DOI:
10.1039/C7RA05433E
(Paper)
RSC Adv., 2017,
7, 39989-39996
Photodegradation of sulfadiazine catalyzed by p-benzoquinones and picric acid: application to charge transfer complexes†
Received
13th May 2017
, Accepted 7th August 2017
First published on 16th August 2017
Abstract
As the treatment of effluents containing the antibiotic drug sulfadiazine (SZ) is one of the challenging problems in the field of environmental chemistry, it is essential to determine the concentration of SZ by a rapid and accurate method and then find a suitable method to degrade the assayed products into harmless chemicals. The color of the charge transfer (CT) complexes developed from the reaction of SZ with 2,3-dichloro-5,6-dicyano-1,4-benzoquinone (DDQ), chloranilic acid (CHL) and picric acid (PA) was used to determine the concentration of SZ at 528, 510 and 410 nm, respectively. The Lambert–Beer's law is obeyed in the ranges of 6.80–68.06, 13.61–136.12 and 6.80–27.22 μg mL−1 for DDQ, CHL and PA complexes. The photolysis of SZ → DDQ in presence of sodium nitrite at 256 nm leads to faster degradation of SZ compared with the control experiments. This was simply spectrophotometrically followed by a decrease in the intensity of the CT band. The effect of some additives such as oxalic acid, and hematite nano particles was studied. For comparison, other π-acceptor reagents such as CHL and PA were used. About 80% of SZ is degraded in 45 min upon the illumination of SZ → DDQ at 256 nm, whereas 90 min is required in the case of CHL and PA to attain the same degradation limit.
Introduction
Sulfadiazine (4-amino-N-pyrimidin-2-yl-benzenesulfonamide, SZ, Scheme 1) is an effective sulfonamide drug used mainly in treatment of different types of bacterial infections.1 Sulfonamides are bacteriostatic rather than bactericidal. Their value lies in their ability to slow down or prevent bacterial multiplication in wounds or infected systems without appreciable toxicity to the body tissues. The metal complexes of SZ were found to be more effective and desirable drugs than the free drugs. Zn(II)–SZ was used to prevent bacterial infection in burned animals,2 while silvadene, Ag(I)–SZ, was applied in the case of the topical burns.3 Several analytical methods were used for determination of SZ in the pharmaceutical formulations and biological samples such as HPLC,4–6 LC-MS,7,8 fluorimetry,9 and UV-Vis.10–23
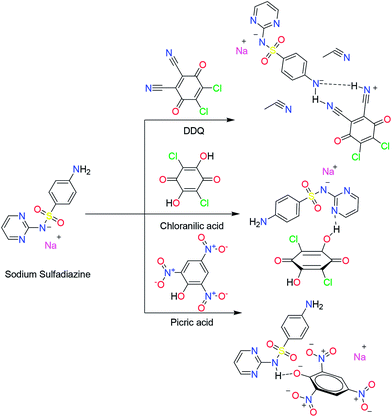 |
| Scheme 1 Structures of SZ, and its proposed charge transfer complexes, SZ → DDQ, SZ → CHL and SZ → PA. | |
Three main reactions were reported for SZ to give colored compounds capable of absorbing light in the visible range. First, diazotization of the primary amino group and coupling with several reagents such as 4-amino-5-hydroxy-naphthalene-2-7-disulfonic acid,10 resorcinol,11 3-amino phenol,12 thymol13 and 4-naphthol14 was one of the reported methods. The coupling of SZ with N,N-diethyl-p-phenylene diamine sulfate in presence of potassium iodate gave a red color product with λmax of 550 nm.15 Another colored compound has a λmax of 510 nm was formed from the reaction of SZ with promethazine–HCl in presence of N-bromosuccinimide.16 Second, the –NH2 group was also used to form some Schiff-base compounds with aldehydes such as 4-dimethylaminocinnamaldehyde17 with a detection limit of 0.4–4.8 μg mL−1. The determination of SZ using some acceptors through the formation of CT complexes, was the third method. This tool was rapidly assessed for its validity as a simple quantitative analytical method.18–23 The reported acceptors used were p-benzoquinone,18 tetrachlorobenzoquinone,19 tetra-cyano-ethylene,20 trinitro-benzene,21 safranin T22 and phenosafranine.23 The investigation of the molecular structures of some sulfonamides, including SZ, in the free acid form, with DDQ using IR, UV-Vis and 1H NMR spectroscopies has been reported elsewhere.24 The authors suggested the formation of H-bond between the –NH2 and C
O of DDQ that did not compare well with our findings from the complete proton transfer (discussed later).
In general, the water soluble NaSZ drug might be excreted from the humans and animal without the complete metabolization and biotransformation. In addition, the expired and unused SZ may be found also in the waste water coming from the households. Therefore, the treatment of effluents containing SZ is one of the challenging problems in the field of environmental chemistry. Most of the wastewater treatment methods do not include the decomposition of the SZ residues and thus the continuous release of these fragments may be terminated with the antibiotic resistance and toxicity effects. Among the different methods used to degrade the pollutants is the photocatalytic technique,25 which involves the creation of the highly reactive free radicals and safe by the formation of harmless chemicals such as water and carbon dioxide. The H2O2/KI assisted photo-decomposition of SZ in water at 365 nm was recently investigated, where the iodine radicals were proposed to be the driving force in the degradation process.26 The performance and the degradation pathways of SZ by UV/O3 have been studied.27 Fenton-reagent was also used in the degradation of SZ,28,29 compared with UV/H2O2 system28,30 and the relevance of the concentration and heterocyclic aromatic groups to the degradation kinetics was studied. The photochemical transformation of SZ in presence of different iron sources such as oxalate, citrate and nitrate was studied during the illumination with the sun-light.31 Unlike the Fenton tool, it was not necessary to add H2O2 to the degradation system, and the high degradation rate in case of the oxalate ion reflects the role of the anion in the degradation process. Immobilized TiO2 (ref. 32 and 33) and TiO2/FeCl3 (ref. 31, 34–36) systems were introduced as catalyst and the irradiation process was carried out at 365 nm.
The aim of the present work is to develop a sensitive, simple, rapid and accurate spectral method for the quantitative determination of SZ in the pure form and the pharmaceutical preparations via the formation of CT complexes with DDQ, CHL and PA (Scheme 1). The stoichiometry, formation constant and other thermodynamic parameters were also determined. Several analytical and spectral tools were used to characterize the solid CT complex with DDQ. Geometry optimization of SZ and its complexes was done by CAM-B3LYP/6-31G(d) method. Because of the oxidative power of DDQ and its role in the oxidation of alcohols37 and oxygenation of benzene,38 for the first time, to the best of our knowledge, the photocatalytic degradation of the environmental pollutants such as the expired and unused SZ were carried out with the aid of some π-acceptor systems as well as other additives such as Fe2O3, oxalic acid and sodium nitrite.
Experimental
Instruments
FT IR spectra were recorded as potassium bromide pellets using a Jasco FTIR 460 plus in the range of 4000 to 200 cm−1. 1H and 13C NMR spectra were run at 300 MHz in DMSO-d6 using a Varian-Oxford Mercury VX-300 NMR. Elemental microanalysis was performed using ElementerVario EL III. Electronic spectra were scanned on OPTIZEN POP automate spectrophotometer.
Synthesis
One mmol of NaSZ (272 mg) was added to one mmol of DDQ (227 mg) in 25 mL acetonitrile/H2O mixture (100
:
1), and then the reaction mixture was refluxed for 6 h, whereupon the solid CT complex was precipitated. Several trials were done to prepare the solid complexes of SZ → CHL and SZ → PA, but unfortunately these trials were failed.
• Data for DDQ (C8Cl2N2O2).39 IR (KBr, cm−1): 2241 (s, C
N), 1674 (s, C
O), 1553 (s, C
C), 1450 (s, C
C), 1267 (s, C–O), 890 (m, C–Cl). UV-Vis (acetonitrile, nm): 219, 264, 286, 352 and 484.
• Data for NaSZ (C10H9N4NaO2S). IR (KBr, cm−1): 3416 (m, NHass2), 1630 (m, C
N), 1292 (m, SOass2), 1131 (m, SOss2), 977 (w, S–N), 674 (w, C–S). 1H-NMR (300 MHz, DMSO-d6, δ): 8.06 (d, 2H, C–Hpym); 6.34 (t, 1H, C–Hpym); 7.48 (d, 2H, C–Hph); 6.46 (d, 2H, C–Hph); 5.30 (s, 2H, NH2).
• Data for SZ → DDQ (C18H9Cl2N6NaO4S·2CH3CN): color: dark brown. Elemental analysis (%): calc.: C 45.45, H 2.60, N 19.27, found C 45.28, H 2.44, N 18.38. IR (KBr, cm−1): 3438 (m, NHass), 3223 (m, NHss), 2211 (w, C
N), 1661 (m, C
O). 1H-NMR (300 MHz, DMSO-d6, δ): 10.96 (s, NH), 9.94 (s, NH), 8.50 (m, 2H), 8.04 (d, 1H), 7.91 (d, 1H), 7.62 (d, 1H), 7.26 (d, 1H), 7.00 (m, 1H). 13C-NMR (300 MHz, DMSO-d6, δ): 177.26 (C
O), 173.13 (C
O), 157.33, 157.15, 151.04, 143.27, 142.38, 140.22, 139.68, 136.29, 130.27, 128.64, 128.17, 127.00, 124.06, 116.27, 113.46, 112.67, 110.64, 83.44.
Quantum chemical calculations
Full geometry optimization of SZ, DDQ, CHL, PA and their charge transfer complexes were carried out by Gaussian 03 program package40 using density functional theory (DFT), hybrid exchange–correlation functional CAM-B3LYP with a long-range correction term41 and the split valence 6-31G(d) basis set. The complexes were characterized as local minima via harmonic frequency analysis.
Photometric measurements
5 × 10−3 mol L−1 stock solutions of SZ, (DDQ, CHL) and PA solutions were prepared in water, acetonitrile, and dioxane, respectively. The stoichiometry of the CT complexes in solution was determined by molar ratio42 and continuous variation43 methods. In the molar ratio method, the concentration of SZ was 2.5 × 10−4, 4 × 10−4, and 1 × 10−4 mol L−1 for DDQ, CHL and PA assay, respectively, while the acceptor is varied from 2.5 × 10−5 to 4.0 × 10−4, 1.0 × 10−5 to 6.0 × 10−4 and 5.0 × 10−5 to 1.5 × 10−4 mol L−1 in that order. The absorbance of SZ → DDQ and SZ → CHL complexes in acetonitrile was measured at 528 and 510 nm, while that of SZ → PA was monitored in dioxane at 410 nm. In the continuous variation method, the same concentration (5 × 10−3 M) of SZ and the acceptor was used. The plot of the absorbance versus the mole fraction of SZ showed a maximum at the molar ratio corresponding to the most stable formed species.
Analysis of dosage form
The analysis of SZ in Dermazin cream (30 mg, Medical Union Pharmaceutical company) was done by dissolving (500 mg) the cream in a concentrated H2SO4 (10 mL, 7 M). Filtration was carried out to remove the insoluble excipients. Then, the neutralization was achieved by adding 30 mL of concentrated sodium hydroxide (5 M), filtration was repeated. The filtrate was treated in the same way as the standard to get the corresponding CT complexes suitable to the spectral analysis.
General procedure
Aliquots of 5 × 10−3 mol L−1 of SZ solution were mixed with 0.5, 0.8 and 0.2 mL of DDQ, CHL and PA, respectively, in 10 mL volumetric flask and subsequent completed with the acetonitrile (DDQ and CHL) or dioxane (PA).
Photocatalytic degradation procedure
The photo degradation was carried out by illumination of SZ with a highly energetic UV hand lamp in presence and absence of the investigated π-acceptors. This was achieved by adding 0.5 mL of DDQ, 0.8 mL of CHL or 0.2 mL of PA from the stock solution (5 × 10−3 M) to an equivalent volume of SZ (5 × 10−3 M) in 10 mL measuring flask and then the volume was completed to the mark with acetonitrile or dioxan (10 mL). Then the sample was illuminated with a UV hand lamp, at two different wave lengths (256 and 365 nm), positioned perpendicular at a distance of 2 cm. The irradiation was interrupted in regular intervals to record the electronic spectrum until no further change was observed. The same experiment was repeated by addition of oxalic acid (2.0 × 10−4 M), suspended Fe2O3/oxalic acid (2.0 × 10−4 M) and sodium nitrite (1.0 × 10−4 to 4.0 × 10−4 M).
Results and discussion
Stoichiometry, stability and optimization conditions
The reaction between SZ and the π-acceptor systems (DDQ, CHL and PA) in solution afforded CT complexes with a ratio of 1
:
1. This stoichiometry was obtained by two different methods; molar ratio and continuous variation methods (Fig. S1 & S2†). The optimum conditions of the formation of the CT complexes and their stabilities were investigated through the study of several parameters such as the concentration of the donor and acceptors, time, pH, and temperature. The complexes were instantaneously formed and the absorbance of the developed color remained unchanged for one hour (Fig. S3†). The optimum pH ranges for the complete formation and high stability of CT complexes are 5.30–12.00, 4.50–6.20 and 5.00–11.20 for SZ → DDQ, SZ → CHL and SZ → PA, respectively (Fig. 1). The CT complexes are not recommended to be formed in the strongly acidic medium. A wide pH range was observed for both the DDQ and PA complexes formation, while it is limited for the CHL complex.
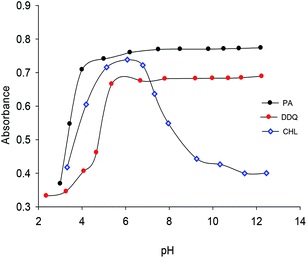 |
| Fig. 1 Effect of pH change on the stability of the SZ charge transfer complexes. | |
The formation constants and the molar absorptivity values of the complexes were determined by the modified Hildebrand method.44 The plot (Fig. S4†) of
values vs. CoA + CoD gives a straight line with a slope of 1/ε and intercept of
, where CoA and CoD are the initial concentrations of the acceptor and SZ, respectively, A is the absorbance of the detected CT band and l is the path-length. The values of kf (L mol−1) and ε (L mol−1 cm−1) are tabulated in Table 1. In comparison, SZ → DDQ has higher stability than the other complexes, which was reflected in the isolation of its solid complex. This may be attributed to the proton transfer and the strong H-bond created between the donor and the acceptor (discussed later). The oscillator strength (f), which is used to measure the integrated intensity of the CT band, was calculated39 and the values are given in Table 1. The highest f value of the CT band of SZ → DDQ complex indicates a high probability of the CT transition due to the strong interaction between the donor and acceptor. The electronic donating power of SZ was measured by calculating its ionization potential (Ip), which is related to the value of the wave number of the CT band (Ip (eV) = 5.76 + 1.52 × 10−4νCT). The lowest Ip value of SZ in SZ → DDQ suggests that SZ needs low energy to donate the electron density to DDQ molecule to form a stable complex. The standard free energy changes of complexation (ΔGo) of the investigated complexes were calculated. As shown in Table 1, the formation of SZ → DDQ is more spontaneous and favor than the other CT complexes.
Table 1 Spectrophotometric data of sulfadiazine CT complexes
Reagent |
DDQ |
Chloranilic acid |
Picric acid |
λmax (nm) |
528 |
510 |
410 |
kf (L mol−1) |
3.30 × 103 |
3.20 × 103 |
1.45 × 103 |
ε (L mol−1 cm−1) |
5.80 × 103 |
2.80 × 103 |
9.74 × 102 |
Ip (eV) |
8.66 |
8.76 |
9.49 |
f |
0.0095 |
0.0056 |
0.0020 |
μ (debye) |
1.80 |
0.78 |
0.42 |
ECT (eV) |
2.36 |
2.43 |
3.00 |
ΔGo (kJ mol−1 K−1) |
−14.50 |
−14.34 |
−12.37 |
Validation of Beer's law
A subsequent of the optimization of the reaction conditions, calibration curves were constructed for SZ using the π-acceptors (Fig. S5†). It was found that Beer's law is obeyed in the concentration range of 6.80–68.06, 13.61–136.12 and 6.80–27.22 μg mL−1 for DDQ, CHL and PA. The analytical parameters of the calibration curve, Sandell's sensitivity, correlation coefficient and relative standard deviation are given in Table 2. Therefore, PA is favored to use in the determination of low concentration of SZ, while CHL can be used for the higher concentration of SZ. The correlation coefficient values are close to the unity, which indicates that the validation of the proposed method in the applied concentration range. The intercept of the regression equation of the calibration curve is very small ((−0.045)–(0.016)), reflecting the corroboration of Beer's law. Highest molar absorptivity value was recorded for SZ → DDQ (3.3 × 103 L mol−1 cm−1) compared with the others. Detection limit is the smallest concentration of a solution of complex that can be detected with percent certainty. Based on the standard deviation of the replicate determination values under the same conditions and the slope of calibration curve, the detection limit (LOD) and the quantitation limit (LOQ) were calculated. The LOD and LOQ are found to be 0.88–7.57 and 2.66–22.94 μg mL−1, respectively.
Table 2 Analytical parameters of the developed spectrophotometric method
Parameters |
DDQ |
Chloranilic acid |
Picric acid |
λmax (nm) |
528 |
510 |
410 |
Linear range (μg mL−1) |
6.80–68.06 |
13.61–136.12 |
6.80–27.22 |
Molar absorptivity (L mol−1 cm−1) |
3.30 × 103 |
3.20 × 103 |
9.53 × 102 |
Sandell sensitivity (μg cm−2) |
0.103 |
0.24 |
0.0279 |
Relative standard deviation (%) |
1.78 |
1.67 |
1.03 |
Detection limit (μg mL−1) |
3.61 |
7.57 |
0.88 |
Quantification limit (μg mL−1) |
10.95 |
22.94 |
2.66 |
Slope |
0.0096 |
0.00415 |
0.0357 |
Intercept |
−0.044 |
+0.016 |
−0.045 |
Correlation coefficient |
0.997 |
0.996 |
0.996 |
The selectivity of the developed method was studied by evaluating the interfering effect of some excipients used in the pharmaceutical preparations of SZ such as lactose, fructose, glucose, starch and sodium citrate. The obtained data (Table S1†) showed that the recoveries of SZ are in the range of 99.3–101.0% with no interference from these excipients. The repeatability and reproducibility of the developed method have been obtained (Table S2†) from the calculation of the intra-day and inter-day precision and accuracy by carrying out the experiment five times at three different concentrations.
The ruggedness and robustness of the proposed method were assessed by another researcher and studying the influence of the small variation of the experimental variables. For example, the concentration of the acceptor was changed up and down by about 0.02 M and the reaction time on the same manner by 2.0 min. In these experiments, one experimental parameter was changed, while the other parameters were kept unchanged. The results (Table S3†) did not show any considerable statistical difference suggesting that the applied method is robust and rugged.
The unique properties such as the simplicity, sensitivity, repeatability and reproducibility are very important for the routine quality control analysis of SZ especially it doesn't require dull extraction, heating at high temperature and long time for the completion of the reaction. As shown in Table S4,† the average recoveries for the determination of SZ in Dermazin drug are in the range of 99.2–100.2%, which reflects the high accuracy and precision of the proposed method as given by their low RSD% values.
Spectral characterization of solid CT complex
To clarify the coordination sites of SZ involved in the formation of SZ → DDQ, the IR spectra of SZ and DDQ were compared with that of SZ → DDQ. The IR bands at 2241 (ν(C
N)), 1553 (ν(C
C)) and 890 cm−1 (ν(C–Cl)) are the characteristic modes39 of DDQ. The NaSZ spectrum exhibits the asymmetric mode of the –NH2 group at 3416 cm−1, while the symmetric mode is overlapped. The bands observed at 1630, 1292 and 1131 cm−1 are attributed to ν(C
N)py, ν(SOass2) and ν(SOss2). In CT complex, the most noteworthy remark is the disappearance of the stretching mode of the –NH2 group and grown of two broad bands at 3438 and 3223 cm−1 because of the formation of two –NH groups. Furthermore, the ν(C
N) mode is shifted to the lower wave number, 2211 cm−1 via the proton transfer process and presence of double bond character to some extent (Scheme 1). An additional band assigned to ν(C
O) of DDQ is observed at 1661 cm−1 in the spectrum of the CT complex.
The 1H NMR spectrum of NaSZ in DMSO displays a singlet broad signal at δ 5.30 ppm with integration of two protons allocated to the –NH2 group. The aromatic protons of the pyrimidine ring give rise to one triplet and one doublet signals at δ 6.34 and 8.06 ppm. The other doublet signals at δ 6.46 and 7.48 ppm are assigned to the phenyl ring. In complex, the vanishing of the –NH2 signal and appearance of two singlet signals at δ 9.94 and 10.97 ppm with the same integration value could be taken as an evidence for the proton transfer from –NH2 group to DDQ moiety. In addition, the symmetry of the aromatic signals of the phenyl ring is lost, where four doublet signals are observed at δ 7.26, 7.62, 7.91 and 8.04 ppm. The pyrimidine ring give rises to two signals at δ 8.50 and δ 7.00 ppm. The addition of picric acid to NaSZ was accompanied by a proton transfer, where an additional singlet signal (δ 11.21 ppm) was observed.
Quantum chemical calculations
The ground state geometry optimization of the mono-negatively CT complexes was carried out at CAM-B3LYP/6-31G(d) level of theory. The geometries were characterized as local minima via the analysis of the harmonic frequencies. A view of the molecular structures, atomic numbering as well as hydrogen bond interactions between the donor and acceptor molecules is shown in Fig. 2. The bond lengths and angles are given in Tables S5–S7.† As shown in Fig. 2, the optimized structure of SZ → DDQ is characterized by two H-bonds, N(39)⋯H–N(24) (1.94182 Å, 167.6°) and N(39)⋯H–C(19) (2.35261 Å, 136.9°), as a result of proton transfer from –NH2 to C
N group. An increase in the bond lengths of C(30)–C(31), C(36)–N(39) and C(37)–N(38) by 0.11723, 0.01105 and 0.06278 Å is observed upon the proton transfer and H-bond interactions. Similar, the C(19)–C(21) and C(17)–C(21) bonds of SZ are 0.06242 and 0.05758 Å lengthened upon the CT formation. Alternatively, the structure of SZ → CHL is stabilized by three H-bonds, which are supportive for the CT process. As the H-bond interaction approaches more linearity as it become stronger.45 The interaction of pyrimidine N with the phenolic group is the strongest one with a distance of 1.57122 Å and angle of 164.5° compared with the other H-bonds, O(34)⋯H⋯C(2) (2.88785 Å, 160.1°) and Cl(38)⋯H–C(2) (2.98315 Å, 132.5°).46 In comparison, the C(29)–C(30) bond lengthens by 0.01084 Å, while the C(30)–O(36) bond shortens by 0.02451 Å as a result of the pyrimidine–phenolic interaction. The other interactions result in an increase in the bond lengths of C(31)–O(34) and C(32)–Cl(38) bonds by 0.00442 and 0.00616 Å upon the formation of SZ → CHL complex. Five H-bond interactions and proton transfer from the acceptor (phenolic group) to the donor (pyrimidine ring) support the stability of SZ → PA (Fig. 2). The phenyl ring participates with one nitro group in two H-bonds with distances of 2.394 (O(44)⋯H–C(2)) and 3.124 Å (O(34)⋯H–C(1)). Followed the proton transfer process to the pyrimidine ring, the NH proton is affected by two H-bonds, 1.929 (O(33)⋯H–N(23)) and 2.329 Å (O(44)⋯H–N(23)). The last H-bond is the strongest H-bond interaction and is created between O(40)NO and pyrimidine ring, O(40)⋯H–C(20) with a distance of 2.401 Å and angle 176.3°.
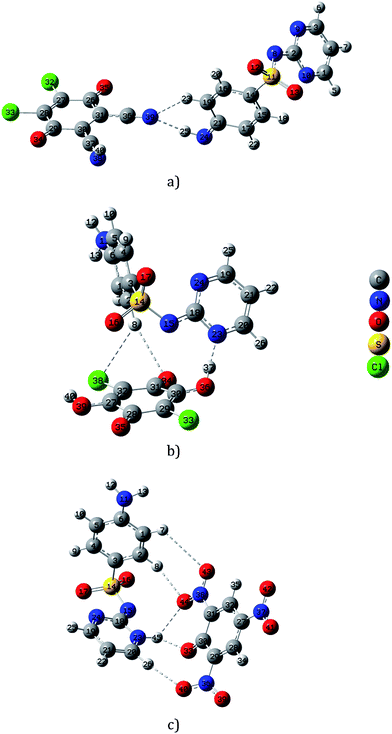 |
| Fig. 2 Local minimum structures of the mono-negatively SZ–CT complexes (a) SZ → DDQ, (b) SZ → CHL, and (c) SZ → PA, calculated by CAM-B3LYP/6-31G(d) method. | |
Photo-catalytic degradation
Recently, an increase consideration has been dedicated to the pharmaceutical compounds as a class of environmental pollutants.47,48 Despite the target of these compounds is to perform a biological action on human beings, may also affect other living organisms in a unexpectable way. Owing to their toxicity, sulfonamides are now replaced by other chemotherapeutics such as quinolones.49 The effectiveness of DDQ to be used as an oxidant for the oxidation of different types of the organic compounds such as alcohols37,38 encouraged us to study the photodegradation process of SZ in presence of different π-acceptor systems. As previously discussed, SZ forms stable colored complexes with some π-acceptors and it is easy to spectrophotometrically follow the degradation process. Illumination of the acetonitrile solution of SZ, as a control experiment at either 256 or 365 nm was followed by monitoring the change of the absorbance value at 239 nm. The degradation efficiency [R = 100 × ((At − A0)/A0)] was determined, where A0 and At are the initial absorbance and that obtained after the illumination time (t). About 19.0% and 9.0% of SZ was decomposed at 256 and 365 nm within 3 h (Fig. S6†). Thus, the degradation of SZ is hardly to achieve in the absence of an oxidant. Introduction of a π-acceptor to the solution of SZ develops a colored solution. This color disappears upon the exposure to UV-light, 365 nm. Illumination of SZ → DDQ (2.5 × 10−4 M), SZ → CHL (4.0 × 10−4 M) and SZ → PA (1.0 × 10−4 M) systems for about 3 h results in degradation of about 85%, 49% and 8% in that order (Fig. S7†). DDQ has been shown to act as an efficient photo-oxidant.
To be able to decrease the degradation time, the combination of Fe2O3 nanoparticles and oxalic acid has been tested with the systems of CT complexes. The irradiation of the ferrioxalate complexes31,34–36 resulted in formation of hydroxyl radicals, which may be able to accelerate the decomposition process. By using oxalic acid alone, about 77.5% and 41% of SZ were decomposed upon the illumination of SZ → DDQ and SZ → CHL systems at 365 nm for 90 min, while the degradation of SZ → PA was hardly to proceed. The degradation efficiency is decreased by adding Fe2O3 to a system of SZ + DDQ + oxalic acid, while a highly resistance towards the degradation was observed with CHL and PA acceptors that was shown in Fig. 3. This may be attributed to complex formation and stabilization of the sulfonamide group.
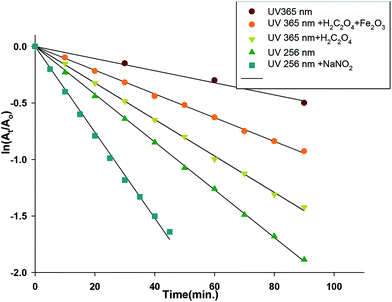 |
| Fig. 3 Linear relationship ln(At/Ao) with the illumination time (min) of SZ → DDQ photodegradation under different conditions. | |
High energetic UV lamp (256 nm) was used for the illumination process to increase the efficiency and rate of the degradation process. The results showed that about 78% (SZ → DDQ), 62% (SZ → CHL) and 75% (SZ → PA) of SZ were decomposed during 90 min. Then sodium nitrite (1.0 × 10−4 to 4.0 × 10−4 M) was tested in combination with the same light source. The decomposition rate was twofold accelerated in case of DDQ, where about 80% of SZ was degraded in 45 min. However, the degradation percent remains nearly the same for the other two systems, as 69% (SZ → CHL) and 76% (SZ → PA) of SZ was decomposed in 90 min. The photodegradation of the CT complexes was found to be a first order reaction. By plotting ln(At/A0) vs. time, a linear relation was obtained with a slope corresponding to the rate constant (Table 3). Therefore, by using DDQ as an acceptor, sodium nitrite and illumination of the reaction mixture at 256 nm, the rate and time of the degradation process are improved. The illumination process of SZ → DDQ/NaNO2 system at 256 nm was followed by solution 1H NMR studies in CD3–CN (Fig. S8†). Exclusion of SO2 gas was monitored, and detected by potassium dichromate test subsequent by formation of a variety of insoluble products, including coupling of the pyrimidine and aminobenzene rings. The broadness of the NMR signals may be taken as an indication of the creation of free radicals, which should be the motive of the degradation process.
Table 3 The rate constant, t1/2 and the degradation efficiency of the degradation of SZ under different conditions
Conditionsa |
Acceptor |
The degradation efficiency% |
Duration time |
t1/2 (min) |
k (min−1) |
0.5 mL of DDQ, 0.8 mL of CHL or 0.2 mL of PA from the stock solution (5 × 10−3 M) was mixed with the equivalent volume of SZ (5 × 10−3 M) in 10 mL measuring flask and then the volume was completed to 10 mL. The same experiment was repeated by addition of oxalic acid (2.0 × 10−4 M), suspended Fe2O3/oxalic acid (2.0 × 10−4 M) and sodium nitrite (1.0 × 10−4 to 4.0 × 10−4 M). |
UV light (365 nm) |
DDQ |
85 |
180 |
126 |
5.5 × 10−3 |
CHL |
49 |
177 |
3.90 × 10−3 |
PA |
8.0 |
1443 |
4.80 × 10−4 |
UV 365 nm + H2C2O4 |
DDQ |
77.5 |
90.0 |
66.5 |
1.04 × 10−2 |
CHL |
41.0 |
88.0 |
7.90 × 10−3 |
UV 365 nm + H2C2O4 + Fe2O3 |
DDQ |
77.0 |
90.0 |
48.0 |
1.60 × 10−2 |
UV light (256 nm) |
DDQ |
78.0 |
90.0 |
43.3 |
2.09 × 10−2 |
CHL |
62.0 |
56.0 |
1.24 × 10−2 |
PA |
75.0 |
43.0 |
1.63 × 10−2 |
UV 256 nm + NaNO2 |
DDQ |
80.0 |
45.0 |
18.7 |
3.70 × 10−2 |
CHL |
69.0 |
90.0 |
58.0 |
1.20 × 10−2 |
PA |
76.0 |
47.0 |
1.03 × 10−2 |
Conclusion
The determination of sulfadiazine has been spectrophotometrically performed through the formation of three charge transfer complexes with 2,3-dichloro-5,6-dicyano-1,4-benzoquinone (DDQ), chloranilic acid (CHL) and picric acid (PA). The Lambert–Beer's law was obeyed in the ranges of 6.80–68.06, 13.61–136.12 and 6.80–27.22 μg mL−1 for DDQ, CHL and PA complexes. The CT complexes are not recommended to be formed in the strongly acidic medium. DDQ and PA are favored to use in the determination of the low concentration of SZ, while CHL can be used for the high concentration of SZ. The results did not show any considerable statistical difference suggesting that the applied method is robust and rugged. The IR and NMR data of SZ → DDQ revealed charge transfer process accompanied by proton transfer from –NH2 of SZ to C
N of DDQ. Density functional theory calculations indicated that the stabilization of the CT complexes is attributed to presence of several H-bond interactions. Owing to the oxidative power of DDQ, for the first time the π-acceptors were investigated in the photodegradation process of the investigated drug. The degradation of SZ is hardly to achieve in the absence of an oxidant or in presence of iron(III) oxide. Illumination of SZ → DDQ system at 365 nm for about 3 h resulted in degradation of about 85%. By using 256 nm as a light source, the time was decreased to 90 min. The decomposition rate was twofold accelerated at 256 nm by adding sodium nitrite to the reaction mixture.
Conflicts of interest
There are no conflicts to declare.
Acknowledgements
Ahmed M. Mansour thanks the Alexander von Humboldt foundation for a postdoctoral fellowship.
References
- A. M. Romero, C. G. Benito and J. M. Calatayud, Anal. Chim. Acta, 1995, 308, 451–456 CrossRef.
-
(a) N. C. Baenziger, S. L. Modak and C. L. Fox, Acta Crystallogr., Sect. C, 1983, 39, 1620 CrossRef;
(b) C. J. Brown, D. S. Cook and L. Sengier, Acta Crystallogr., Sect. C, 1985, 41, 718 CrossRef.
-
(a) N. C. Baenziger and A. W. Struss, Inorg. Chem., 1976, 15, 1807 CrossRef CAS;
(b) D. S. Cook and M. F. Turner, J. Chem. Soc., Perkin Trans. 2, 1975, 1021 RSC.
- A. V. Herrera, J. Hernandez-Borges, T. M. Borges-Miquel and M. A. Rodríguez-Delgado, J. Pharm. Biomed. Anal., 2013, 75, 130 CrossRef PubMed.
- C. Lin and S. Huang, Anal. Chim. Acta, 2008, 612(1), 37 CrossRef CAS PubMed.
- K. E. Maudens, G. Zhang and W. E. Lambert, J. Chromatogr. A, 2004, 1047(1), 85 CrossRef CAS PubMed.
- S. De-Baere, K. Baert, S. Croubels, P. De-Backer, J. De-Busser and K. De-Wasch, Analyst, 2000, 125(3), 409 RSC.
- T. A. M. Msagati and M. M. Mathew, Talanta, 2004, 64(1), 87 CrossRef CAS PubMed.
- R. Diez and M. C. O. Sarabia, Anal. Chim. Acta, 2007, 585(2), 350 CrossRef CAS PubMed.
- N. D. Dinesh, P. Nagaraja and K. S. Rangappa, Proc. Natl. Acad. Sci., India, Sect. A, 2002, 72(3), 231 CAS.
- P. Nagaraja, K. R. Sunitha, R. A. Vasantha and H. S. Yathirajan, Indian J. Pharm. Sci., 2002, 64(4), 391 CAS.
- P. Nagaraja, H. S. Yathirajan, C. R. Raju, R. A. Vasantha, P. Nagendra and M. S. Hemantha-Kumar, Farmaco, 2003, 58(12), 1295 CrossRef CAS PubMed.
- S. A. Dhahir and A. H. Mhemeed, Asian J. Chem., 2012, 24(7), 3053 CAS.
- P. Nagaraja, H. R. Arun-Kumar, S. D. Naik, A. K. Shrestha and A. Shivakumar, Indian Pharm., 2007, 6(64), 76 CAS.
- P. Nagaraja, A. K. Shrestha, A. Shivakumar and A. K. Gowda, Acta Pharm., 2010, 60(2), 217 CrossRef CAS PubMed.
- N. D. Dinesh, P. Nagaraja and K. S. Rangappa, J. Indian Chem. Soc., 2003, 80(10), 934 CAS.
- S. Raghuveer, I. R. K. Raju, D. K. Vatsa and C. M. R. Srivastava, Indian Drugs, 1993, 30(4), 191 CAS.
- A. I. Mohamed, H. F. Askal and G. A. Saleh, J. Pharm. Biomed. Anal., 1991, 9(7), 531 CrossRef CAS PubMed.
- X. Zhou, L. Feng, W. Zhou, G. Zhao, J. Yang and N. Zhang, Fenxi Huaxue, 1999, 27(2), 244 CAS.
- A. M. Nour El-Din, Arch. Pharm., 1986, 319(2), 143 CrossRef CAS.
- Y. M. Issa, H. B. Hassib, A. L. El-Ansary and S. Z. Henein, ACH - Models Chem., 1994, 131(5), 607 CAS.
- A. S. Al-Attas, J. Saudi Chem. Soc., 2002, 6(2), 201 CAS.
- A. S. Al-Attas, Saudi Pharm. J., 2003, 11(3), 141 CAS.
- A. L. El-Ansary, H. B. Hassib, Y. M. Issa and S. Z. Henein, Egypt J. Chem., 1997, 40(3), 201 CAS.
-
(a) A. M. Mansour, RSC Adv., 2015, 5, 62052 RSC;
(b) A. M. Mansour, E. M. El-Bakry and N. T. Abdel-Ghani, J. Photochem. Photobiol. A. Chem., 2016, 327, 21 CrossRef CAS.
- H. Wei, X. Yang, K. Li and T. Min, Huanjing Kexue Xuebao, 2016, 36(5), 1697 CAS.
- W. Guo, Z. Yang, J. Du, R. Yin, X. Zhou, S. Jin and N. Ren, RSC Adv., 2016, 6, 57138 RSC.
- A. P. S. Batista, F. C. C. Pires and A. C. S. C. Teixeira, J. Photochem. Photobiol. A. Chem., 2014, 286, 40 CrossRef CAS.
- A. P. S. Batista and R. F. P. Nogueira, J. Photochem. Photobiol. A. Chem., 2012, 232, 8 CrossRef CAS.
- N. Lemanska-Malinowska, E. Felis and J. Surmacz-Gorska, Arch. Environ. Prot., 2013, 39(3), 79 CAS.
- A. P. S. Batista, B. A. Cottrell and R. F. P. Nogueira, J. Photochem. Photobiol. A. Chem., 2014, 274, 50 CrossRef CAS.
- J. Zhang, M. Xia, H. Wei, G. Wang and R. Han, Anquan Yu Huanjing Xuebao, 2014, 14(6), 143 CAS.
- W. Baran, J. Sochacka and W. Wardas, Chemosphere, 2006, 65(8), 1295 CrossRef CAS PubMed.
- E. Adamek, W. Baran, J. Ziemianska and A. Sobczak, Appl. Catal., B, 2012, 126, 29 CrossRef CAS.
- J. Zhang, D. Fu, L. Yang, L. Deng and Y. Sun, J. Southeast Univ., 2011, 27(3), 275 CAS.
- W. Baran, E. Adamek, A. Sobczak and A. Makowski, Appl. Catal., B, 2009, 90(3–4), 516 CrossRef CAS.
- L. Wang, J. Li, H. Yang, Y. Lv and S. Gao, J. Org. Chem., 2012, 77, 790 CrossRef CAS PubMed.
- K. Ohkubo, A. Fujimoto and S. Fukuzumi, J. Am. Chem. Soc., 2013, 135, 5368 CrossRef CAS PubMed.
- O. R. Shehab and A. M. Mansour, J. Mol. Struct., 2015, 1093, 186 CrossRef CAS.
- M. J. Frisch, G. W. Trucks, H. B. Schlegel, G. E. Scuseria, M. A. Robb, J. R. Cheeseman, V. G. Zakrzewski, J. A. Montgomery, R. E. Stratmann, J. C. Burant, S. Dapprich, J. M. Millam, A. D. Daniels, K. N. Kudin, M. C. Strain, O. Farkas, J. Tomasi, V. Barone, M. Cossi, R. Cammi, B. Mennucci, C. Pomelli, C. Adamo, S. Clifford, J. Ochterski, G. A. Petersson, P. Y. Ayala, Q. Cui, K. Morokuma, D. K. Malick, A. D. Rabuck, K. Raghavachari, J. B. Foresman, J. Cioslowski, J. V. Ortiz, A. G. Baboul, B. B. Stefanov, G. Liu, A. Liashenko, P. Piskorz, I. Komaromi, R. Gomperts, R. L. Martin, D. J. Fox, T. Keith, M. A. Al-Laham, C. Y. Peng, A. Nanayakkara, C. Gonzalez, M. Challacombe, P. M. W. Gill, B. G. Johnson, W. Chen, M. W. Wong, J. L. Andres, M. Head-Gordon, E. S. Replogle and J. A. Pople, GAUSSIAN 03 (Revision A.9), Gaussian, Inc., Pittsburgh, 2003 Search PubMed.
- T. Yanai, D. Tew and N. Handy, Chem. Phys. Lett., 2004, 393, 51–57 CrossRef CAS.
- J. H. Yoe and A. L. Jones, Ind. Eng. Chem., Anal. Ed., 1944, 16, 14 Search PubMed.
- W. C. Vosburgh and G. R. Cooper, J. Am. Chem. Soc., 1941, 63, 437 CrossRef CAS.
- H. A. Benesi and J. H. Hildebrand, J. Am. Chem. Soc., 1949, 71, 2703 CrossRef CAS.
- A. M. Mansour, Dalton Trans., 2015, 43, 15950 RSC.
- P. Malla, D. Marion, E. V. Ivanova and H. M. Muchall, J. Mol. Struct., 2010, 979, 101 CrossRef CAS.
- D. Kolpin, E. Furlong, M. Meyer, E. Thurman, S. Zaugg, L. Barber and H. Buxton, Environ. Sci. Technol., 2002, 36, 1202 CrossRef CAS PubMed.
- M. L. Richardson and J. M. Bowron, J. Pharm. Pharmacol., 1985, 37, 1 CrossRef CAS PubMed.
- A. M. Mansour, Inorg. Chim. Acta, 2013, 394, 436 CrossRef CAS.
Footnote |
† Electronic supplementary information (ESI) available. See DOI: 10.1039/c7ra05433e |
|
This journal is © The Royal Society of Chemistry 2017 |
Click here to see how this site uses Cookies. View our privacy policy here.