DOI:
10.1039/C7RA04577H
(Paper)
RSC Adv., 2017,
7, 32601-32606
A biomimetic ion-crosslinked layered double hydroxide/alginate hybrid film†
Received
24th April 2017
, Accepted 20th June 2017
First published on 27th June 2017
Abstract
Natural nacre has an ordered layered arrangement of calcium carbonate platelets and ion-crosslinked protein, which enable it to achieve outstanding mechanical properties. Inspired by the relationship between structure and mechanical properties of natural nacre, we fabricate a Ca2+-crosslinked layered double hydroxide/alginate (LDH/ALG-Ca2+) hybrid film through filtration of Ca2+-crosslinked LDH/ALG hybrid building blocks. The LDH nanoplatelets and alginate were alternately stacked giving an ordered layered structure, in which alginate was crosslinked by Ca2+. The effect of LDH content and Ca2+ crosslinking on the microstructure and interfacial interaction of LDH/ALG-Ca2+ hybrid films was studied systematically. The optimized nacre-like hybrid film exhibits good flexibility and high strength (194 MPa), superior to natural nacre. The ordered layered structure and small diameter of LDH give the hybrid film a high transparency of 74–94% in the visible light wavelength range.
1. Introduction
Natural nacre has a layered structure with alternately stacked calcium carbonate platelets and ion-crosslinked protein, which are connected together through plenty of interfacial hydrogen bonds.1–5 The oriented arrangement of calcium carbonate platelets improves its tensile strength along the alignment direction.6 Ionic crosslinking strengthens the protein matrix and increases its ability to dynamically dissipate energy. The many interfacial hydrogen bonds promote stress transfer from the protein matrix to the oriented calcium carbonate platelets.3 These factors work together and lead to its extraordinary mechanical properties.7 Thus, natural nacre offers an ideal structural model for constructing high-performance composites.8,9
Up to date, there are many reports about nacre-mimetic materials with mimicking the layered structure of natural nacre.10–12 These layered composites are composed of clay,13–21 graphene oxide22–28 or alumina29–33 as two-dimensional inorganic platelets, and poly(vinyl alcohol),13,15,16,34 chitosan,21,35,36 polyurethane37,38 or cellulose15,39,40 as organic components. The assembly techniques include bottom-up layer-by-layer assembly,13,14,41 ice template assembly,31,32,42,43 vacuum-assisted filtration,17,36,44 evaporation-induced assembly15,16 and electrophoretic deposition.45,46 It has been demonstrated that interfacial adhesion between inorganic platelets and organic matrices is crucial to the mechanical properties of resultant layered composites.12 Interfacial hydrogen bond, ionic bond and covalent bond are usually designed and complemented to improve stress transfer efficiency at interface.11,12,47–51 In addition, the strength of organic matrices themselves is also important and can be enhanced through chemical or physical crosslinking.30 However, relative to large-size inorganic nanoplatelets (montmorillonite, graphene oxide, alumina typically has diameter more than 1 μm), small-size inorganic nanoplatelets with diameter less than 100 nm are rarely used to construct nacre-mimetic materials.
In this work, we choose small-size Ni–Al–NO3 layered double hydroxide (LDH) with a diameter of about 70 nm as inorganic nanoplatelets and sodium alginate (ALG) as organic component to construct nacre-mimetic layered composites with high transparency. The Ni–Al–NO3 LDH with positive charge on surface would interact with ALG through electrostatic and hydrogen-bonding interaction. Divalent calcium ion is introduced to strengthen ALG matrix through ionic cross-linking with the G units of ALG, forming “egg-box” structure.52–55 The effect of oriented arrangement of LDH, ionic crosslinking of ALG and interfacial interaction between LDH and ALG on the mechanical properties of hybrid film are studied. The resultant nacre-mimetic hybrid film has tensile strength as high as 194 MPa and excellent flexibility. In addition, the hybrid film exhibits high transparency because small size of LDH with diameter much less than visible light wavelength (400–900 nm) decreases light scattering. It is expected that the strong, flexible and transparent layered composite film has the potential as optical functional materials for a variety of applications, such as substrates for flexible displays, components for precision optical devices, windows for automobile and trains.
2. Experimental section
2.1. Materials
ALG was purchased from Guangdong Guanghua Chemical Factory Co. Ltd. Ni(NO3)2·6H2O, Al(NO3)3·9H2O and NaOH were purchased from Sinopharm Chemical Reagent Co. Ltd. Anhydrous CaCl2 was purchased from Tianjin Jinke Fine Chemical Research Institute. All materials were used as received. Polyamide filtration membrane (220 nm pore size) was purchased from Shanghai Xinya.
2.2. Fabrication of LDH/ALG-Ca2+ hybrid film
The fabrication process for LDH/ALG-Ca2+ artificial nacre is illustrated in Scheme 1. The Ni–Al–NO3 LDH powder was synthesized by the method that has been reported in our previous work.41,56 The synthesized LDH powder (0.1 g) was exfoliated in formamide (100 ml) by vigorously agitating for 24 h under N2 gas flow. The exfoliated LDH was mixed with a required amount of ALG solution with a concentration of 0.1 wt%, forming a homogeneous dispersion. Then, a required amount of CaCl2 aqueous solution with a concentration of 0.1 wt% was gradually instilled into the homogeneous dispersion and continually stirred for about 3 h, forming LDH/ALG-Ca2+ hybrid building blocks. The obtained mixture was filtrated under vacuum through polyamide filtration membrane, and dried under room temperature, leading to a transparent LDH/ALG-Ca2+ hybrid film. In the hybrid film, LDH contents was controlled by adjusting the mixing volume ratios of LDH dispersion to ALG aqueous solution. Ca2+ content relative to ALG was altered by adjusting the mixing volume ratio of CaCl2 solution to ALG solution (ESI, Table S1†).
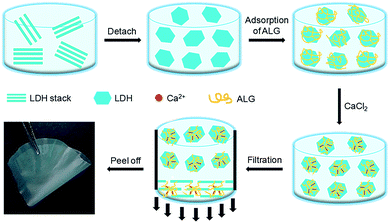 |
| Scheme 1 Fabrication process for LDH/ALG-Ca2+ hybrid film. The synthesized Ni–Al–NO3 LDH stacks are exfoliated mechanically, followed by mixing with ALG solution to form ALG-coated LDH. Then, the ALG-coated LDH is further crosslinked with Ca2+, leading to LDH/ALG-Ca2+ hybrid building blocks. Finally, the hybrid building blocks are assembled into transparent LDH/ALG-Ca2+ hybrid film through filtration. | |
2.3. Characterization
X-ray diffraction (XRD) was recorded by a Shimadzu XRD-6000 diffractometer under the following conditions: 40 kV, 40 mA and Cu Kα radiation. Fourier transform infrared (FTIR) spectra were conducted on an iN10MX FTIR instrument with the attenuated total reflection mode (ATR). Dynamic light scattering measurements and zeta potential tests were carried out on Malvern Zetasizer Nano ZS90. The light transmittance spectra were recorded in the range from 400 to 900 nm on a Shimadzu-3600 spectrophotometer. Scanning electron microscopy (SEM) was performed on a JEOL JSM7500FA field emission microscope. Tensile mechanical properties were tested using a Shimadzu AGS-X at a loading speed of 1 mm min−1 with a gauge length of 5 mm. All samples were cut into 10 mm in length and 3 mm in width.
3. Results and discussion
The synthesized Ni–Al–NO3 LDH was exfoliated through mechanical stirring, generating single-layer LDH nanoplatelets with positive charge on surface (ESI, Fig. S1†). The exfoliated LDH platelets have a diameter of about 70 nm and a thickness of about 0.9 nm, as measured by TEM and AFM (ESI, Fig. S2†). After mixed with ALG solution, the positively charged LDH nanoplatelets were coated by negatively charged ALG because of electrostatic and hydrogen-bonding interactions. Considering that ALG, a well known ionic polysaccharide, can chelate with divalent cations to form the “egg-box” structure.54,57 We use Ca2+ to crosslink ALG on the surface of LDH, forming LDH/ALG-Ca2+ hybrid building blocks. The hybrid building blocks were further processed into film through vacuum-assisted filtration. The ionic crosslinking would strengthen ALG and thus help to improve mechanical strength of hybrid film.17 After drying, the film was peeled from filtration membrane, leading to transparent, flexible and glossy LDH/ALG-Ca2+ hybrid film (Scheme 1).
3.1. Microstructure of LDH/ALG-Ca2+ hybrid film
To investigate the effect of LDH content on microstructure, six LDH/ALG-Ca2+ hybrid films with different LDH contents (5%, 10%, 20%, 30%, 40%, 50%) and constant mixing mass ratio of CaCl2 to ALG (0.5) were prepared. The microstructure of these hybrid films was examined by SEM, as shown in Fig. 1. For low LDH content (5%), it can be observed that the LDH platelets are homogeneously embedded into the ALG matrix without oriented arrangement (Fig. 1a). Differently, a layered structure appears when the LDH concentration is 10% (Fig. 1b). The sheet-like layers are parallel to the film surface and interpenetrated into adjacent layers, similar to previously reported nacre-mimetic hybrid films based on large-size inorganic platelets (clay,13,16,48 GO,36,44,58 Al2O3 (ref. 29, 31 and 59)). The full EDX spectrum of the cross section exhibits the signals originating from ALG (carbon and oxygen), LDH (nickel, aluminium) and calcium chloride (calcium) (ESI, Fig. S3†). These elements are uniformly distributed within the hybrid films, as proved by element mapping. However, when the LDH concentration is 20% or more, the layered structure disappears and is transformed to LDH aggregation (Fig. 1c–f). This is possibly attributed to the fact that high content of LDH nanoplatelets cannot be sufficiently covered by Ca2+-crosslinked ALG molecules.16 Thus, a part of bare LDH nanoplatelets aggregate together, leading to random distribution. The aggregation is also evidenced by X-ray diffraction (ESI, Fig. S4†). When the content of LDH is 20% or more, the diffraction peak position is the same as that of pure LDH. The aggregation is also reflected by dynamic light scattering measurement, which is carried out for LDH/ALG/CaCl2 solutions before filtration (ESI, Fig. S5†). For the mixing solution with high LDH content relative to ALG, the hydrodynamic diameter is obviously larger than those of exfoliated LDH dispersion and ALG solution.
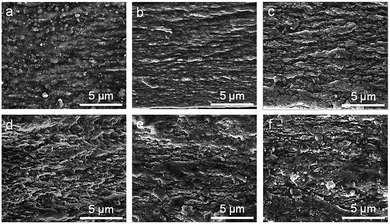 |
| Fig. 1 Cross-sectional SEM images of LDH/ALG-Ca2+ hybrid films with different LDH contents. (a) 5%, (b) 10%, (c) 20%, (d) 30%, (e) 40%, (f) 50%. | |
In order to investigate the effect of Ca2+ on microstructure, four LDH/ALG-Ca2+ hybrid film with different Ca2+ contents and constant LDH content of 10% were prepared. The effect of Ca2+ on the microstructure of hybrid films was examined by SEM, as shown in Fig. 2. For low ratio of CaCl2 to ALG (0.25, 0.5), the LDH/ALG-Ca2+ hybrid building blocks form a distinct layered structure, like that of natural nacre. Differently, when the mixing mass ratio of CaCl2 to ALG surpasses 0.5, the structure of LDH/ALG-Ca2+ hybrid film transforms from nacre-like layered arrangement to LDH aggregation. Such structure change is probably attributed to the fact that Ca2+ crosslinking affects the absorption of ALG on the surface of LDH nanoplatelets. Ca2+-crosslinked ALG has an “egg-box” structure, which increase the stiffness of ALG molecule chains and change their spatial configuration.54,57,60 The stiffening ALG at high Ca2+ content cannot sufficiently absorb onto the surface of LDH nanoplatelets, generating a part of bare LDH nanoplatelets. The insufficient absorption would decrease hydrogen bond and electrostatic interaction between ALG and LDH. As a result, these bare LDH nanoplatelets would aggregate together. The aggregation is also proved by X-ray diffraction, as shown in ESI, Fig. S6.† When the mixing mass ratio of CaCl2 to ALG is 1 or 2, the diffraction peak position is the same as that of pure LDH. The aggregation is also reflected by dynamic light scattering measurement, which is carried out for LDH/ALG/CaCl2 solutions before filtration (ESI, Fig. S7†). For the mixing solution with high mixing mass ratio of CaCl2 to ALG, the hydrodynamic diameter is obviously larger than those of exfoliated LDH dispersion and ALG solution.
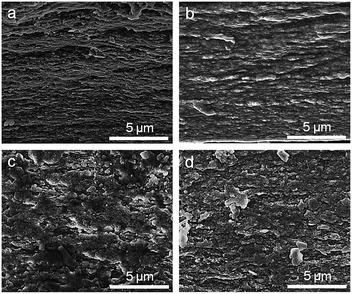 |
| Fig. 2 Cross-sectional SEM images of LDH/ALG-Ca2+ hybrid film with different mixing mass ratio of CaCl2 to ALG. (a) 0.25, (b) 0.5, (c) 1, (d) 2. For low mass ratio (0.25, 0.5), a layered structure are observed. For high mass ratio (1, 2), the structure is transformed to LDH aggregation. | |
3.2. Ionic crosslinking and interfacial interaction
The interfacial hydrogen bond and electrostatic interaction between LDH and ALG was proved by FTIR spectra, as shown in Fig. 3a. Pure ALG exhibits O–H stretching vibration in the wavenumber range of 3100–3600 cm−1 and C
O stretching vibration at 1751 cm−1. Pure Ni–Al–NO3 LDH exhibits O–H stretching vibration in the wavenumber range of 3100–3600 cm−1, interlayer water absorption peak at 1648 cm−1 and NO3− feature peak at 1353 cm−1. For LDH/ALG-Ca2+ hybrid film, the O–H stretching vibration is suppressed, due to the formation of hydrogen bond network between LDH and ALG.13,44 Because the negatively charged ALG, instead of NO3− and interlayer water, coats positively charged LDH, the absorption peak of interlayer water and NO3− disappears.56 In addition, the C
O stretching vibration at 1751 cm−1 was weakened, and a new C
O stretching vibration peak at 1686 cm−1 appears. This is due to that the carboxyl groups in M units of ALG form hydrogen bond with the hydroxyl groups on the surface of LDH, while the carboxyl groups in G units of ALG are crosslinked by Ca2+, stiffen and cannot form hydrogen bond with LDH (ESI, Fig. S8 and S9†).12
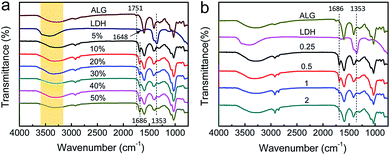 |
| Fig. 3 FTIR spectra of LDH/ALG-Ca2+ hybrid films. (a) Hybrid films with different LDH content and constant mass ratio of CaCl2 to ALG (0.5). (b) Hybrid films with constant LDH content (10%) and different mass ratio of CaCl2 to ALG. | |
The effect of Ca2+ crosslinking on interfacial hydrogen bond between LDH and ALG was investigated by FTIR spectra, as shown in Fig. 3b. At low ratio of CaCl2 to ALG (0.25, 0.5), interfacial hydrogen bond interaction induces the C
O stretching vibration of ALG to divide into two peaks, locating at 1751 and 1686 cm−1. At high ratio of CaCl2 to ALG (1, 2), the C
O stretching vibration peak at 1686 cm−1 obviously became weak.17,54 It suggests that Ca2+ crosslinks ALG for reinforcement at the cost of the decrease of hydrogen bond interaction between LDH and ALG. It is probably due to that overmuch Ca2+ crosslinking sharply increases the rigidness of ALG molecular chains, and thus impedes hydrogen bond formation between LDH and M units of ALG.35 So, the mixing mass ratio of CaCl2 to ALG has a optimal value of 0.5, at which ionic crosslinking of ALG by Ca2+ and interfacial hydrogen bond between LDH and ALG is finely balanced.
3.3. Properties of LDH/ALG-Ca2+ hybrid film
3.3.1. Mechanical property. The tensile properties of LDH/ALG-Ca2+ hybrid films with different LDH contents and constant mass ratio of CaCl2 to ALG (0.5) were measured to relate microstructure with mechanical property (Fig. 4a and b). In the range of 0–10%, the tensile strength sharply increases from 84 MPa for 5% LDH/ALG-Ca2+ to 194 MPa for 10% LDH/ALG-Ca2+. In this content range, ALG molecules are easily coated onto exfoliated LDH nanosheets to form hybrid building blocks by strong electrostatic and hydrogen bond interactions. The microstructure of hybrid films transforms from random distribution for 5% LDH/ALG-Ca2+ to nacre-like layered arrangement for 10% LDH/ALG-Ca2+, which leads to the dramatic increase of tensile strength. When LDH content is higher than 20%, a part of LDH nanoplatelets start to aggregate together and the layered structure disappears, which deteriorate tensile strength.
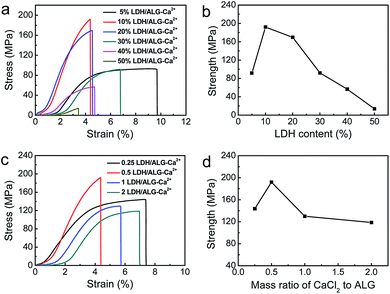 |
| Fig. 4 Mechanical properties of LDH/ALG-Ca2+ hybrid films. (a) Representative tensile stress–strain curves of the hybrid films with different LDH content. (b) The effect of LDH content on tensile strength of hybrid films, showing a maximum strength at 10% LDH. (c) Representative tensile stress–strain curves of the hybrid film with different mass ratio of CaCl2 to ALG. (d) The effect of the mass ratio of ALG to CaCl2 on tensile strength of hybrid films, showing a maximum strength at mass ratio of 0.5. | |
The tensile properties of LDH/ALG-Ca2+ hybrid films with different mixing mass ratio of CaCl2 to ALG and constant LDH content (10%) were measured to relate interfacial interaction with mechanical property (Fig. 4c and d). In general, Ca2+ crosslinking can increase the strength of ALG (ESI, Fig. S10†). When the mass ratio of CaCl2 to ALG increases from 0.25 to 0.5, Ca2+ crosslinking does not obviously weaken the hydrogen bond interaction between ALG and LDH. The synergism of hydrogen bonds and ionic crosslinking results in the increase of tensile strength from 142 MPa to 194 MPa. However, with further increase of the mass ratio of CaCl2 to ALG, the tensile strength decrease obviously. Although more Ca2+ strengths ALG through ionic crosslinking, the interfacial hydrogen bond interaction between LDH and ALG is weakened with a part of LDH nanoplatelets being aggregated together.
According to above-mentioned analysis, it can be found that layered structure, moderate ionic crosslinking of ALG by Ca2+ and plenty of hydrogen bond between LDH and ALG are the key to achieve high-strength LDH/ALG-Ca2+ hybrid films. 10% LDH/ALG-Ca2+ with mass ratio of CaCl2 to ALG being 0.5 meets all of these conditions and generate optimal synergism, leading to highest tensile strength of 194 MPa, 1.5 times higher than natural nacre (tensile strength of 80–135 MPa).7 Furthermore, the hybrid film is flexible and can be folded without risk of fracture.
3.3.2. Transparency. The nacre-mimetic LDH/ALG-Ca2+ hybrid film has high optical transparency, as shown in Fig. 5. In visible light wavelength range of 400 to 900 nm, the light transmittance is 70–94%, obviously higher than previously reported layered montmorillonite/chitosan hybrid film (60–80%) with similar thickness.21 The high optical transparency is mainly attributed to two reasons: small size of LDH nanoplatelets and ordered layered structure. The LDH nanoplatelets have a thickness of about 0.9 nm and a diameter of about 70 nm. The diameter is much smaller than visible light wavelength. Thus, the LDH is free from light scattering, make them acceptable for a variety of optical applications.61,62 The nacre-like layered structure also help to decrease light scattering between the nanoplatelets, which was demonstrated in nacre-mimetic montmorillonite/polymer hybrid films.15,21,48
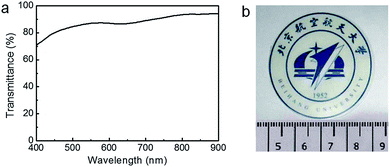 |
| Fig. 5 (a) Light transmittances and (b) photograph of 10% LDH/ALG-Ca2+ hybrid films. The thicknesses of film is about 20 μm. | |
4. Conclusions
Inspired by the relationship between structure and mechanical property of natural nacre, we prepared LDH/ALG-Ca2+ hybrid film by filtrating LDH/ALG-Ca2+ hybrid building blocks. The effect of the content of small-size LDH and ionic crosslinking of ALG by Ca2+ on the microstructure and interfacial interaction of LDH/ALG-Ca2+ hybrid films was studied. It was demonstrated that the cooperation of ionic crosslinking of ALG by Ca2+, interfacial hydrogen bonds between LDH and ALG, and alignment arrangement of LDH enable the nacre-mimetic hybrid film to posses a high strength of 194 MPa and good flexibility. Furthermore, the hybrid film exhibit high transparency of 70–94% in visible light range. We believe that these results offers an innovative insight into design and preparation of high performance bio-mimetic layered materials with high transparency for practical application.
Acknowledgements
This work was supported by the National Natural Science Foundation of China (51403008), 111 Project (B14009) and China Scholarship Council.
Notes and references
- A. P. Jackson, J. F. V. Vincent and R. M. Turner, Proc. R. Soc. London, Ser. A, 1986, 234, 415–440 CrossRef.
- G. Mayer, Science, 2005, 310, 1144–1147 CrossRef CAS PubMed.
- B. L. Smith, T. E. Schäffer, M. Viani, J. B. Thompson, N. A. Frederick, J. Kindt, A. Belcher, G. D. Stucky, D. E. Morse and P. K. Hansma, Nature, 1999, 399, 761–763 CrossRef CAS.
- H. D. Espinosa, J. E. Rim, F. Barthelat and M. J. Buehler, Prog. Mater. Sci., 2009, 54, 1059–1100 CrossRef CAS.
- F. Barthelat, H. Tang, P. D. Zavattieri, C. M. Li and H. D. Espinosa, J. Mech. Phys. Solids, 2007, 55, 306–337 CrossRef CAS.
- G. E. Padhwer and N. Beecher, Polym. Eng. Sci., 1970, 10, 185–192 Search PubMed.
- R. Z. Wang, Z. Suo, A. G. Evans, N. Yao and I. A. Aksay, J. Mater. Res., 2001, 16, 2485–2493 CrossRef CAS.
- J. Y. Sun and B. Bhushan, RSC Adv., 2012, 2, 7617–7632 RSC.
- J. Wang, Q. Cheng and Z. Tang, Chem. Soc. Rev., 2012, 41, 1111–1129 RSC.
- H. B. Yao, J. Ge, L. B. Mao, Y. X. Yan and S. H. Yu, Adv. Mater., 2014, 26, 163–188 CrossRef CAS PubMed.
- Q. Cheng, L. Jiang and Z. Tang, Acc. Chem. Res., 2014, 47, 1256–1266 CrossRef CAS PubMed.
- S. Wan, J. Peng, L. Jiang and Q. Cheng, Adv. Mater., 2016, 28, 7862–7898 CrossRef CAS PubMed.
- P. Podsiadlo, A. K. Kaushik, E. M. Arruda, A. M. Waas, B. S. Shim, J. D. Xu, H. Nandivada, B. G. Pumplin, J. Lahann, A. Ramamoorthy and N. A. Kotov, Science, 2007, 318, 80–83 CrossRef CAS PubMed.
- Z. Tang, N. A. Kotov, S. Magonov and B. Ozturk, Nat. Mater., 2003, 2, 413–418 CrossRef CAS PubMed.
- J. F. Wang, Q. F. Cheng, L. Lin and L. Jiang, ACS Nano, 2014, 8, 2739–2745 CrossRef CAS PubMed.
- J. Wang, Q. Cheng, L. Lin, L. Chen and L. Jiang, Nanoscale, 2013, 5, 6356–6362 RSC.
- B. L. Liang, H. W. Zhao, Q. Zhang, Y. Z. Fan, Y. H. Yue, P. G. Yin and L. Guo, ACS Appl. Mater. Interfaces, 2016, 8, 28816–28823 CAS.
- T. Verho, M. Karesoja, P. Das, L. Martikainen, R. Lund, A. Alegria, A. Walther and O. Ikkala, Adv. Mater., 2013, 25, 5055–5059 CrossRef CAS PubMed.
- B. L. Zhu, N. Jasinski, A. Benitez, M. Noack, D. Park, A. S. Goldmann, C. Barner-Kowollik and A. Walther, Angew. Chem., Int. Ed., 2015, 54, 8653–8657 CrossRef CAS PubMed.
- P. Das, J.-M. Malho, K. Rahimi, F. H. Schacher, B. Wang, D. E. Demco and A. Walther, Nat. Commun., 2015, 6, 5967 CrossRef PubMed.
- H.-B. Yao, Z.-H. Tan, H.-Y. Fang and S.-H. Yu, Angew. Chem., Int. Ed., 2010, 49, 10127–10131 CrossRef CAS PubMed.
- Y. Y. Zhang, Y. C. Li, P. Ming, Q. Zhang, T. X. Liu, L. Jiang and Q. F. Cheng, Adv. Mater., 2016, 28, 2834–2839 CrossRef CAS PubMed.
- S. Gong, L. Jiang and Q. Cheng, J. Mater. Chem. A, 2016, 4, 17073–17079 CAS.
- Q. Cheng, M. Wu, M. Li, L. Jiang and Z. Tang, Angew. Chem., Int. Ed., 2013, 52, 3750–3755 CrossRef CAS PubMed.
- M. Zhang, L. Huang, J. Chen, C. Li and G. Q. Shi, Adv. Mater., 2014, 26, 7588–7592 CrossRef CAS PubMed.
- L. Huang, C. Li, W. Yuan and G. Shi, Nanoscale, 2013, 5, 3780–3786 RSC.
- Y. Q. Li, T. Yu, T. Y. Yang, L. X. Zheng and K. Liao, Adv. Mater., 2012, 24, 3426–3431 CrossRef CAS PubMed.
- L. Q. Liu, Y. Gao, Q. Liu, J. Kuang, D. Zhou, S. T. Ju, B. H. Han and Z. Zhang, Small, 2013, 9, 2466–2472 CrossRef CAS PubMed.
- J. Wang, J. Qiao, J. Wang, Y. Zhu and L. Jiang, ACS Appl. Mater. Interfaces, 2015, 7, 9281–9286 CAS.
- L. J. Bonderer, A. R. Studart and L. J. Gauckler, Science, 2008, 319, 1069–1073 CrossRef CAS PubMed.
- E. Munch, M. E. Launey, D. H. Alsem, E. Saiz, A. P. Tomsia and R. O. Ritchie, Science, 2008, 322, 1516–1520 CrossRef CAS PubMed.
- H. Zhao, Y. Yue, L. Guo, J. Wu, Y. Zhang, X. Li, S. Mao and X. Han, Adv. Mater., 2016, 28, 5099–5105 CrossRef CAS PubMed.
- L. J. Bonderer, A. R. Studart, J. Woltersdorf, E. Pippel and L. J. Gauckler, J. Mater. Res., 2009, 24, 2741–2754 CrossRef CAS.
- Y. Q. Shu, P. G. Yin, B. L. Liang, H. Wang and L. Guo, ACS Appl. Mater. Interfaces, 2014, 6, 15154–15161 CAS.
- P. Podsiadlo, Z. Tang, B. S. Shim and N. A. Kotov, Nano Lett., 2007, 7, 1224–1231 CrossRef CAS PubMed.
- S. J. Wan, J. S. Peng, Y. C. Li, H. Hu, L. Jiang and Q. F. Cheng, ACS Nano, 2015, 9, 9830–9836 CrossRef CAS PubMed.
- R. Libanori, F. H. L. Munch, D. M. Montenegro and A. R. Studart, Compos. Sci. Technol., 2012, 72, 435–445 CrossRef CAS.
- L. J. Bonderer, K. Feldman and L. J. Gauckler, Compos. Sci. Technol., 2010, 70, 1966–1972 CrossRef CAS.
- H. Sehaqui, J. Kochumalayil, A. D. Liu, T. Zimmermann and L. A. Berglund, ACS Appl. Mater. Interfaces, 2013, 5, 7613–7620 CAS.
- A. Liu, A. Walther, O. Ikkala, L. Belova and L. A. Berglund, Biomacromolecules, 2011, 12, 633–641 CrossRef CAS PubMed.
- Y. Q. Shu, P. G. Yin, B. L. Liang, S. S. Wang, L. C. Gao, H. Wang and L. Guo, J. Mater.
Chem., 2012, 22, 21667–21672 RSC.
- S. Deville, E. Saiz, R. K. Nalla and A. P. Tomsia, Science, 2006, 311, 515–518 CrossRef CAS PubMed.
- L.-B. Mao, H.-L. Gao, H.-B. Yao, L. Liu, H. Cölfen, G. Liu, S.-M. Chen, S.-K. Li, Y.-X. Yan, Y.-Y. Liu and S.-H. Yu, Science, 2016, 354, 107–110 CrossRef CAS PubMed.
- P. Ming, Z. F. Song, S. S. Gong, Y. Y. Zhang, J. L. Duan, Q. Zhang, L. Jiang and Q. F. Cheng, J. Mater. Chem. A, 2015, 3, 21194–21200 CAS.
- B. Long, C.-A. Wang, W. Lin, Y. Huang and J. Sun, Compos. Sci. Technol., 2007, 67, 2770–2774 CrossRef CAS.
- W. Lin, C.-a. Wang, H. Le, B. Long and Y. Huang, Mater. Sci. Eng., C, 2008, 28, 1031–1037 CrossRef CAS.
- J. F. Wang, L. Lin, Q. F. Cheng and L. Jiang, Angew. Chem., Int. Ed., 2012, 51, 4676–4680 CrossRef CAS PubMed.
- A. Walther, I. Bjurhager, J.-M. Malho, J. Pere, J. Ruokolainen, L. A. Berglund and O. Ikkala, Nano Lett., 2010, 10, 2742–2748 CrossRef CAS PubMed.
- A. Walther, I. Bjurhager, J.-M. Malho, J. Ruokolainen, L. Berglund and O. Ikkala, Angew. Chem., Int. Ed., 2010, 49, 6448–6453 CrossRef CAS PubMed.
- P. Podsiadlo, A. K. Kaushik, B. S. Shim, A. Agarwal, Z. Tang, A. M. Waas, E. M. Arruda and N. A. Kotov, J. Phys. Chem. B, 2008, 112, 14359–14363 CrossRef CAS PubMed.
- P. Podsiadlo, Z. Q. Liu, D. Paterson, P. B. Messersmith and N. A. Kotov, Adv. Mater., 2007, 19, 949–955 CrossRef CAS.
- H. Zhu, Q. Zhang and S. P. Zhu, ACS Appl. Mater. Interfaces, 2016, 8, 17395–17401 CAS.
- C. Menakbi, F. Quignard and T. Mineva, J. Phys. Chem. B, 2016, 120, 3615–3623 CrossRef CAS PubMed.
- J. Y. Sun, X. H. Zhao, W. R. K. Illeperuma, O. Chaudhuri, K. H. Oh, D. J. Mooney, J. J. Vlassak and Z. G. Suo, Nature, 2012, 489, 133–136 CrossRef CAS PubMed.
- Y. A. Morch, I. Donati, B. L. Strand and G. Skjak-Braek, Biomacromolecules, 2006, 7, 1471–1480 CrossRef CAS PubMed.
- Y. Q. Shu, P. G. Yin, J. F. Wang, B. L. Liang, H. Wang and L. Guo, Ind. Eng. Chem. Res., 2014, 53, 3820–3826 CrossRef CAS.
- G. L. Du, F. X. Wu, Y. Cong, L. Nie, S. H. Liu, G. R. Gao and J. Fu, Chem. Commun., 2015, 51, 15534–15537 RSC.
- S. J. Wan, H. Hu, J. S. Peng, Y. C. Li, Y. Z. Fan, L. Jiang and Q. F. Cheng, Nanoscale, 2016, 8, 5649–5656 RSC.
- S. Park, K.-S. Lee, G. Bozoklu, W. Cai, S. T. Nguyen and R. S. Ruoff, ACS Nano, 2008, 2, 572–578 CrossRef CAS PubMed.
- Y. Zhuang, F. Yu, H. Chen, J. Zheng, J. Ma and J. Chen, J. Mater. Chem. A, 2016, 4, 10885–10892 CAS.
- M. Nogi, S. Iwamoto, A. N. Nakagaito and H. Yano, Adv. Mater., 2009, 21, 1595–1598 CrossRef CAS.
- H. Yano, J. Sugiyama, A. N. Nakagaito, M. Nogi, T. Matsuura, M. Hikita and K. Handa, Adv. Mater., 2005, 17, 153–155 CrossRef CAS.
Footnote |
† Electronic supplementary information (ESI) available. See DOI: 10.1039/c7ra04577h |
|
This journal is © The Royal Society of Chemistry 2017 |