DOI:
10.1039/C7RA04797E
(Paper)
RSC Adv., 2017,
7, 33568-33571
Highly permeable ionic liquid 1-butyl-3-methylimidazoliumtetrafluoroborate (BMIMBF4)/CuO composite membrane for CO2 separation
Received
28th April 2017
, Accepted 26th June 2017
First published on 3rd July 2017
Abstract
An ionic liquid (IL) 1-butyl-3-methylimidazoliumtetrafluoroborate (BMIM+BF4−)/CuO composite was prepared for CO2 transport membranes. CuO nanoparticles (NPs) were successfully generated in BMIM+BF4−. The formation of CuO NPs was ascribed to the favorable interaction between the ions of BMIM+BF4− and the surface of the CuO particles. To confirm the coordination behavior of the BMIM+BF4−/CuO membrane, TGA and FT-Raman spectroscopy were employed. The ideal CO2/N2 selectivity in the case of the composite material was 21.0, with a CO2 permeance of 52.4 GPU, while the neat BMIM+BF4− membrane showed a selectivity of 5.0 and a CO2 permeance of 17.0 GPU. The enhanced separation performance was attributed to (1) the synergistic effect of well-dispersed CuO nanoparticles, resulting in enhanced CO2 solubility due to the increased surface of the oxide particle layer and (2) the abundant free ions in BMIM+BF4−.
I. Introduction
Presently, one of the greatest challenges for researchers is finding a thermodynamically efficient process for separating and capturing CO2.1 It has been commonly accepted that global warming is related directly to the increased levels of CO2.2–4 CO2 is mainly emitted as a result of the combustion of fossil fuels, certain chemical plant processes, and production of synthesis gas.5 Currently, the principal CO2 separation technologies for post-combustion capture include physical adsorption, physical or chemical absorption, cryogenic distillation, and membrane separation.6,7 Among the various types of CO2 separation technologies, membrane technology promises excellent separation performance while being relatively less energy- and capital intensive.8–11 However, current commercial polymeric membranes, such as polyimide and cellulose acetate membranes, have low CO2 selectivity and permeability, since they are based on a solution-diffusion mechanism and rely chiefly on the subtle size differences of the penetrants to accomplish separation.12,13 To overcome the low separation performance of polymeric membranes, facilitated transport was introduced so that higher gas permeability and outstanding selectivity could be achieved.14–16 The facilitated transport membrane has attracted attention due to the reversible reaction with a specific gas component.17 Hence, the reaction in the membrane can generate another transport mechanism alongside the simple solution-diffusion mechanism.18,19
Given the above, ionic liquids (ILs) have been utilized to prepare facilitated CO2 transport membranes.20,21 ILs have attracted great attention because of their highly tunable properties such as low flammability, high chemical and thermal stability, and extremely low volatility.22 Moreover, ILs based on imidazolium cations present outstanding CO2 separation performance since the amine group in the imidazolium ring acts a CO2 carrier in CO2/CH4 and CO2/N2 separation.23 For example, CO2 separation performance utilizing 1-butyl-3-methylimidazolium tetrafluoroborate (BMIM+BF4−) showed that CO2/N2 and CO2/CH4 ideal selectivity were 5 and 4.8, respectively.24 Surprisingly, the CO2 permeance was 17 GPU.24 Furthermore, in the case of CO2 separation utilizing pure 1-butyl-3-methylimidazolium nitrate (BMIM+NO3−), the ideal CO2/N2 selectivity and CO2 permeance were 4.7 and 5.2 GPU, respectively.25
To enhance the CO2 separation performance of neat ILs, our group investigated selective CO2 separation utilizing ILs with lithium tetrafluoroborate (LiBF4).26 It was established that the facilitated transport membrane consisting of lithium tetrafluoroborate as a CO2 carrier and BMIM+BF4− exhibits an ideal selectivity of 8.40 for CO2/N2 with a CO2 permeance of 13.36 GPU.26 Lee et al. reported that a 1-methyl-3-octylimidazolium tetrafluoroborate (MOIM+BF4−)/positively polarized Cu nanoparticle membrane shows CO2/N2 and CO2/CH4 selectivities of 25.2 and 24.3, respectively, with a CO2 permeance of 24.2 GPU.27 In addition, our group reported that BMIM+NO3−/Cu nanoparticle membranes demonstrate a CO2 permeance of 9.8 GPU with an ideal selectivity of 12.25 for CO2/N2 and CO2/CH4.25 These results indicated that Cu NPs could be an excellent carrier for CO2 separation in ILs.
Very recently, our group reported that metal oxide nanoparticles could be utilized for a CO2 separation membrane. For example, an MOIM+BF4−/AgO composite membrane showed a high ideal selectivity of 23.4 for CO2/N2, with a CO2 permeance of 18.7 GPU; on the other hand, the neat MOIM+BF4− membrane showed a selectivity of 15.9, with a CO2 permeance of 12.7 GPU.28 Furthermore, when AgO particles were added to BMIM+BF4−, a CO2/N2 selectivity of 28.2 and CO2 permeance of 14.1 GPU were reported.29 These results indicated that the dissociated AgO nanoparticles have strong affinity for CO2 due to the oxide layer.29
In this study, in order to clarify the effect of the metal oxide in ILs, experiments were conducted with other oxides. Copper(II) oxide was selected because its properties are similar to those of silver oxide. For the BMIM+BF4−/CuO composite membrane, the dissociated CuO particles were expected to enhance the solubility of the CO2 molecules due to the nano-oxide particle layer. Moreover, when CuO was incorporated into BMIM+BF4−, the free ions could also enhance the solubility of CO2 molecules since the quadrupole moment of CO2 was relatively higher than N2, resulting in the increased separation performance.
II. Experimental
2.1 Materials
BMIM+BF4− was purchased from Merck KGaA (Darmstadt, Germany). CuO was purchased from Sigma-Aldrich Chemical Co. Ethyl alcohol (>94.0% purity) was purchased from Daejung Chemicals & Metals. All chemicals were used as received.
2.2 Characterization
A sonifier (Branson 450, Branson Ultrasonics Corporation, Danbury CT, USA) with a standard tip was used. Scanning electron microscopy (SEM) images were obtained using a JEOL JSM-5600LV system. Transmission electron microscopy (TEM) images were obtained using a JEM-2010 system operating at 200 kV. The weight loss was measured by thermogravimetric analysis (TGA, TGA Q50, TA Instrument) of the complex membrane in flowing N2. FT-Raman measurements were performed for the BMIM+BF4−/CuO solution at room temperature using a Horiba Jobin Yvon LabRam Aramis apparatus with a diode laser beam at an excitation wavelength of 785 nm.
2.3 Membrane preparation
The membranes were prepared utilizing BMIM+BF4−, copper(II) oxide, and ethanol. First, copper(II) oxide was sonicated to disperse in ethanol for 5 min, and then, BMIM+BF4− was added into the mixture. The dispersion was heated at 85 °C for 24 h to evaporate ethanol. The final solution was coated onto a polysulfone support (Toray Chemical Korea Inc.) and then cast using an RK control coater (Model 202, Control Coater RK Print-Coat Instruments Ltd., UK). The best performance of BMIM+BF4−/CuO was observed at a weight ratio of 1/0.007.
2.4 Gas separation performance
Gas flow rates were measured with a bubble flow meter at an upstream pressure of 2 kgf·cm−2 and atmospheric downstream pressure. The unit of gas permeance is GPU, with 1 GPU = 1 × 10−6 cm3 (STP)/(cm2 s cmHg). Selectivity was defined as ideal selectivity (CO2 permeance/N2 permeance).
III. Results and discussion
3.1 Transmission electron microscope (TEM) image of BMIM+BF4−/CuO
TEM images were recorded to investigate the size and dispersity of the dissociated copper oxide particles in BMIM+BF4−. As shown in Fig. 1, no significant copper(II) oxide agglomerates were present, and CuO particles were mostly observed as small nanoparticles with an average diameter of 10 nm. These results indicated that the dissociated CuO nanoparticles are advantageous for increasing the solubility of CO2 molecules.
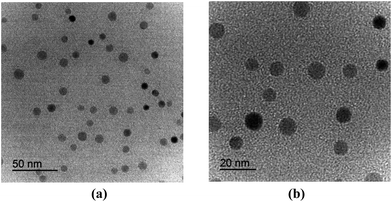 |
| Fig. 1 (a) TEM image of the dissociated CuO nanoparticles in BMIM+BF4− and (b) enlarged image. | |
3.2 Separation performance
Fig. 2 presents the cross-section structures of the BMIM+BF4−/CuO composite membrane. The utilized polysulfone support had a finger-like structure. The thickness of the coated selective layer was approximately 4.1 μm. Based on the SEM image, it was suggested that the selective layer was not penetrated into the polysulfone microporous support.
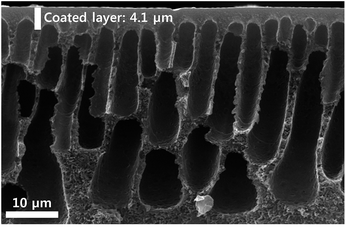 |
| Fig. 2 SEM image of BMIM+BF4−/CuO composite membrane. | |
To investigate the effect of CuO concentration in the BMIM+BF4−/CuO membrane on the CO2 separation performance, the pure gas permeance of CO2 and N2 through the BMIM+BF4−/CuO composite membrane was measured at increasing weight ratios of CuO, as shown in Fig. 3. As the CuO weight ratio increased, the CO2 selectivity also sharply increased. As shown in Table 1, the highest CO2 permeance (52.4 GPU) and CO2/N2 selectivity (21.0) were observed at a BMIM+BF4−/CuO weight ratio of 1/0.007. At CuO weight ratios higher than 0.007, both CO2 permeance and selectivity remained unchanged. However, above a CuO weight ratio of 0.04, the copper oxide was not well dispersed in BMIM+BF4−, and therefore, significant copper oxide agglomerates were present. A previous study revealed that for neat BMIM+BF4−, the CO2 permeance and CO2/N2 selectivity were 17.0 GPU and 5.0, respectively, as shown Table 1.24 On the other hand, when CuO was added to BMIM+BF4−, the CO2 permeance increased from 17 to 52.4 GPU and the CO2/N2 selectivity increased from 5.0 to 21.0. Furthermore, it was confirmed that CO2 permeance was much higher than the value reported in previous study consisting of BMIMBF4/AgO composite. (CO2 permeance: 14.1 GPU)29 These results were ascribed to two factors: (1) well-dispersed CuO nanoparticles, which could lead to enhanced CO2 solubility due to the increased oxide layer and (2) abundant free ions in BMIM+BF4−, enabling the enhanced CO2 transport.
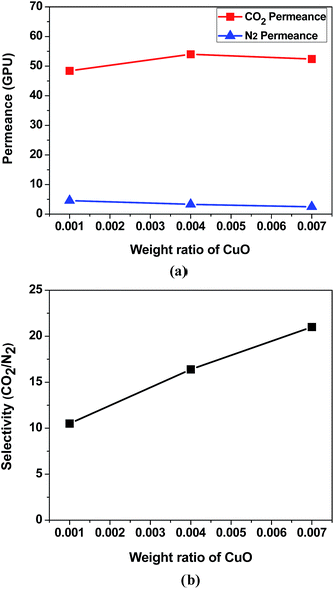 |
| Fig. 3 Gas separation performance of BMIM+BF4−/CuO composite membranes: (a) pure gas permeance and (b) ideal CO2/N2 selectivity. | |
Table 1 Pure gas permeance and selectivity of neat BMIM+BF4− and BMIM+BF4−/CuO membranes with a weight ratio of 1/0.007
|
CO2 permeance (GPU) |
N2 permeance (GPU) |
CO2/N2 selectivity |
Neat BMIM+BF4− (ref. 24) |
17.0 |
3.4 |
5.0 |
BMIM+BF4−/AgO29 |
14.1 |
0.5 |
28.2 |
BMIM+BF4−/CuO |
52.4 |
2.5 |
21.0 |
3.3 Thermogravimetric analysis
The thermal properties of the neat BMIM+BF4− and BMIM+BF4−/CuO composite membranes were analyzed by TGA. Fig. 4 presents weight loss at temperatures from 350 to 500 °C. The major weight loss was ascribed to the structural decomposition of the IL BMIM+BF4. The BMIM+BF4−/CuO composite showed slower decomposition with a smaller weight loss of 70.6% at 450 °C, as compared to that (53.2%) of neat BMIM+BF4− at the same temperature. If the agglomeration of CuO particles in BMIM+BF4− occurred, the decomposition temperature would remain constant. However, this result indicates that CuO particles were well dispersed in the IL BMIM+BF4−, because of the coordinative interaction between the CuO surface and BMIM+BF4−. Thus, the thermal stability of the BMIM+BF4−/CuO composite membrane increased.
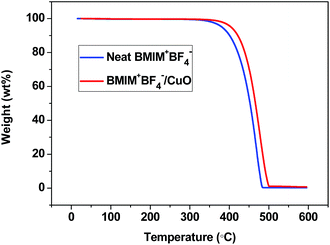 |
| Fig. 4 TGA results for neat BMIM+BF4− (blue) and BMIM+BF4−/CuO composite (red). | |
3.4 FT-Raman spectroscopy
Interactions between BMIM+BF4− and CuO in the BMIM+BF4−/CuO composite membranes were investigated by FT-Raman spectroscopy. The Raman spectrum in the region of the BF4− stretching bands is shown in Fig. 5. Three different ionic species were observed in the FT-Raman spectra of the ILs: free ions, ion pairs, and ionic aggregates. The BF4− stretching bands at 765, 770, and 774 cm−1 were assigned to the free ions, ion pairs, and ion aggregates, respectively.30 BMIM+ forms ion aggregates and ion pairs with BF4− in BMIM+BF4− through hydrogen bonding.24 A previous report showed that the Raman spectrum of neat BMIM+BF4− included signals attributed to ion pairs and ion aggregates.24 As the copper oxide is well dispersed in BMIM+BF4−, free BMIM+ and BF4− ions are observed. These results can be explained by the coordinative interaction between BMIM+BF4− and the surface of the CuO nanoparticles, which results in weakened interactions between BMIM+ and BF4−. Therefore, CO2 separation performance could be enhanced by the increase of solubility due to abundant free ions.
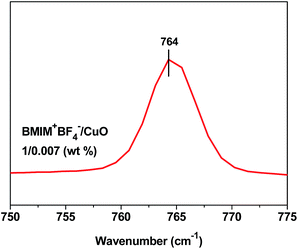 |
| Fig. 5 FT-Raman spectrum of BMIM+BF4−/CuO nanoparticles. | |
IV. Conclusions
We have successfully stabilized CuO nanoparticles by employing an ionic liquid BMIM+BF4−. The coordinative interactions of the BMIM+BF4−/CuO composite membrane were characterized by TEM, TGA, and FT-Raman analyses. The separation performance of the BMIM+BF4−/CuO composite membrane was considerably enhanced compared to that of the neat BMIM+BF4−. When CuO was incorporated into BMIM+BF4−, the CO2/N2 selectivity increased to 21.0, with a CO2 permeance of 52.4 GPU. These results were attributed to (1) the synergistic effect of well-dispersed CuO nanoparticles, which result in enhanced CO2 solubility due to the increased surface of the oxide particle layer and (2) the abundant free ions in BMIM+BF4−.
Acknowledgements
This work was supported by an Energy Efficiency & Resources grant from the Korea Institute of Energy Technology Evaluation and Planning (KETEP), funded by the Korean Government via the Ministry of Trade, Industry, and Energy (20122010100040). This work was also supported by the Basic Science Research Program (2017R1D1A1B03032583) through the National Research Foundation of Korea (NRF), and funded by the Ministry of Science, ICT, and Future Planning.
Notes and references
- N. V. Blinova and F. Svec, J. Mater. Chem. A, 2014, 2, 600–604 CAS.
- N. Du, H. B. Park, M. M. Dal-Cin and M. D. Guiver, Energy Environ. Sci., 2012, 5, 7306–7322 CAS.
- D. Aaron and C. Tsouris, Sep. Sci. Technol., 2005, 40, 321–348 CrossRef CAS.
- H. Q. Yang, Z. H. Xu, M. H. Fan, R. Gupta, R. B. Slimane, A. E. Bland and I. Wright, J. Environ. Sci., 2008, 20, 14–27 CrossRef CAS.
- J. Huang, J. Zou and W. S. Winston Ho, Ind. Eng. Chem. Res., 2008, 47, 1261–1267 CrossRef CAS.
- C. H. Yu, C. H. Huang and C. S. Tan, Aerosol Air Qual. Res., 2012, 12, 745–769 CAS.
- O. Davidson and B. Metz, International Panel on Climate Change, 2005 Search PubMed.
- P. Jungho, K. Deukju and N. Sangyong, Membr. J., 2015, 25, 547–557 CrossRef.
- P. arume and K. Sungjoong, Membr. J., 2016, 26, 126–134 CrossRef.
- R. W. Baker and K. Lokhandwala, Ind. Eng. Chem. Res., 2008, 47, 2109–2121 CrossRef CAS.
- W. Yave, A. Car, J. Wind and K. V. Peinemann, Nanotechnology, 2010, 21, 395301 CrossRef PubMed.
- A. Meisen and X. Shuai, Energy Convers. Manage., 1997, 38, S37–S42 CrossRef CAS.
- W. J. Koros and R. Mahajan, J. Membr. Sci., 2000, 175, 181–196 CrossRef CAS.
- L. Deng, T. J. Kim and M. B. Hagg, J. Membr. Sci., 2009, 340, 154–163 CrossRef CAS.
- W. J. Ward and W. L. Robb, Science, 1957, 156, 1481–1484 CrossRef.
- R. Yegani, H. Hirozawa, M. Teramoto, H. Himei, O. Okada, T. Takigawa, N. Ohmura, N. Matsumiya and H. Matsuyama, J. Membr. Sci., 2007, 291, 157–164 CrossRef CAS.
- S. Kasahara, E. Kamio, T. Ishigami and H. Matsuyama, Chem. Commun., 2012, 48, 6903–6905 RSC.
- S. U. Hong, J. H. Jin, J. Won and Y. S. Kang, Adv. Mater., 2000, 12, 968–971 CrossRef CAS.
- Y. Suzuki, H. Nishide and E. Tsuchida, Macromol. Res., 2000, 33, 2530–2534 CrossRef CAS.
- C. Yu, T. Zhu, X. Zhang and H. Liu, Sci. Adv. Mater., 2015, 7, 1213–1220 CrossRef CAS.
- J. Chang, G. H. Hong and S. W. Kang, RSC Adv., 2015, 5, 69698–69701 RSC.
- P. T. Nguyen, B. A. Voss, E. F. Wiesenauer, D. L. Gin and R. D. Noble, Ind. Eng. Chem. Res., 2013, 52, 8812–8821 CrossRef CAS.
- K. E. Gutowski and E. J. Maginn, J. Am. Chem. Soc., 2008, 130, 14690–14704 CrossRef CAS PubMed.
- J. H. Lee, J. Hong, J. H. Kim, Y. S. Kang and S. W. Kang, Chem. Commun., 2012, 48, 5298–5300 RSC.
- G. H. Hong, J. H. Oh, D. Ji and S. W. Kang, Chem. Eng. J., 2014, 252, 263–266 CrossRef CAS.
- Y. Choi, G. H. Hong and S. W. Kang, J. Nanosci. Nanotechnol., 2016, 16, 2832–2835 CrossRef CAS PubMed.
- J. H. Lee, J. Hong, J. H. Kim, D. Song, Y. S. Kang and S. W. Kang, Chem. Eng. J., 2014, 235, 252–256 CrossRef CAS.
- D. Ji and S. W. Kang, Korean J. Chem. Eng., 2016, 33(2), 666–668 CrossRef CAS.
- D. Ji, Y. S. Kang and S. W. Kang, Sci. Rep., 2015, 5, 16362 CrossRef CAS PubMed.
- S. W. Kang, D. H. Lee, J. H. Park, K. Char, J. H. Kim, J. Won and Y. S. Kang, J. Membr. Sci., 2008, 322, 281–285 CrossRef CAS.
|
This journal is © The Royal Society of Chemistry 2017 |