 12.3b |
12.7 ± 2.76a |
40.7 ± 4.83a |
26.5 ± 7.97a |
224 ± 11.4a |
Apatite |
86.0 ± 16.8b |
17.0 ± 5.46a |
47.1 ± 11.4a |
26.0 ± 5.12a |
229 ± 33.1a |
Lime |
108 ± 4.82b |
14.9 ± 1.48a |
55.7 ± 1.19a |
37.2 ± 3.71a |
214 ± 6.81b |
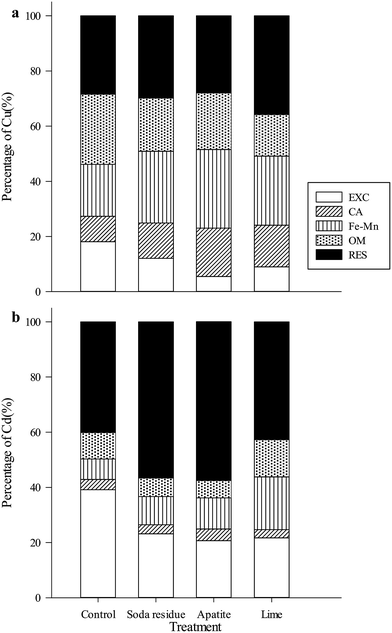 |
| Fig. 1 Effects of soda residue, apatite, and lime application on the percentages of: (a) Cu and (b) Cd fractions after three years. EXC = exchangeable fraction, CA = carbonate-bound fraction, Fe–Mn = Fe–Mn oxides-bound fraction, OM = organic matter-bound fraction, RES = residual fraction. | |
Without any treatment (i.e., the control group), the RES fraction of Cu (111 mg kg−1, 28.4%) was the most abundant, followed by the OM-fraction (100 mg kg−1, 25.4%), the Fe–Mn fraction (74.2 mg kg−1, 18.8%), the EXC fraction (71.4 mg kg−1, 18.1%), and the CA fraction (36.2 mg kg−1, 9.18%). With the amendments application (soda residue, apatite, or lime), the exchangeable fractions were significantly reduced to 56.3 mg kg−1 (12.1%), 25.2 mg kg−1 (5.50%), and 41.2 mg kg−1 (8.99%) in turn; while the CA fractions were increased to 59.2 mg kg−1 (12.8%), 81.9 mg kg−1 (17.5%), and 68.7 mg kg−1 (15.1%), respectively; the RES-fractions were increased to 137 mg kg−1 (29.8%), 127 mg kg−1 (28.0%), and 162 mg kg−1 (35.8%), respectively. No significant differences in the other factions were obtained as compared with the control.
In the untreated soil, the main form of Cd was the RES fraction (162 µg kg−1, 40.1%), similar to the distribution of Cu. Additionally, the exchangeable fraction (158 µg kg−1, 39.2%) was markedly greater than the CA fraction (14.9 µg kg−1, 3.69%). With the amendments application (soda residue, apatite, or lime), the exchangeable fractions of Cd were significantly reduced to 91.8 µg kg−1 (23.2%), 86.0 µg kg−1 (21.2%), and 108 µg kg−1 (25.2%), respectively, while the RES and Fe–Mn fractions were markedly increased to 224 and 40.7 µg kg−1 (56.6%, 10.3%), 239 and 47.1 µg kg−1 (59.1%, 11.3%), and 144 and 55.7 µg kg−1 (33.6%, 19.1%), respectively. No significant differences in the other two factions were observed when compared with the control.
The exchangeable fraction of heavy metals is considered the most easily mobile and available45 form. Additionally, the carbonate-, Fe–Mn oxides-, and organic matter-bound fractions of heavy metals are potentially available to plants and microorganisms, while the residual fraction was dominant among the five fractions. The exchangeable and CA-bound fractions of Cu are often present in uncontaminated soil at low concentrations (<10% of total Cu).46 In the present study, the exchangeable and carbonate-bound fractions of Cu constituted 27.3% of the total Cu in the untreated soil, which made it inappropriate for agricultural use. We conjectured that the source of Cu was mainly artificial and introduced from exhaust gases, dust, and wastewater from the nearby copper smelter.
Additionally, the exchangeable fraction can be used to evaluate the bioavailability and environmental toxicity of heavy metals.47,48 In the current study, the concentrations of Cu and Cd were decreased in the exchangeable fraction but increased in the Fe–Mn oxides-bound and residual fractions after the amendments application compared with that of the control. This result illustrated that all three kinds of amendments could significantly reduce the bioavailability of Cu and Cd in the contaminated soil, and thus were effective in remediating the soils contaminated with the two metals. The exchangeable fraction of Cd (39.2%) was greater than that of Cu (18.1%) in the untreated soil, indicating a higher mobility of Cd than that of Cu at this contaminated site.
Distribution of the soil aggregate fraction
As the basic unit of soil structure, soil aggregates are made up of individual soil particles and organic matter, which makes the, one of the most important factors to determine soil fertility. The size and stability of soil aggregates exert direct effects on soil aeration and water availability, and are closely related to the soil's physical, chemical, and biological characteristics and plant growth.49 The content of mechanical stability aggregates (DR0.25) were 46.7–55.1% before the plants were harvested in 2015 (Table 6) in this study. This content was lower than that in many other reports,50,51 and highlighted the poor physical structure of the soil in this area. This might be related to the serious soil pollution in this area, which resulted in difficult plant growth and a higher intensity of surface erosion, which both eventually have led to deterioration of the soil structure. The content of the mechanical stability aggregates (DR0.25) increased by 2.57–17.9% after 3 years of combined remediation of three different materials and Elsholtzia splendens (Table 6) over the course of this 3 year study. The combined remediation mainly changed the content in the 2–5 mm, 1–2 mm, and 0.25–0.5 mm sized aggregates by 12.8–29.4%, 18.5–29.8%, and 18.5–22.6% (Table 6), respectively.
Table 6 Composition of aggregates in the soil after the remediation (%). Mechanical stability aggregates (DR0.25) and water-stable aggregates (WR0.25). Different lowercase letters indicate significant differences between treatments after three years (n = 3, P < 0.05)
Treatment |
Sizes of the soil mechanical-stable aggregates |
>5 mm |
5–2 mm |
2–1 mm |
1–0.5 mm |
0.5–0.25 mm |
<0.25 mm |
DR0.25 |
Control |
6.77 ± 1.59a |
8.61 ± 1.86ab |
4.74 ± 1.48b |
7.48 ± 0.589a |
17.4 ± 1.23c |
51.4 ± 3.22a |
46.7 ± 2.58b |
Soda residue |
7.22 ± 0.29a |
8.29 ± 0.418b |
6.21 ± 0.287ab |
5.22 ± 1.13b |
22.9 ± 1.16a |
49.7 ± 1.82ab |
50.1 ± 1.86ab |
Apatite |
7.39 ± 0.855a |
9.41 ± 0.442ab |
6.20 ± 0.686ab |
6.66 ± 0.843ab |
17.3 ± 0.605c |
51.4 ± 2.25a |
47.9 ± 0.447b |
Lime |
7.26 ± 1.58a |
11.6 ± 1.42a |
7.63 ± 1.10a |
7.31 ± 0.397a |
20.6 ± 1.27b |
44.0 ± 3.43b |
55.1 ± 3.29a |
Treatment |
Sizes of the soil water-stable aggregates |
>2 mm |
0.25–2 mm |
0.053–0.25 mm |
<0.053 mm |
WR0.25 |
Control |
17.9 ± 0.489b |
31.2 ± 2.11a |
45.8 ± 2.82a |
3.76 ± 0.212a |
49.1 ± 1.63b |
Soda residue |
25.3 ± 0.600a |
31.5 ± 0.441a |
38.4 ± 0.855b |
3.88 ± 0.211a |
56.8 ± 1.04a |
Apatite |
23.2 ± 2.26a |
28.9 ± 0.700a |
40.7 ± 2.26ab |
4.12 ± 0.838a |
52.1 ± 2.96ab |
Lime |
24.5 ± 2.88a |
30.3 ± 0.106a |
38.0 ± 2.63b |
3.75 ± 0.522a |
54.8 ± 2.98ab |
For the water-stable aggregates, the content of water-stable aggregates (WR0.25) ranged from 49.1% to 56.8% (Table 6). The combined remediation significantly increased the content of water-stable aggregates by 6.11–15.7%. This indicated that the effect of vegetation restoration on the mechanical stability aggregates was more obvious than on the water-stable aggregates during the in situ remediation. The combined remediation mainly changed the content of aggregates sized >2 mm by 29.6–41.3%.
This study clearly showed that the content of mechanical stability aggregates DR0.25 and water-stable aggregates WR0.25 in the soil were greatly increased after the remediation, especially the concentration of aggregates sized >2 mm. These results indicated that the combined remediation measures had positively contributed to the formation of soil stable aggregates in this region, which could further support re-establishing vegetation (Elsholtzia splendens). The stability of the soil aggregates was closely related to the level of organic carbon. Soil organic carbon could promote the cementation of soil particles, whereby inorganic colloids play a dominant role during the formation of agglomerates in bare land because of the absence of organic matter.52 Vegetation restoration increased the amount of litter and root exudates, thus benefiting the accumulation of organic carbon (Table 2), especially for new organic carbon, where the new organic carbon in soil could cement smaller aggregates to form stable aggregates,53 which consequently improved the content of stability aggregates.
Concentration of SOC in the wet aggregate fractions
The largest concentration of SOC was found in the aggregate size fraction 0.25–2 mm and ranged from 18.6 to 23.3 g kg−1, followed by those in the fractions sized >2 mm (16.4–19.9 g kg−1), <0.053 mm (10.8–12.8 g kg−1), 0.053–0.25 mm (9.24–10.9 g kg−1) in turn (Fig. 2). These results confirmed that the concentration of SOC in >0.25 mm aggregates was greater than that in smaller aggregates; this result was similar to John,54 who found that the SOC content in micro-aggregates was lower than that in macro-aggregates, and the SOC content in >2 mm aggregates was 3.62 times higher than that in <0.053 mm aggregates in the farmland tested. In addition, the combined remediation significantly increased the concentration of SOC in all the aggregate fractions compared with the control, and in the >2 and 0.053–0.25 mm sized aggregate fractions it followed the order: soda residue > lime > apatite > control (Fig. 2); while in the 0.25–2 and <0.053 mm sized aggregate fractions, it followed the order: apatite > soda residue > lime > control. This might be due to the larger Elsholtzia splendens biomass exhibited in the soda residue- and lime-treated soils (Table 3), where the increase of biomass then resulted in an increase in plant litter and rhizosphere exudates in the soil, which further elevated the content of organic carbon in the soil.55
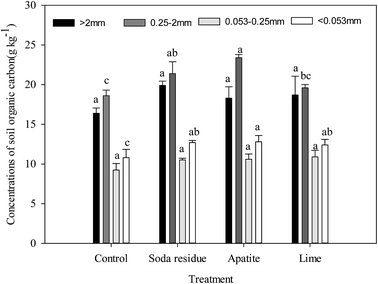 |
| Fig. 2 Concentrations of soil organic carbon (g kg−1) in different size fractions of wet soil aggregates. Different lowercase letters indicate significant differences between treatments after three years (n = 3, P < 0.05). | |
Content of Cu and Cd in the wet aggregate fractions
Previous studies have shown that wet aggregates can be used to study changes in the SOC content and microbial community structure as well as the distribution of heavy metals in long-term soil use.14,56 Moreover, in our study, a subtropical monsoon climate dominates the area, with an annual average precipitation of 1808 mm, therefore, it was necessary to evaluate the water stability of the aggregates. Soil resistance against water erosion can be well demonstrated by WR0.25.57 Thus, this study was mainly focused on examining the content of Cu and Cd in the wet soil aggregate fractions.
The highest concentrations of Cu and Cd were found in the <0.053 mm sized fraction, followed by the 0.25–2, >2, and 0.053–0.25 mm sized fractions (Table 7). The concentrations of Cu and Cd in both the >2 and 0.25–2 mm sized fractions followed the order of soda residue > apatite > lime > control, while the Cu concentration in both the 0.053–0.25 and <0.053 mm sized fractions followed the descending order of apatite > soda residue > lime > control. The Cd concentration in the 0.053–0.25 mm sized fraction followed the order: soda residue > lime > apatite > control, while in the <0.053 mm sized fraction, it followed the order lime > apatite > soda residue > control (Table 7). The highest mass loading concentrations of Cu and Cd were obtained in the 0.25–2 mm sized fraction (39.9–42.5% and 36.1–39.4%), followed by the 0.053–0.25 (26.7–32.4% and 32.9–39.2%), >2 (21.3–27.2% and 15.2–24.3%), and <0.053 mm (5.85–6.37% and 5.56–7.06%) sized fractions, respectively (Fig. 3).
Table 7 Concentrations of total Cu and Cd in different size aggregates of wet soil aggregates for different treatments. Different lowercase letters indicate significant differences between treatments after three years (n = 3, P < 0.05)
Treatment |
>2 mm |
0.25–2 mm |
0.053–0.25 mm |
<0.053 mm |
Cu (mg kg−1) |
Control |
406 ± 12.9a |
437 ± 37.5b |
241 ± 3.02a |
577 ± 23.4b |
Soda residue |
472 ± 5.41a |
560 ± 42.7a |
305 ± 8.82a |
663 ± 38.5a |
Apatite |
462 ± 16.3a |
582 ± 14.1a |
323 ± 25.3a |
671 ± 41.8a |
Lime |
425 ± 35.1a |
524 ± 50.6ab |
281 ± 35.7a |
640 ± 7.17ab |
![[thin space (1/6-em)]](https://www.rsc.org/images/entities/char_2009.gif) |
Cd (µg kg−1) |
Control |
286 ± 16.4c |
424 ± 30.4c |
254 ± 20.3a |
559 ± 42.2b |
Soda residue |
416 ± 43.4a |
512 ± 7.28ab |
373 ± 44.4a |
624 ± 28.4ab |
Apatite |
378 ± 38.2ab |
548 ± 46.7a |
344 ± 43.2a |
691 ± 39.7a |
Lime |
295 ± 32.3bc |
438 ± 39.2c |
361 ± 48.8a |
692 ± 37.3a |
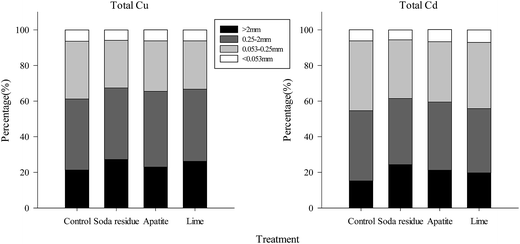 |
| Fig. 3 Total Cu and Cd mass loading values of four size fractions (mm). | |
Similar to the results of Xu,58 the present study found that the highest concentrations of heavy metals were found in the smallest aggregates (<0.053 mm), which might be due to the larger surface area of the small aggregates.59 The silt and clay were the main components in the <0.053 mm sized fraction, where metals might act as the binding agents for the clay-polyvalent metal–organic matter complexes.60 Thus, heavy metals could easily accumulate on their large surfaces by adsorption, forming chelating complexes with the organic-mineral colloidal particles in the finest fractions.61 Furthermore, the Cu and Cd concentrations in all the wet soil aggregate fractions of the treatments were slightly increased, which might be attributed to the higher content of total Cu and Cd in the treated whole soil than in the control (Table 2).
In addition, although the concentration of Cu and Cd in the <0.053 mm sized aggregates was the fundamental part, the mass loading levels were not predominant, which was consistent with the previous findings showing there was only 2.9–18.3% heavy metals loading in the <0.045 mm sized fraction.62 Meanwhile, the >2 and 0.25–2 mm sized fractions played important roles as Cu and Cd reservoirs in all the soils. Notably, the mass loading capacities of Cu and Cd in the >2 and 0.25–2 mm sized aggregates were markedly promoted after adding the amendments. This was due partly to the higher aggregate content of the >2 and 0.25–2 mm sized aggregates. Meanwhile, the content of heavy metals in the atmospheric deposition was high in this area, with some of the new input of heavy metals being first adsorbed on the surface of the micro-aggregates, and then the fine soil particles, leading to a high concentration of heavy metals and the formation of macro-aggregates under the action of SOC.63 In our combination remediation, the content of organic carbon in soil was raised because of the better growth of plants, which subsequently promoted the transition of soil from the micro-aggregates to macro-aggregates. This eventually led to the transfer of heavy metals from micro-aggregates into macro-aggregates. This may be the possible reason for the increased mass loading levels of Cu and Cd in the >0.25 mm sized aggregates following the chemical-plant treatments.
Moreover, previous studies have shown that SOC could affect the transportation and distribution of heavy metals in the soil through terrestrial ecosystems. In our present study, the concentration and mass loading of Cu in the wet aggregates were significantly positively correlated with SOC (rSOC–Cu concentration = 0.376**, rSOC–Cu mass loading = 0.558***, Table 8) which indicated that the SOC might control the distribution of Cu in the wet aggregates. However, the distribution of Cd in the aggregates was not significantly correlated with the SOC content. These results also agreed with those of Egli et al.,64 who found that Cu and Pb concentrations were significantly correlated with the labile organic carbon pools, and the content of SOC could affect the storage capacity and mobility of Cu and Pb in soils. Furthermore, we found a significant relationship between the size of the soil wet aggregates and both the concentrations and mass loading of Cu and Cd (Table 8); these results also agreed with those of Cui et al.41 Thus, we speculated that the concentration and distribution of Cu and Cd in the wet soil aggregate fractions were not only controlled by the size of the soil wet aggregates, but also by the SOC concentrations.
Table 8 The correlation between the concentration and mass loading of Cu and Cd and the concentrations of SOC and soil wet aggregates. Different lowercase letters indicate significant differences between treatments after three years (n = 48, ***P < 0.001, **P < 0.01 and *P < 0.05)
Factor |
|
Cu concentration |
Cu mass loading |
Cd concentration |
Cd mass loading |
SOC |
r |
0.376** |
0.558*** |
0.008 |
0.256 |
P |
0.009 |
<0.0001 |
0.956 |
0.078 |
Size of soil wet aggregates |
r |
−0.756*** |
0.718*** |
−0.805*** |
0.923*** |
P |
<0.0001 |
<0.0001 |
<0.0001 |
<0.0001 |
Conclusion
This study demonstrates the benefits of combining three kinds of amendments application and Elsholtzia splendens for improving soil quality during the process of heavy metals remediation of contaminated soils. The application of soda residue, apatite, or lime could increase the soil pH and decrease the available and exchangeable Cu and Cd concentrations in a smelter-impacted soil. In addition, after 3 years of the combined remediation, the concentration of SOC in the whole soil and in wet aggregates was dramatically improved, while the DR0.25 and WR0.25 also were significantly improved. The treatments increased the mass loading of Cu and Cd in the >0.25 mm sized aggregates, where the distribution of total Cu and Cd were not only significantly related to the size of soil wet aggregates, but also correlated with the SOC concentration in wet soil aggregates. Additionally, this study reminded us that the soil's physical indexes, such as soil aggregate stability, should be taken into consideration in the assessment of heavy metal contaminated soil remediation, especially for the purpose of improving the crop yield through remediation.
Conflicts of interest
There are no conflicts to declare.
Acknowledgements
The authors acknowledge the National Basic Research Program of China (2013CB934302), the National Science and Technology Support Plan (2015BAD05B01), and the National Natural Science Foundation of China (41571461, 41601340).
References
- Y. Yao, Nature, 2016, 533, 469 CrossRef CAS PubMed.
- A. Mahar, W. Ping, L. Ronghua and Z. Zhang, Pedosphere, 2015, 25, 555–568 CrossRef.
- R. Xiao, X. Sun, J. Wang, J. Feng, R. Li, Z. Zhang, J. J. Wang and A. Amjad, J. Anal. Appl. Pyrolysis, 2015, 113, 575–583 CrossRef CAS.
- Y. Sun, G. Sun, Y. Xu, L. Wang, X. Liang and D. Lin, Geoderma, 2013, 193, 149–155 CrossRef.
- N. Bolan, A. Kunhikrishnan, R. Thangarajan, J. Kumpiene, J. Park, T. Makino, M. B. Kirkham and K. Scheckel, J. Hazard. Mater., 2014, 266, 141–166 CrossRef CAS PubMed.
- V. Sheoran, A. Sheoran and P. Poonia, Crit. Rev. Environ. Sci. Technol., 2010, 41, 168–214 CrossRef.
- H. B. Cui, J. Zhou, Y. B. Si, J. D. Mao, Q. G. Zhao, G. D. Fang and J. N. Liang, J. Soils Sediments, 2014, 14, 1397–1406 CrossRef CAS.
- M. Vithanage, B. B. Dabrowska, A. B. Mukherjee, A. Sandhi and P. Bhattacharya, Environ. Chem. Lett., 2012, 10, 217–224 CrossRef CAS.
- X. Liang, Y. Xu, L. Wang, Y. Sun, D. Lin, Y. Sun, X. Qin and Q. Wan, Chemosphere, 2013, 90, 548–555 CrossRef CAS PubMed.
- A. Zanuzzi, A. Faz and J. Acosta, Environ. Earth Sci., 2013, 70, 2623–2632 CrossRef CAS.
- Z. Michálková, M. Komárek, H. Šillerová, L. Della Puppa, E. Joussein, F. Bordas, A. Vaněk, O. Vaněk and V. Ettler, J. Environ. Manage., 2014, 146, 226–234 CrossRef PubMed.
- G. Garau, P. Castaldi, L. Santona, P. Deiana and P. Melis, Geoderma, 2007, 142, 47–57 CrossRef CAS.
- E. Madejón, P. Madejón, P. Burgos, A. P. de Mora and F. Cabrera, J. Hazard. Mater., 2009, 162, 931–938 CrossRef PubMed.
- J. Fan, W. Ding and N. Ziadi, Commun. Soil Sci. Plant Anal., 2013, 44, 1224–1241 CrossRef CAS.
- H. R. Schulten and P. Leinweber, Biol. Fertil. Soils, 2000, 30, 399–432 CrossRef CAS.
- Q. Y. Wang, J. S. Liu, Y. Wang and H. W. Yu, J. Soils Sediments, 2015, 15, 1075–1082 CrossRef CAS.
- B. Huang, Z. Li, J. Huang, L. Guo, X. Nie, Y. Wang, Y. Zhang and G. Zeng, J. Hazard. Mater., 2014, 264, 176–183 CrossRef CAS PubMed.
- F. Madrid, M. Biasioli and F. Ajmone-Marsan, Arch. Environ. Contam. Toxicol., 2008, 55, 21–32 CrossRef CAS PubMed.
- J. Chen, F. He, X. Zhang, X. Sun, J. Zheng and J. Zheng, FEMS Microbiol. Ecol., 2014, 87, 164–181 CrossRef CAS PubMed.
- Z. Li, X. F. Chen and M. Liu, Sci. Agric. Sin., 2013, 46, 950–960 Search PubMed.
- Y. Lu, S. Song, R. Wang, Z. Liu, J. Meng, A. J. Sweetman, A. Jenkins, R. C. Ferrier, H. Li and W. Luo, Environ. Int., 2015, 77, 5–15 CrossRef CAS PubMed.
- P. Li, X. X. Wang, T. L. Zhang, D. M. Zhou and Y. Q. He, J. Environ. Sci., 2008, 20, 449–455 CrossRef CAS.
- X. Y. Zhang, L. D. Chen, B. J. Fu, Q. Li, X. Qi and Y. Ma, Journal of Resources and Ecology, 2006, 26, 3198–3204 CAS.
- E. Elliott, Soil Sci. Soc. Am. J., 1986, 50, 627–633 CrossRef.
- P. L. Yang, Y. P. Luo and Y. C. Shi, Chin. Sci. Bull., 1993, 38, 1896–1899 Search PubMed.
- A. Walkley and I. A. Black, Soil Sci., 1934, 37, 29–38 CrossRef CAS.
- F. T. Bingham, Methods of Soil Analysis. Part 2. Chemical and Microbiological Properties, 1982, pp. 431–447 Search PubMed.
- M. Pansu and J. Gautheyrou, Handbook of Soil Analysis: Mineralogical, Organic and Inorganic Methods, Springer Science & Business Media, 2007 Search PubMed.
- D. J. Walker, R. Clemente, A. Roig and M. P. Bernal, Environ. Pollut., 2003, 122, 303–312 CrossRef CAS PubMed.
- M. Jalali and N. H. Matin, Environ. Earth Sci., 2015, 73, 2047–2059 CrossRef CAS.
- V. Antoniadis, K. Damalidis and A. Dimirkou, J. Soils Sediments, 2012, 12, 396–401 CrossRef CAS.
- V. Antoniadis and E. Golia, Chemosphere, 2015, 138, 364–369 CrossRef CAS PubMed.
- M. Ashman, P. Hallett and P. Brookes, Soil Biol. Biochem., 2003, 35, 435–444 CrossRef CAS.
- S. DeGryze, J. Six, K. Paustian, S. J. Morris, E. A. Paul and R. Merckx, Global Change Biology, 2004, 10, 1120–1132 CrossRef.
- A. Gunina, I. Ryzhova, M. Dorodnikov and Y. Kuzyakov, Plant Soil, 2015, 387, 265–275 CrossRef CAS.
- M. Tao, J. Zhou, J. Liang, H. Cui, L. Xu and Z. Zhu, J. Agro-Environ. Sci., 2014, 33, 1328–1334 CAS.
- A. Fayiga and U. Saha, Soil Sediment Contam., 2016, 5, 7–23 Search PubMed.
- B. Seshadri, N. Bolan, H. Wijesekara, A. Kunhikrishnan, R. Thangarajan, F. Qi, R. Matheyarasu, C. Rocco, K. Mbene and R. Naidu, Geoderma, 2016, 270, 43–59 CrossRef CAS.
- P. Madejón, A. Pérez-de-Mora, P. Burgos, F. Cabrera, N. Lepp and E. Madejón, Geoderma, 2010, 159, 174–181 CrossRef.
- M. J. Tao, J. Zhou, J. N. Liang, H. B. Cui, L. Xu and Z. Q. Zhu, J. Agro-Environ. Sci., 2014, 33, 1328–1334 CAS.
- H. B. Cui, K. Q. Ma, Y. C. Fan, X. Peng, J. D. Mao, D. M. Zhou, Z. B. Zhang and J. Zhou, Environ. Sci. Pollut. Res., 2016, 10808–10818 CrossRef CAS PubMed.
- H. B. Cui, Y. C. Fan, L. Xu, J. Zhou, D. M. Zhou, J. D. Mao, G. D. Fang, L. Cang and Z. Q. Zhu, J. Soils Sediments, 2016, 16, 1498–1508 CrossRef CAS.
- A. P. Puga, L. C. A. Melo, C. A. de Abreu, A. R. Coscione and J. Paz-Ferreiro, Soil Tillage Res., 2016, 164, 25–33 CrossRef.
- P. Bhattacharya, A. C. Samal, T. Bhattacharya and S. C. Santra, Int. J. Exp. Res. Rev., 2016, 6, 39–49 Search PubMed.
- P. J. Mrdakovic, S. Meland, B. Salbu and L. Skipperud, Environ. Sci.: Processes Impacts, 2014, 16, 1124–1134 Search PubMed.
- S. C. Wong, X. D. Li, G. Zhang, S. H. Qi and Y. S. Min, Environ. Pollut., 2002, 119, 33–44 CrossRef CAS PubMed.
- L. Xu, H. B. Cui, X. B. Zheng, Z. Q. Zhu, J. N. Liang and J. Zhou, RSC Adv., 2016, 6, 103955–103965 RSC.
- G. Zeng, H. Wu, J. Liang, S. Guo, L. Huang, P. Xu, Y. Liu, Y. Yuan, X. He and Y. He, RSC Adv., 2015, 5, 34541–34548 RSC.
- R. Xiao, M. Zhang, X. Yao, Z. Ma, F. Yu and J. Bai, J. Soils Sediments, 2016, 16, 1–10 CrossRef.
- X. B. Zheng, J. B. Fan and J. Zhou, Agric. Sci. China, 2015, 48, 3201–3210 CAS.
- J. Li, X. Y. Yang, B. H. Sun and S. L. Zhang, Journal of Plant Nutrition and Fertilizer, 2014, 20, 346–354 Search PubMed.
- J. Six, C. Feller, K. Denef, S. M. Ogle, J. C. D. M. Sa and A. Albrecht, Agronomie, 2016, 22, 755–775 CrossRef.
- P. Puget, C. Chenu and J. Balesdent, Eur. J. Soil Sci., 2001, 51, 595–605 CrossRef.
- B. John, T. Yamashita, B. Ludwig and H. Flessa, Geoderma, 2005, 128, 63–79 CrossRef CAS.
- S. S. An, F. Darboux and M. Cheng, Geoderma, 2013, 210, 75–85 CrossRef.
- M. Hardie, B. Clothier, S. Bound, G. Oliver and D. Close, Plant Soil, 2014, 376, 347–361 CrossRef CAS.
- C. J. Bronick and R. Lal, Geoderma, 2005, 124, 3–22 CrossRef CAS.
- G. Xu, M. Liu and G. Li, J. Hazard. Mater., 2013, 260, 74–81 CrossRef CAS PubMed.
- R. Xiao, M. Zhang, X. Yao, Z. Ma, F. Yu and J. Bai, J. Soils Sediments, 2016, 16, 821–830 CrossRef CAS.
- L. Shen, A. Lo, X. Nguyen and N. Hankins, Sep. Purif. Technol., 2016, 159, 169–176 CrossRef CAS.
- H. Li, X. Han, F. Wang, Y. Qiao and B. Xing, Commun. Soil Sci. Plant Anal., 2007, 38, 1673–1690 CrossRef CAS.
- X. S. Wang, Y. Qin and Y. K. Chen, Environ. Geol., 2006, 50, 1061–1066 CrossRef CAS.
- R. A. Sutherland, Environ. Pollut., 2003, 121, 229–237 CrossRef CAS PubMed.
- M. Egli, G. Sartori, A. Mirabella, D. Giaccai, F. Favilli, D. Scherrer, R. Krebs and E. Delbos, Sci. Total Environ., 2010, 408, 931–946 CrossRef CAS PubMed.
|
This journal is © The Royal Society of Chemistry 2017 |