DOI:
10.1039/C7RA04943A
(Paper)
RSC Adv., 2017,
7, 38243-38250
Physicochemical properties and in vitro bioaccessibility of lutein loaded emulsions stabilized by corn fiber gums
Received
2nd May 2017
, Accepted 11th July 2017
First published on 3rd August 2017
Abstract
Lutein is a natural colorant and functional ingredient with many health-promoting bioactivities. However, its use in functional foods is limited due to its low water-solubility and chemical instability. The objectives of this study were to investigate the physicochemical properties and in vitro bioaccessibilities of lutein loaded emulsions stabilized by corn fiber gums (CFG1 and CFG2). The physicochemical properties and stabilities of the emulsions were evaluated by droplet size and distribution, ζ-potential, viscosity, visual observation of phase separation, and lutein retention during storage. The lutein bioaccessibilities of the emulsions were measured after digestion in a simulated gastrointestinal tract (SGT). The results showed that CFG2 had better emulsifying capacity and stability than CFG1. Compared to lutein dispersed in corn oil, the chemical stability and in vitro bioaccessibility of lutein in the emulsions were significantly increased through emulsification. These results suggest that corn fiber gum is a promising natural emulsifier, which has potential to fabricate emulsions for delivering lutein or other lipophilic bioactive compounds in functional foods.
Introduction
Lutein is a natural coloring agent widely existing in many biological materials including yellow corn, egg yolk and marigold flowers. Lutein, belonging to the xanthophyll class of carotenoids, possesses many health-promoting bioactivities, such as lowering of the risk of age-related eye diseases and antioxidant activities.1–3 The use of lutein as a functional ingredient is currently limited due to its relatively low and variable oral bioavailability.1,4 Poor bioavailability is attributed to its chemical instability, low water-solubility and high melting point.5 Overcoming these limitations will broaden the application of lutein in functional foods and pharmaceutical fields. Oil-in-water (O/W) emulsion, consisting of small lipid droplets in an aqueous system, is a promising delivery system for improving the bioavailability of lipophilic bioactive compounds.4,6 For the emulsions, the lipid phase breaks down to form mixed micelles in the gastrointestinal tract. The lipid-dissolved lipophilic compounds are then solubilized in the mixed micelles, and thus increase their bioavailability.7,8 In addition, emulsion-based delivery systems have been indicated to improve the physical and chemical stability of lutein.4,9 Nowadays, gum arabic (GA), one of the most commonly used emulsifiers, is extensively used to prepare O/W emulsions due to its good emulsifying properties, low viscosity, and high water solubility.10,11 However, the utilization of GA has been restrained due to high cost and variable sources in the food industry.11,12 It was reported that increased utilization of natural emulsifiers in food products can result in a healthier and more sustainable food supply.13 Therefore, there is a need to find alternative natural polysaccharide-based emulsifiers with potential application within the food industry.
Corn is one of the most important economic crops in the world. According to the 2017 USDA's report, the amount of corn production in China will increase to more than two million tons. Corn fiber is considered as an abundant low-value by-product in the process of corn wet- and/or dry-milling. Corn fiber contains the most fibrous portions of corn kernel pericarp and endosperm tissues. Corn fiber gum (CFG) could be easily isolated from de-starched and de-oiled corn fiber by the alkaline hydrogen peroxide extraction, which was proven to be a facile, reproducible, and economic method.14,15 CFG has been demonstrated to have good thickening, emulsifying, and film-forming properties in a number of previous studies.15–18 Therefore, CFG has potential to be an ideal natural emulsifier for commercial utilization within the food industry.13 However, little work has been reported on the application of CFG in designing emulsion-based delivery systems for nutraceuticals, such as lutein.
The aim of this work was therefore to study the physicochemical property and in vitro bioaccessibility of lutein loaded emulsions stabilized by corn fiber gum (CFG). Two CFG fractions (CFG1 and CFG2) were extracted from de-oiled and de-starched corn fiber. The physicochemical properties and stabilities of lutein emulsions stabilized by CFG1, CFG2, and GA were comparatively evaluated by droplet size and distribution, zeta-potential, viscosity, visual observation of phase separation, and lutein retention during storage. Furthermore, in vitro bioaccessibilities of lutein in the emulsions were measured in a simulated gastrointestinal tract (SGT).
Experimental
Materials and chemicals
Corn fiber was kindly provided by Inner Mongolia Yuwang Biological Technology Co. Ltd (Tongliao, Inner Mongolia, China). This source of corn fiber used in this study has good reliability and no obvious batch-to-batch variations according to the manufacturer's instruction. The corn fiber was ground in a cutting mill and passed through a 20-mesh screen to obtain fine powder. Corn oil (Wilmar Trading Co. China) was purchased from a local store (Guangzhou, China) and used without further purification. Food grade α-amylase (20
000 U mL−1) was kindly provided by Novozymes Biotechnology Co. Ltd (Wuxi, China). Gum arabic (GA) (containing around 16.2% moisture, 69.7% polysaccharide, 1.12% protein, and 0.3% minerals) was purchased from Cargill Co. (Minneapolis, USA). Trifluoroacetic acid (TFA), monosaccharide standards (arabinose; galactose; glucose; xylose; mannose) and uronic acid (galacturonic acid; glucuronic acid) were purchased from Aladdin Chemistry Co. (Shanghai, China). 2,2-diphenyl-1-picrylhydrazyl (DPPH), gastric lipase (105 units per g), trypsin (300 units per mg), pepsin (3000 units per g), and bile salts were purchased from Sigma-Aldrich Chemical Co. (St. Louis, MO, USA). All other chemicals and solvents were of analytical grade.
Extraction of corn fiber gum (CFG)
CFG was isolated from de-oiled and de-starched corn fiber by the alkaline hydrogen peroxide method with modifications.14 Corn fiber (50.0 g) was mechanically stirred into water (0.5 L) containing NaOH (2 g) and Ca(OH)2 (1.9 g). The mixture was boiled for 1 h and then centrifuged at 6000 × g for 20 min and first supernatant was collected. The residue was resuspended in 400 mL of distilled water, boiling for 5 min and then centrifuged at 6000 × g for 20 min. The supernatant was collected and combined with first supernatant. Then 5.1 g of 30% H2O2 was added to the combined extract (0.7 L, pH 11.3) and the pH was readjusted to 11.5 by adding NaOH solution (25%) with stirring at room temperature for 2 h. The pH was adjusted to 4.0–4.5 by adding concentrated HCl to precipitate hemicellulose A, which was collected by centrifugation at 10
000 × g for 30 min. Two volumes of ethanol were added to the supernatant to precipitate the major arabinoxylan fraction. Then the precipitate was collected by centrifugation at 5000 × g for 10 min and lyophilized to obtain CFG-1. The residue left after alkali extraction was further extracted with alkaline H2O2 solution at 100 °C with stirring for 1.5 h. The supernatant was separated by centrifugation at 6000 × g for 15 min and then adjusted to pH 4.0–4.5. The precipitate was removed by centrifugation at 10
000 × g for 30 min. The CFG-2 was obtained from the supernatant by precipitating it with 2-fold volumes of ethanol, collected and lyophilized.
Chemical composition and structure analysis
The carbohydrate content was determined by the phenol–sulfuric acid colorimetric method.19 The protein content was determined by the Lowry's method.20 The ferulic acid content was determined by a reported method.21
The molecular weight (Mw) distribution of the sample was measured using a reported method in our lab.22 In brief, the experiment was performed on an Agilent 1260 instrument (Agilent, USA) equipped with an Agilent 1260 Refractive Index Detector (Agilent Co., USA) and TSK-G5000 (7.8 × 300 mm i.d., 10 μm) and TSK-G3000 (7.8 × 300 mm i.d., 5 μm) analytical columns in series maintained at a temperature of 35 °C. The columns were eluted with 0.02 M KH2PO4 buffer solution at a flow rate of 0.6 mL min−1. Dextran standards with different Mw (5.2, 11.6, 23.8, 48.6, 148, 273, 410, 668, and 1400 kDa) were used as standards. The calibration curve was obtained by plotting the retention time of standards against the logarithm of their respective Mw.
The sample (4 mg) was hydrolyzed with 4 mL of TFA (4 M) at 110 °C for 6 h in a 10 mL ampere bottle. Excess TFA was removed using a rotary evaporator at 45 °C. The residue was dissolved in 4 mL of methanol and evaporated to dryness using a nitrogen gas blowing instrument. The residue was re-dissolved in 8 mL of distilled water, filtered through a 0.22 μm microfilter and then analyzed by an ion chromatography (IC) instrument (Dionex ICS 3000, Sunnyvale, CA).23 Standards of fucose, glucose, rhamnose, xylose, mannose, galactose, arabinose, glucuronic acid and galacturonic acid were used to determine the equation of the standard curve. The content of monosaccharide was calculated using a linear regression equation for each monosaccharide with various concentrations proportional to peak areas.
IR spectra of CFG1 and CFG2 were determined using an IR spectrophotometer (Vector 33, Bruker, Germany). The sample was mixed with KBr powder and pressed into a pellet for measurement. The spectrum of the sample was recorded in the range of 400–4000 cm−1.
Interfacial tension measurement
Interfacial tension of the sample was measured at different concentrations (0, 0.5, and 2.0%) by the Wilhelmy plate method with a DCAT21 tensiometer (Datephysics CO., Germany).11 Corn oil was added into a measuring cup and placed in a temperature-control jacket on a moveable platform. A platinum plate was immersed in corn oil by raising the platform. After lowering the platform, the measuring cup was removed. A constant volume of sample solution was added to another measuring cup. Immediately afterward, corn oil was gently layered on the top of sample solution. After equilibrium for 1 min, the interfacial tension was measured.
Preparation of lutein emulsion
The formulation of emulsion was selected based on the result of optimization for preparing stable emulsions. The 5% of CFG was the maximum level used in this study. Each aqueous phase was prepared by dispersing 5.0 g of emulsifier sample into 90 mL phosphate buffer (pH 4.0) containing citric acid (0.13%) and sodium benzoate (0.12%). An organic phase was prepared by dissolving 25 mg lutein (250 mg L−1 in the final emulsion) into 5 g corn oil. A coarse oil-in-water emulsion was prepared by mixing each aqueous phase and each organic phase using a high-speed shear homogenizer T25 (IKA Co., Germany) for 2 min at 13
000 rpm. The resulting coarse emulsions were homogenized immediately using a single-pass laboratory-scale jet homogenizer M-110EH (MFIC Co., USA) three times at 50 MPa to obtain fine emulsion samples.
Droplet size and zeta-potential measurements
The average droplet size and distribution of emulsion was measured using a Mastersizer analyzer (MS2000, Worcestershire, UK). The average droplet size of emulsion was characterized by the surface area mean diameter: d3,2 = ∑nidi3/∑nidi2, where ni is number of droplets, and di is droplet diameter. The refractive indices of corn oil and buffer solution were 1.474 and 1.330, respectively. The d3,2 was manually calculated by data analysis software.
The ζ-potential of emulsion droplets was measured using a Zetasizer Nano-ZS instrument (Malvern Instruments, Worcestershire, U.K.) equipped with a MPT-2 pH autotitrator at room temperature. The diluted emulsions (1
:
10) were injected directly into the capillary electrophoresis cell and then inserted into the module. The ζ-potential was automatically calculated by measuring the electrophoresis mobility of the droplets.
Viscosity measurement
Viscosity of fresh emulsion was measured using a NDJ-8S digital viscometer (Shanghai Rex Instruments, China) at room temperature. The first rotator was selected and rotation speed was set at 60 rpm.
Encapsulation efficiency of lutein measurement
The 50 μL of emulsion sample was mixed with 4.95 mL of DMSO in a brown bottle and incubated at 40 °C for 1 h with ultrasonic treatment. The resulting mixture was centrifugated at 3500 rpm for 30 min and the supernatant was collected. The lutein concentration in the supernatant was determined by absorbance measurements at 446 nm according to a previous method.4 The encapsulation efficiency (EE) of lutein in the emulsion sample was calculated using the eqn (1): |
EE (%) = lutein content of supernatant/lutein content of emulsion
| (1) |
In vitro digestion assay
The in vitro digestion of lutein-enriched emulsions in a SGT model was carried out according to the method described previously.11 Briefly, emulsion sample (1.5 mL) was mixed with 13.5 mL of saline (containing 140 mM NaCl, 5 mM KCl, and 150 μM BHT) and stirred (500 rpm) for 10 min using a magnetic stirrer. The mixture was incubated at 37 °C and acidified to pH 1.2 with 1.0 M HCl solution. The resulting mixture was mixed with 1 mL of pepsin solution (40 mg mL−1) and incubated at 37 °C under magnetic stirring (500 rpm) for 1 h. After gastric digestion, the pH of the digestive sample was adjusted to 6.8 with 1.0 M NaOH and 4.5 mL of intestinal enzymes (including 2 mg mL−1 of pancreatin and 12 mg mL−1 of porcine bile extract prepared with 0.1 M NaHCO3) were added to start the digestion process, which occurred in a shaking water bath at 37 °C for 2 h. During lipolysis, the pH was maintained at 6.8 ± 0.02 by adding 0.2 M NaOH manually. The amount of NaOH consumed over time was recorded through intestinal ingestion to calculate the concentration of free fatty acids (FFA). Raw corn oil containing the same concentration of lutein as the emulsion was used as the control. The amount of FFA was calculated using the eqn (2): |
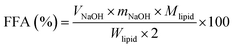 | (2) |
where VNaOH was the volume of NaOH required to neutralize the released FFA (L), mNaOH was the molarity of NaOH (in M), Wlipid was the total mass of corn oil initially present in the digestion emulsion (g), and Mlipid was average molecular mass of corn oil (872 g mL−1).
Determination of lutein bioaccessibility
The in vitro bioaccessibility of lutein was determined using the procedure described previously with a few modifications.10 In brief, the above end-product of digestion in the simulated gastrointestinal tract was centrifuged to obtain three phases (top, middle and bottom). The middle aqueous phase was filtered through a 0.22 μm microfilter (Millipore corp., Bedford, MA), and the concentration of lutein in the aqueous phase was determined by absorbance measurements (446 nm) according to a previous method.4 The bioaccessibility of lutein after digestion was calculated using the eqn (3): |
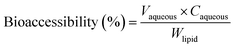 | (3) |
where Vaqueous was the volume of the aqueous phase, Caqueous was the concentration of lutein (not including chemical transformation of lutein) in the aqueous phase, and Wlipid was the mass of the lutein in corn oil, respectively.
Physical stability analysis
The physical stability of emulsion was evaluated by changes of droplet size and creaming index after seven days of storage. Fresh emulsion samples were transferred to glass tubes sealed with screw caps to prevent evaporation, and stored at room temperature for 7 days. During storage, the extent of creaming was characterized by the creaming index (%), which is defined as creaming index = (HS/HE) × 100%, where HS is the height of the serum layer and HE is the initial emulsion height. The change in d3,2 was measured using a Mastersizer analyzer.
Chemical stability analysis
The chemical stability of lutein emulsions was evaluated by lutein retention in emulsions under different storage conditions. The temperature and light had no significant effects on particle size and creaming stability of the emulsions. The changes of particle size and creaming index were similar with above results of physical stability (data not shown). For thermal stability analysis, 10 mL of fresh emulsion samples were transferred into 25 mL screw-cap bottles and stored in the dark at 25 and 55 °C for a period of 7 d. Another group of emulsions were stored in the dark or light at 25 °C for a period of 7 d. Changes of lutein content were measured every two days according to the method described previously.4
Results and discussion
Chemical composition and preliminary structure
The chemical compositions of CFG1 and CFG2 are shown in Table 1. The yields of CFG1 and CFG2 based on original corn fiber were 16.6 and 5.7%, respectively. The moisture contents of CFG1 and CFG2 were 9.66 and 11.3%, respectively. CFG1 contained carbohydrate (88.9%), protein (0.27%), and ferulic acid (0.45%), respectively, while CFG2 consisted of carbohydrate (85.6%), protein (1.0%), and ferulic acid (1.2%), respectively.
Table 1 Chemical compositions and structure of CFG1 and CFG2 from corn fiber
Item |
CFG1 |
CFG2 |
Yield (%) |
16.6 ± 0.3 |
5.7 ± 0.1 |
Moisture (%) |
9.66 ± 0.32 |
11.3 ± 0.15 |
Carbohydrate (%) |
88.9 ± 0.87 |
85.6 ± 3.9 |
Protein (%) |
0.27 |
1.0 |
Ferulic acid (%) |
0.45 |
1.2 |
![[thin space (1/6-em)]](https://www.rsc.org/images/entities/char_2009.gif) |
Monosaccharide compositions (molar percentage) |
Ara (%) |
24.4 |
25.0 |
Gal (%) |
11.4 |
11.4 |
Glc (%) |
3.97 |
6.58 |
Xyl (%) |
35.9 |
34.5 |
Man (%) |
0.16 |
1.40 |
GalA (%) |
19.8 |
18.1 |
GlcA (%) |
4.37 |
3.02 |
Molecular weight (kDa) |
319.2 |
382.8 |
The monosaccharide compositions of CFG1 and CFG2 as determined by ion-exchange chromatography are shown in Fig. 1. CFG1 and CFG2 were composed of Ara, Gal, Glu, Xyl, Man, GalA, and GlcA with similar molar ratios. According to the linear regression equation, CFG1 was composed of Ara, Gal, Glu, Xyl, Man, GalA, and GlcA in molar percentages of 24.4, 11.4, 3.97, 35.9, 0.16, 19.8, and 4.37%, respectively, and CFG2 consisted of Ara, Gal, Glu, Xyl, GalA, and GlcA in molar percentages of 25.0, 11.4, 6.58, 34.5, 1.40, 18.1, and 3.02%, respectively (Table 1). Previous studies reported that CFG was a high molecular-weight hydrophilic biopolymer composed of D-xylose (48–54%), L-arabinose (33–35%), galactose (7–11%), and glucuronic acid (3–6%).14,24 The result showed that CFG1 and CFG2 had different chemical compositions from previous reports.
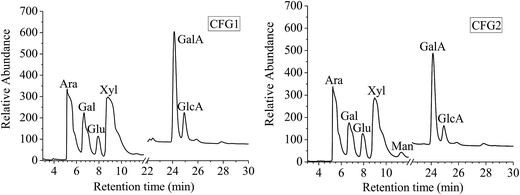 |
| Fig. 1 Monosaccharide composition IC profiles of CFG1 and CFG2. | |
The molecular weights of CFG1 and CFG2 were determined using HPGPC as shown in Fig. 2A. The regression equation of dextran standards was log
Mw = −0.2025X + 9.8398 (where Mw represents the molecular weight of dextrans and X represents the retention time) with a correlation coefficient of 0.999. Both CFG1 and CFG2 exhibited a single and symmetrical elution peak, indicating their homogeneity. According to the equation and retention time, the average molecular weights of CFG1 and CFG2 were calculated to be 319.2 and 382.8 kDa, respectively (Table 1).
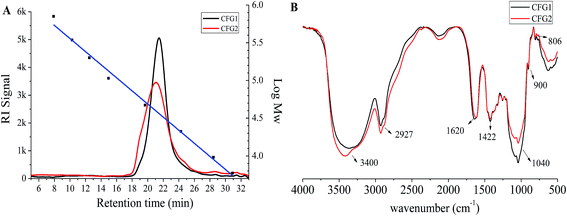 |
| Fig. 2 Mw distributions (A) and IR spectra (B) of CFG1 and CFG2. | |
IR analysis has been widely adopted to elucidate the structure and configuration of polysaccharides.22 As shown in Fig. 2B, the broad absorption peaks at 3400 cm−1 and weak absorption peaks at around 2927 cm−1 were the characteristic stretching peaks of O–H and C–H, respectively. The peaks at 1040 cm−1 were ascribed to the stretching vibration of C–O–C in xylopyranose rings.25 The stretching peaks at 900 cm−1 were the characteristic of β-D-pyranosidic configuration.12
Interfacial tension
In order to understand the interfacial characteristics of the samples, interfacial tensions of CFG1, CFG2 and GA solutions were measured. As shown in Fig. 3A, the interfacial tensions of the samples decreased with the increase in the concentrations from 0.1 to 2.0%. However, three samples showed significant differences to lower the interfacial tension. At the concentration of 2.0%, the interfacial tension values of CFG1, CFG2, and GA solutions were 20.8, 17.8, and 19.1 mN m−1, respectively. Interfacial characteristic of surface-active ingredient could influence the formation and stabilities of the emulsions.17 CFG2 with lower interfacial tension values resulted in the formation of smaller droplets during emulsification, thus forming a more stable emulsion.21,26 It is known that polysaccharide-based emulsifiers are less effective at reducing the interfacial tension than small molecular surfactant or proteins.17 The protein and ferulic acid played a main role in reducing the interfacial tension in the oil–water interface. Therefore, lower interfacial tension of CFG2 solution might be due to the fact that CFG2 contained more protein and ferulic acid. Overall, these results indicated that CFG2 was more effective than CFG1 and GA in reducing the interfacial tension of corn oil–water interface.
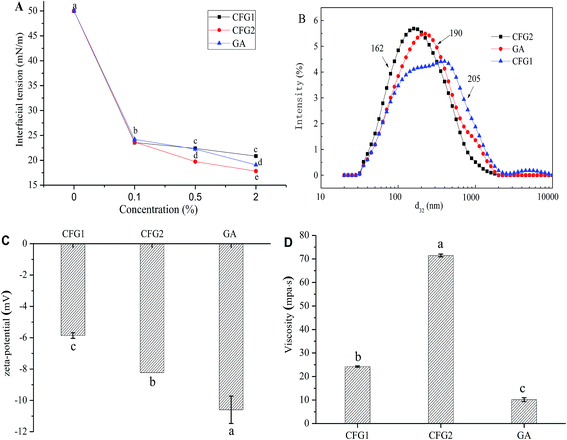 |
| Fig. 3 (A) Influence of CFG1, CFG2 and GA concentrations on the interfacial tension at the corn oil–water interface; (B) droplet size distribution and (C) ζ-potential values of emulsions stabilized by CFG1, CFG2 and GA, respectively; (D) viscosity of fresh prepared emulsions. Data with different letters are significantly different (p < 0.05). | |
Characterization of the emulsions
In order to compare the emulsifying properties of CFG1, CFG2, and GA, the formation of lutein loaded oil-in-water emulsions were initially investigated. Fig. 3B shows the droplet size distributions of three emulsions. Compared to CFG1-stabilized emulsion, CFG2-stabilized emulsion had a narrower droplet size distribution, ranging from 0.02–2.0 μm. The average d3,2 values of the emulsions stabilized by CFG1 and CFG2 were 205 and 162 nm, respectively. It is well known that the droplet size of O/W emulsion depends on two factors, including the initial formation of droplets diameter and the rapid stabilization of droplets by emulsifiers against bridging flocculation and re-coalescence.17 Thus, the final droplet size depends on droplet disruption ability and adsorption rate onto the surface of dispersed oil droplets of emulsifiers. The proteinaceous and ferulic acid moieties have been reported to facilitate the adsorption of CFG onto the surface of dispersed oil droplets.13–15,21 Similar results could be found in other polysaccharide-based emulsifiers, such as beet pectin and gum arabic.21,27 Therefore, high contents of protein and ferulic acid of CFG2 might explain its good emulsifying property.
ζ-potential is an important indication of electrostatic repulsion force. Droplets with higher absolute ζ-potential can reduce aggregation and flocculation of the droplets, which was more favorable for the emulsion stability.27,28 The ζ-potentials of lutein loaded emulsions are shown Fig. 3C. The ζ-potential values of three emulsions were −5.86, −8.23, and −10.6 mV, respectively. CFG2-stabilized emulsion had higher absolute ζ-potential, indicating that the emulsion was more stable.29 In addition, CFG1 and CFG2 were indicated as anionic emulsifiers, which were due to the existence of ferulic acid in their molecular chain.15,30
Fig. 3D shows the viscosity of lutein emulsions stabilized by CFG1, CFG2 and GA at the same concentration. The emulsion stabilized by CFG2 had higher apparent viscosity than CFG1 and GA. The ability of polysaccharides to increase viscosity depends on their molecular weight, conformation and interactions. Increasing molecular weight and radius of hydration could increase the viscosity of polymer solution.17,31 Therefore, differences in apparent viscosities of CFG1 and CFG2 might be caused by their different molecule weight and structure.
In vitro digestion and bioaccessibility of lutein emulsions
To investigate the effects of CFG1, CFG2 and GA on the lipid digestion and bioaccessibility of lipid-soluble lutein, corn oil and three lutein-enriched emulsions were digested in the SGT. Fig. 4A showed different digestion profiles in four formulations. The amount of FFA released increased with increasing of digestion time during the first 100 min, at which point the FFA reached a plateau level. The final amount of FFA release decreased in the order of CFG2 (90.9%) > CFG1 (82.7%) > GA (74.4%) > corn oil (26.9%). In addition, the rate of FFA release also decreased in the order of CFG2 > CFG1 > GA > corn oil. The lower rate and extent of bulk oil (corn oil) was mainly attributed to the low reactive area available for lipase adsorption due to the large oil droplet size.8,10,32 Thus, CFG2-stabilized emulsion exhibited the maximum rate and extent of FFA release.
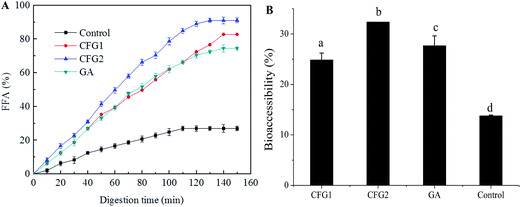 |
| Fig. 4 (A) Release profiles of FFA released from corn oil and emulsions in the digestion process; (B) bioaccessibilities of lutein in three emulsions and bulk oil (corn oil) after in vitro lipolysis. Data with different letters are significantly different (p < 0.05). | |
After lipid digestion, the lipid was hydrolyzed into diacylglycerols and monoglycerols. The FFA released from the interface of oil droplets will combine with bile salts and phospholipids to form micelles, in which lutein dissolved in lipid could be solubilized and thus become bioaccessible.33,34 As shown in Fig. 4B, lutein bioaccessibilities of emulsions were greatly improved compared to that of corn oil. The encapsulation efficiency of lutein in CFG1-, CFG2-, and GA-stabilized emulsions were 79, 86, and 88%, respectively. Based on the results of encapsulation efficiency of lutein, the bioaccessibility of lutein decreased in the order CFG2 (32.4%) > GA (27.7%) > CFG1 (24.9%) > corn oil (13.8%), indicating that an increase in the extent of lipolysis would improve bioaccessibility similar results were found in the reported studies.8,32 The results demonstrated that corn fiber gum-stabilized emulsion could be used as a delivery system for improving the bioavailability of lutein. It has been reported that 10 mg per day of lutein is an effective dose for providing protection against diseases.35 Based on the above results, the lowest effective dosage of lutein in the emulsions absorbed by human body was calculated to be >49.2 mg mL−1. Therefore, CFG-stabilized lutein emulsions could meet the demand for effective dosage of lutein in commercial products.
Physical stability of lutein emulsions
The changes in d3,2 and creaming index of the emulsions are shown in Table 2. Moreover, to visually observe the creaming, optical photographs were captured. As shown in Fig. 5, fresh lutein emulsions exhibited more stability, and no creaming was appeared. After 7 days of storage, the CFG2-stabilized emulsion kept good stability. The d3,2 increased slightly from 162 to 172 nm. However, a different extent of creaming were observed in the CFG1- and GA-stabilized emulsions, with a creaming index of 7% and 3%, respectively (Table 2). The d3,2 of the emulsion stabilized by CFG1 remained the same, whereas the d3,2 of the emulsion stabilized by GA slightly increased from 190 to 212 nm. Droplet size and viscosity greatly influenced the creaming velocity of emulsion, which can be explained by Stokes' Law.36 Higher viscosity of the emulsion stabilized by CFG2 (71.5 MPa s) could also explain higher emulsion stability. This phenomenon can be supported by the previous study that higher viscosity could improve the emulsion stability through retarding free motion of droplets.22 However, these emulsions with low absolute ζ-potential values exhibited excellent stability after 7 days of storage, which was due to that the electrostatic repulsive force was not the main stabilization mechanism for this emulsions. Previous studies have reported that polysaccharide-based emulsifiers, such as gum arabic, corn fiber gum, and beet pectin, favor the emulsion stability mainly through thickening and steric repulsion generated by their molecular structure.11,17 In all, this result indicated that CFG has better stabilizing capacity than GA.
Table 2 Changes in d3,2 and creaming index of the emulsions stabilized by CFG1, CFG2 and GAa
Sample |
Fresh emulsions |
After 7 d of storage |
d3,2 (nm) |
CI (%) |
d3,2 (nm) |
CI (%) |
Data in columns with different superscript letters are significantly different (p < 0.05). |
CFG1 |
205a |
0a |
205 ± 1.0a |
7.0 ± 1.0a |
CFG2 |
162b |
0a |
172 ± 2.3b |
0b |
GA |
190 ± 0.5c |
0a |
212 ± 2.0c |
3.0c |
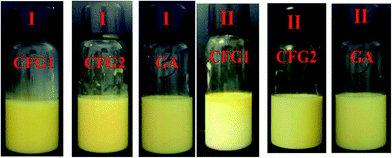 |
| Fig. 5 Optical photographs of the emulsions stabilized by CFG1, CFG2, and GA, respectively. (I) Fresh emulsions; (II) the emulsions after storage for 7 d at room temperature. | |
Chemical stability of emulsion loaded with lutein
In the present of heat, light, and oxygen, lutein is readily prone to isomerization and chemical degradation.37 We therefore monitored the chemical stability of lutein in the emulsion by measuring the degradation extent of lutein during different storage conditions.
As shown in Fig. 6, after storage at 25 and 55 °C in the dark or in the light for a period of time, the lutein degradation of the emulsions increased with the increase in the storage time but in a slower speed compared to the control. In addition, the degradation rates of lutein increased with the increase in the storage temperature, and in the present of/irradiation by light, the lightness could also accelerate the degradation of lutein. This result further explained the change of color from yellow to light yellow in the CFG1-, CFG2-, and GA-stabilized emulsions. Similar result was found in the reported study that lutein was prone to degrade at higher temperatures or in the light.4 However, lutein of the emulsions stabilized by CFG2 and CFG1 showed higher stabilities than GA. These results indicated that CFG-stabilized emulsion was capable of inhibiting deterioration of lutein. Taken together, CFG revealed better emulsifying properties and improved physical and chemical stabilities of lutein emulsions when compared to a commercial emulsifier (GA).
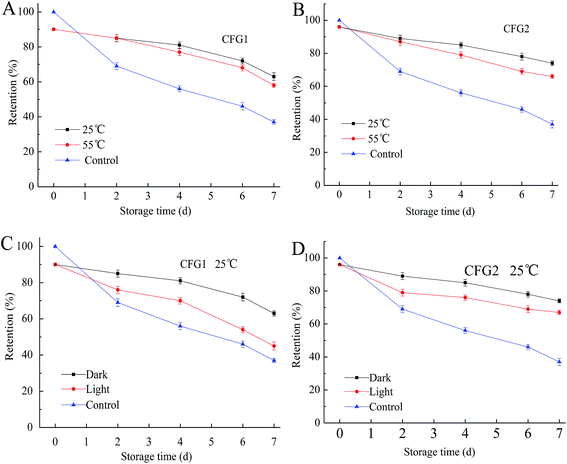 |
| Fig. 6 Degradation of lutein in emulsions stabilized by CFG1 and CFG2 under different storage conditions for 7 d. Lutein in the bulk oil was used as a control. | |
Conclusion
In this study, CFG1 and CFG2 were isolated from corn fiber by the alkaline hydrogen peroxide method. CFG1 contained 88.9% of carbohydrate, 0.27% of protein, and 0.45% of ferulic acid, while CFG2 contained 85.6% of carbohydrate, 1.0% of protein, and 1.2% of ferulic acid. CFG1 and CFG2 had similar monosaccharide compositions but different from previous reports.14,24 CFG2 exhibited better emulsifying capacity and emulsification stability than CFG1 and GA. The lutein bioaccessibilities of the emulsions stabilized by CFG1 and CFG2 were increased from 13.8% (corn oil) to 24.9% and 32.4%, respectively. During storage, CFG1- and CFG2-stabilized emulsions could significantly inhibit the degradation of lutein under the conditions of high temperature and the light. These results suggest that corn fiber gum could be used to fabricate emulsions for delivering lutein or other lipophilic bioactive compounds. Thus, this research contributes to the development and utilization of this natural emulsifier in the food industry.
Acknowledgements
This work was funded by the China Postdoctoral Science Foundation (2016M602474), Science and Technology Planning Project of Nansha, Guangzhou (2016GJ001) and the Fundamental Research Funds for the Central Universities of China (2017BQ100).
References
- J. Mares, Annu. Rev. Nutr., 2016, 36, 571–602 CrossRef CAS PubMed.
- B. Nidhi, G. Sharavana, T. R. Ramaprasad and B. Vallikannan, Food Funct., 2015, 6, 450–460 CAS.
- T. T. Y. Woo, S.-Y. Li, W. W. K. Lai, D. Wong and A. C. Y. Lo, Graefe's Arch. Clin. Exp. Ophthalmol., 2013, 251, 41–51 CrossRef CAS PubMed.
- G. Davidov-Pardo, C. E. Gumus and D. J. McClements, Food Chem., 2016, 196, 821–827 CrossRef CAS PubMed.
- D. J. McClements and Y. Li, Adv. Colloid Interface Sci., 2010, 159, 213–228 CrossRef CAS PubMed.
- D. J. McClements, E. A. Decker and J. Weiss, J. Food Sci., 2007, 72, R109–R124 CrossRef CAS PubMed.
- A. Nagao, Jpn. Agric. Res. Q., 2014, 48, 385–391 CrossRef.
- J. Xiao, C. Li and Q. Huang, J. Agric. Food Chem., 2015, 63, 10263–10270 CrossRef CAS PubMed.
- C. E. Gumus, G. Davidov-Pardo and D. J. McClements, Food Hydrocolloids, 2016, 60, 38–49 CrossRef CAS.
- P. S. Given, Curr. Opin. Colloid Interface Sci., 2009, 14, 43–47 CrossRef CAS.
- V. Castel, A. C. Rubiolo and C. R. Carrara, Food Hydrocolloids, 2017, 63, 170–177 CrossRef CAS.
- S. Patel and A. Goyal, Int. J. Food Prop., 2015, 18, 986–998 CrossRef CAS.
- D. J. McClements, L. Bai and C. Chung, Annu. Rev. Food Sci. Technol., 2017, 8, 205–236 CrossRef PubMed.
- M. P. Yadav, D. B. Johnston, A. T. Hotchkiss Jr and K. B. Hicks, Food Hydrocolloids, 2007, 21, 1022–1030 CrossRef CAS.
- S. Kokubun, M. P. Yadav, R. A. Moreau and P. A. Williams, Food Hydrocolloids, 2014, 41, 164–168 CrossRef CAS.
- K. S. Mikkonen, M. P. Yadav, P. Cooke, S. Willför, K. B. Hicks and M. Tenkanen, BioResources, 2008, 3, 178–191 Search PubMed.
- L. Bai, S. Huan, Z. Li and D. J. McClements, Food Hydrocolloids, 2017, 66, 144–153 CrossRef CAS.
- S. Qiu, M. P. Yadav, H. Chen, Y. Liu, E. Tatsumi and L. Yin, Carbohydr. Polym., 2015, 115, 246–252 CrossRef CAS PubMed.
- M. Dubois, K. A. Gilles, J. K. Hamilton, P. Rebers and F. Smith, Anal. Chem., 1956, 28, 350–356 CrossRef CAS.
- O. H. Lowry, N. J. Rosebrough, A. L. Farr and R. J. Randall, J. Biol. Chem., 1951, 193, 265–275 CAS.
- E. N. Fissore, A. M. Rojas, L. N. Gerschenson and P. A. Williams, Food Hydrocolloids, 2013, 31, 172–182 CrossRef CAS.
- C. Li, X. Fu, F. Luo and Q. Huang, Food Hydrocolloids, 2013, 32, 79–86 CrossRef CAS.
- C. Li, X. Li, L. You, X. Fu and R. H. Liu, Carbohydr. Polym., 2017, 155, 261–270 CrossRef CAS PubMed.
- M. P. Yadav, M. L. Fishman, H. K. Chau, D. B. Johnston and K. B. Hicks, Cereal Chem., 2007, 84, 175–180 CrossRef CAS.
- R. C. Sun and X. F. Sun, Carbohydr. Polym., 2002, 49, 415–423 CrossRef CAS.
- Y. Zhang, C. Tan, S. Abbas, K. Eric, S. Xia and X. Zhang, Food Hydrocolloids, 2015, 51, 108–117 CrossRef CAS.
- H.-m. Chen, X. Fu and Z.-g. Luo, Food Hydrocolloids, 2016, 54, 99–106 CrossRef CAS.
- P. Shao, Y. Zhu and W. Jin, Food Hydrocolloids, 2017, 64, 28–35 CrossRef CAS.
- Y. Yamanaka, I. Kobayashi, M. A. Neves, S. Ichikawa, K. Uemura and M. Nakajima, LWT--Food Sci. Technol., 2017, 76, 344–350 CrossRef CAS.
- J. Cirre, S. Al-Assaf, G. O. Phillips, M. P. Yadav and K. B. Hicks, Food Hydrocolloids, 2014, 35, 122–128 CrossRef CAS.
- X. Yu, C. Zhou, H. Yang, X. Huang, H. Ma, X. Qin and J. Hu, Carbohydr. Polym., 2015, 117, 650–656 CrossRef CAS PubMed.
- L. Salvia-Trujillo, C. Qian, O. Martín-Belloso and D. J. McClements, Food Chem., 2013, 141, 1472–1480 CrossRef CAS PubMed.
- R. Liang, C. F. Shoemaker, X. Yang, F. Zhong and Q. Huang, J. Agric. Food Chem., 2013, 61, 1249–1257 CrossRef CAS PubMed.
- Y. Ting, Y. Jiang, C.-T. Ho and Q. Huang, J. Funct. Foods, 2014, 7, 112–128 CrossRef CAS.
- K. Frede, A. Henze, M. Khalil, S. Baldermann, F. J. Schweigert and H. Rawel, J. Funct. Foods, 2014, 8, 118–127 CrossRef CAS.
- M. N. Nasrabadi and S. A. H. Goli, Food Chem., 2016, 199, 258–264 CrossRef PubMed.
- M. M. Alam, K. Ushiyama and K. Aramaki, J. Oleo Sci., 2009, 58, 361–367 CrossRef CAS PubMed.
Footnote |
† Current address: School of Food Science and Engineering, South China University of Technology, 381 Wushan Road, Guangzhou 510640, China. |
|
This journal is © The Royal Society of Chemistry 2017 |