DOI:
10.1039/C7RA04376G
(Paper)
RSC Adv., 2017,
7, 35558-35564
Lightweight, interconnected VO2 nanoflowers hydrothermally grown on 3D graphene networks for wide-voltage-window supercapacitors†
Received
18th April 2017
, Accepted 12th July 2017
First published on 17th July 2017
Abstract
Highly stable and interconnected VO2 nanoflowers were uniformly grown on flexible three dimensional graphene networks, which directly served as a lightweight and high conductivity supercapacitor electrode (VO2 NF@3DG). The uniform VO2 NF@3DG hybrid provided direct and stable pathways for rapid electron and ion transport. The hybrid produced an improved areal specific capacitance of 466 mF cm−2 and 283.2 mF cm−2 for the three- and the two-electrode configurations, respectively. A capacitance retention of 63.5% after 3000 cycles showed that the VO2 NF@3DG hybrid had a stable cycling performance at a high specific capacitance. A high energy density of 279.6 mW h m−2 and a high power density of 60
000 mW m−2 were achieved in symmetrical supercapacitors. The effective strategy could be applied to improve the performance of supercapacitors with high efficiency, wide potential windows and long life.
1. Introduction
Energy storage technologies have attracted global attention emanating from the ever-growing demands for renewable energy and the environmental crisis. Supercapacitors, as an essential energy storage device, have been studied because of their high-power density, long cycle life and fast charge/discharge rate.1–3 Typically, supercapacitors can be classified as electric double-layer capacitors (EDLCs) and pseudocapacitors.4–6 Pseudocapacitors with metal oxide or conducting polymer electrodes offer a better capacitive behavior, and store energy by employing a fast-reversible redox reaction at the interfaces between the electrodes and electroactive species in the electrolyte.7–9 In general, the most important factor is the electrode materials, which influence the capacitive behavior of supercapacitors. Various transition-metal oxides have been studied as electrode materials for pseudocapacitors, such as MoO3,10 MnO2,11 Fe2O3,12 VO2,13 and Co3O4.14
Among various oxides, vanadium oxides has been suggested as one of the most promising pseudocapacitance electrode materials due to its high energy density, low cost, and wide potential windows, arising from vanadium multivalent states (V2+, V3+, V4+, and V5+).15,16 Based on the basic principle of energy storage, the nanostructures of vanadium oxides become an inevitable way to improve the performance of supercapacitors. The various nanostructures of vanadium oxides for supercapacitors had been realized, such as nanobelts,17 nanoflakes,18 nanowires,19 nanotextiles.20 However, vanadium oxides did not usually deliver ideal specific capacitance behavior because of its low electrical conductivity and the poor structural stability.21 Therefore, a strategy has been employed to be a hybrid by introducing excellent conductive materials with high structure stability into vanadium oxides, such as graphene. Graphene has a unique superior electrical conductivity, large specific surface area, high mechanical flexibility and chemical stability.22
In this work, three dimensional graphene (3DG) networks grown by chemical vapor deposition (CVD) acted as a current collector, VO2 nanoflowers were hydrothermally anchored on 3DG networks (VO2 NF@3DG) using dodecylamine as a reducing agent. As a binder-free, conductive-agent-free and self-supported supercapacitor electrode, the VO2 NF@3DG hybrid had been investigated the electrochemical performances in detail. The results showed that the VO2 NF@3DG electrode possessed high areal specific capacity (466 mF cm−2), outstanding cycling stability (the retention of 63.5% after 3000 cycles) and high energy densities (279.6 mW h m−2).
2. Experimental section
A schematic diagram of preparation process for the VO2 NF@3DG hybrid electrode was illustrated in Fig. 1. Above all, 3DG was synthesized by CVD technique, which had been described in our previous reports.23,24 In brief, Ni foam slice (110 pores per inch, ∼380 g m−2 and ∼1.5 mm thick, pressed to ∼0.5 mm) was heated up to 1000 °C in a tube furnace under H2/Ar ambience. Ethanol was introduced by flowing H2/Ar (20 sccm/100 sccm) gas to the furnace under atmospheric pressure. After 20 min reaction, the quartz tube was quickly cooled down to room temperature, 3DG grown on Ni foam slice was obtained. Afterwards, the samples were eliminated by 3 M HCl aqueous solution for 6 h at 60 °C to gain lightweight free-supported 3DG networks.
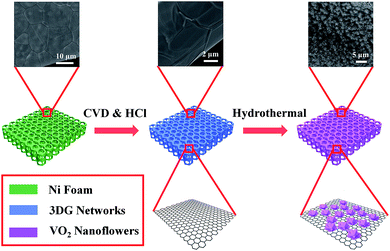 |
| Fig. 1 The schematic diagram of preparation process for VO2 NF@3DG hybrid. | |
VO2 nanoflowers were synthesized on 3DG networks by a simple hydrothermal method. Initially, 0.5 g of V2O5 was dissolved in 25 ml deionized water followed by slow addition of 25 ml hydrogen peroxide (30%) solution with strongly string for 3 h at 0 °C. The resultant solution (V2O5·nH2O) was obtained after standing at ambient temperature for 12 hours. In this process, the chemical reactions was as follows:
|
V2O5 + H2O2 → 2HVO4 + H2O
| (1) |
|
HVO4 + (n − 1)H2O → V2O5·nH2O + O2
| (2) |
Then, 0.255 g of dodecylamine was dissolved in 2 ml ethanol with strongly string for 1 h and added drop wise to the above-prepared solution. The resultant solution was stirred for 24 hours at 0 °C, and then transferred into a 50 ml Teflon-liner autoclave within a piece of 3DG networks. The reaction was completed at 180 °C for different time (12, 24, 36, 48 h). Finally, the samples were washed with deionized water for several times, dried at 60 °C for 8 h. The lightweight VO2 NF@3DG hybrid were obtained.
The morphology of the samples was characterized by field emission scanning electron microscopy (FE-SEM, TESCAN MIRA3 XMU). The microstructure was characterized using high resolution transmission electron microscopy (HRTEM, FEI Tecnai F30, operated at 300 kV). The crystal structures were examined by X-ray diffraction (XRD, Philips, X'pert pro, Cu Kα, 0.154056 nm) and Raman spectroscopy (JY-HR800 micro-Raman, using a 532 nm wavelength YAG laser with a laser spot diameter of ∼600 nm). The mass of active materials was measured by a microbalance (Mettler Toledo, XSE) with an accuracy of 0.01 mg. The loaded quantity was obtained by the mass difference of the samples between the before and the after hydrothermal reaction. The mass of VO2 nanoflowers on 3D graphene was around 0.91 mg cm−2. 3DG has the mass density of ∼8.22 mg cm−2. Electrochemical measurements (CHI 660E) were accomplished in three-electrode and two-electrode configurations at room temperature in a 0.5 M K2SO4 aqueous electrolyte. A platinum sheet was used as counter electrode, and a saturated calomel electrode (SCE) was used as reference electrode. The VO2 NF@3DG hybrid was directly utilized as the working electrode. The nominal area of the VO2 NF@3DG hybrid immersed into the electrolyte was controlled to be around 1 cm × 1 cm. Electrochemical impedance spectroscopy (EIS) was applied with 5 mV AC perturbation amplitude in the frequency range from 1 MHz to 0.01 Hz. The areal-specific capacitance (Ca, mF cm−2) of the three-electrode configuration was calculated by the following equation.
|
 | (3) |
where,
I is the charge–discharge current (A),
t is the discharge time (s),
S is the electrode area and Δ
V is the potential (V).
3. Results and discussion
Fig. 1 shows the schematic diagram of preparation for VO2 NF@3DG hybrid, which demonstrates the two procedures, including the growth of lightweight self-supported 3DG networks by combining CVD and HCl corrosion, and the anchoring of VO2 nanoflowers by hydrothermal process. Fig. 2 shows the typical SEM and TEM images of VO2 nanoflowers, which are interconnected and distributed on 3DG networks. (1) The nanoflowers were made of small VO2 nanobelts. Fig. S1† shows the SEM images of VO2 nanoflowers grown at different hydrothermal time. More details can be seen in the high-resolution TEM image in Fig. 2d. The lattice fringe with a spacing distance of 0.186 nm was indexed to the (312) crystal planes of VO2 phase (JCPDS card no. 31-1438). Fig. 2e shows the crystal phase and structure information of the VO2 NF@3DG hybrid, VO2 NF and 3DG. The XRD peaks of VO2 NF were all indexed to VO2 phase (JCPDS card no. 31-1438). A sharp diffraction peak located at 49.496° was clearly visible and assigned to VO2 (312) reflections, which was consistent with the HRTEM result. Therefore, VO2 NF@3DG hybrid has been prepared successfully. The micro-zone Raman spectra of VO2 NF@3DG hybrid, VO2 NF and 3DG have been shown in Fig. 2f. The Raman spectrum of VO2 NF displayed the bending vibrations of V
O, the triply coordinated oxygen of V3–O, the doubly coordinated oxygen of V2–O and terminal V
O bond, which were identified to the Raman-shift peaks of 183, 265, 527, 693 and 923 cm−1.13,25 The Raman spectrum of 3DG networks shows a G peak at 1580 cm−1 and a 2D peak at 2716 cm−1. The G peak originated from the vibration of carbon atoms in the hexagonal graphene lattice, and the 2D peak corresponded to the second order of zone-boundary phonons. The lack of D peak for graphene suggested few defects and the high quality 3DG networks, which could shorten transport paths for ions and electrons, amplify the contact area with electrolyte, and then elevate the utilization of VO2 pseudo-capacitance materials.26,27
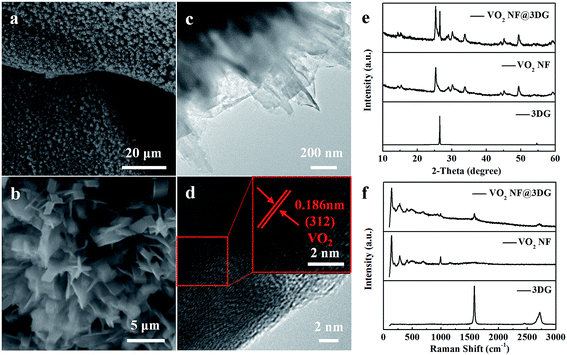 |
| Fig. 2 (a, b) SEM and (c, d) TEM images of VO2 NF@3DG hybrid. (e) XRD pattern and (f) micro-zone Raman spectrum of VO2 NF@3DG hybrid, VO2 NF and 3DG. | |
A typical XPS spectrum for the VO2 NF@3DG hybrid (Fig. 3a) indicates the existence of C, O and V elements. The high-resolution XPS spectrum for C can be deconvoluted into three peaks (Fig. 3b), where the peaks at 284.8, 286 and 288.5 eV correspond to the reported binding energy for C–O (sp2-hybridized carbon), C–O and C
O.28,29 The corresponding O1s spectrum is shown in Fig. 3b. The O1s spectrum is broad and asymmetric, which can be deconvoluted into three peaks, indicating the existence of three different oxygen species. The peaks located at the binding energy of 530, 531 eV are attributed to the V–O linkage of VO2 and V2O5, while the peaks at 532.2 is due to OH−.30,31 The V2p3/2 spectrum can be deconvoluted into two peaks (Fig. 3d), where the peaks at 516.3 and 517.2 eV correspond to the reported binding energy of V2p3/2 for V4+ and V5+, respectively.32 The V2p1/2 peak is located at 524.1 eV. The existence of V5+ might result from the surface oxidation of the samples in air. The same phenomenon had also been reported by other groups.31,33 These results further proved that the nanostructures of VO2 had been successfully grown on the surface of graphene.
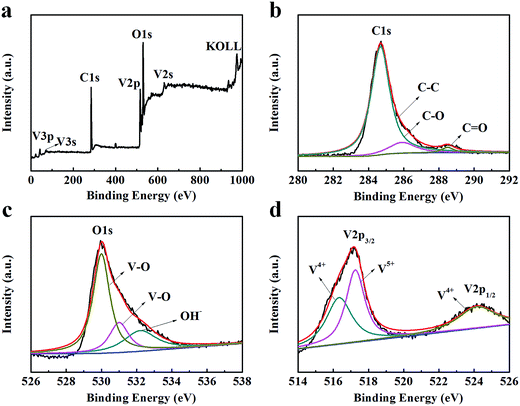 |
| Fig. 3 XPS spectra of VO2 NF@3DG hybrid. (a) Survey spectrum. High-resolution XPS spectra of (b) C, (c) O and (d) V element. | |
Electrochemical measurements were carried out to verify the potential application of VO2 NF@3DG electrode materials. Fig. 4a shows typical cyclic voltammetry (CV) curves of VO2 NF@3DG hybrids prepared at different hydrothermal time (12, 24, 36, and 48 h). It can be clearly seen that the CV curves, at the scan rate of 5 mV s−1, exhibit an approximate rectangular shape with small redox peaks. Fig. S2† shows the CV curves of VO2 NF@3DG hybrids and 3DG electrodes. These indicated the faradaic pseudocapacitance behaviors of the hybrid electrodes, which were caused by the electrochemical K+ insertion procedure as follows:13,34
|
VO2 + xK+ + xe− ↔ 4KxVO2
| (4) |
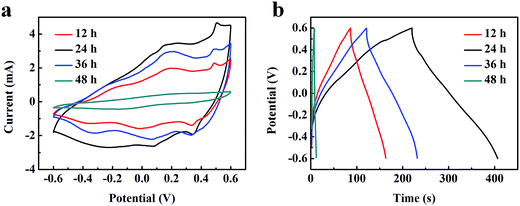 |
| Fig. 4 (a) CV and (b) GCD curves of VO2 NF@3DG hybrid electrodes prepared at different hydrothermal time (12, 24, 36 and 48 h). | |
In terms of the area of CV curves, related to the capacitance value, the VO2 NF@3DG synthesized for 24 h had the larger, which represented the more excellent capacitance behavior. Fig. 4b showed galvanostatic charge–discharge (GCD) curves of VO2 NF@3DG electrodes synthesized at different hydrothermal time with a current density of 3 mA cm−2. The areal specific capacitances of VO2 NF@3DG hybrid electrodes at different hydrothermal time (12, 24, 36, and 48 h) were 11.3, 466.5, 277.5, 192.5 mF cm−2 respectively. All the results indicated that the VO2 NF@3DG hybrid with hydrothermal 24 h had the most excellent electrochemical performance. So the VO2 NF@3DG hybrid grown for 24 h was further investigated in the following.
Fig. 5a showed the CV curves of VO2 NF@3DG hybrid prepared for 24 h at different sweep rates. The capacitive current was enhanced with the increased scan rate, indicating an excellent electrochemical reversibility and the fast diffusion of the electrolyte ions into the VO2 NF@3DG hybrid electrode. The GCD was carried out at different current densities (Fig. 5b). The calculated areal capacitance Ca of VO2 NF@3DG hybrid was 466 mF cm−2 (507 F g−1), 278 mF cm−2 (302 F g−1), 240 mF cm−2 (261 F g−1), 191 mF cm−2 (208 F g−1) at 3, 5, 8, 10 mA cm−2, respectively (Fig. 5c). The ∼41% Ca was remained with the increasing current density from 3 to 10 mA cm−2. The rate performance was better than the reported results. Nie et al. obtained 27.3% rate capability of VO2@PANi coaxial nanobelts (from 0.5 A g−1 to 5.0 A g−1),25 Zheng et al. fabricated V2O3@C core–shell composites and get 32.3% rate performance (0.1 A g−1 to 10 A g−1).35 Li et al. obtained the rate of 39% for rGO coated V2O5 microspheres (from 1 A g−1 to 20 A g−1) as the supercapacitor electrodes.36
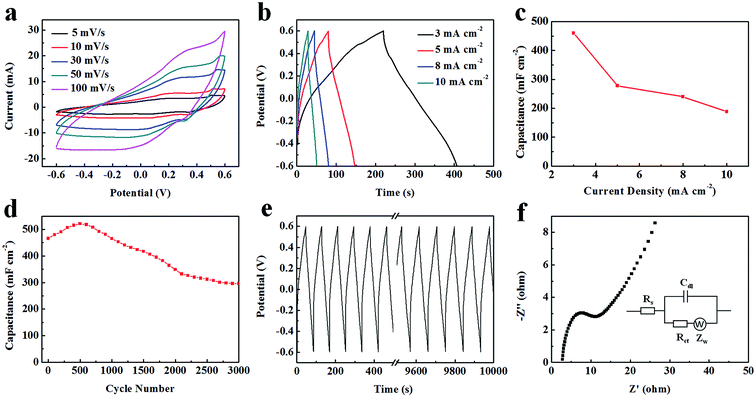 |
| Fig. 5 (a) CV and (b) GCD curves of VO2 NF@3DG hybrid electrode, (c) the areal specific capacitance of VO2 NF@3DG as a function of current density. (d) Electrochemical cycling performance, and (e) the charge–discharge curves during electrochemical cycling process of VO2 NF@3DG hybrid. (f) Nyquist plot and equivalent circuit diagram (the inset) of VO2 NF@3DG hybrid. | |
Electrochemical cycle of VO2 NF@3DG hybrid electrode was further carried out, which was shown in Fig. 5e, with the increase of cycles, the areal-specific capacitance remained 63.5% after 3000 cycles, compared to the initial Ca (466 mF cm−2), which reflected a good cycling performance of VO2 NF@3DG hybrid electrode. The increase of the Ca at first 500 cycles could be due to the improved wettability and activation process of the electrodes. In Table 1, our results are compared with other results on the electrochemical performances of supercapacitor electrodes.13,25,36–40 It can be clearly seen that the capacitance and cycle stability of the VO2 NF@3DG hybrid electrode are comparable or better than those in other works. What's more, as seen from the EIS curve (Fig. 5f), the intersection of the curve at the real axis indicates the resistance of the electrochemical system at the high frequency, and the semicircle diameter reflects the charge-transfer resistance (Rct).41,42 Through calculating, the equivalent series resistance (Rs) was a low value of 2.918 Ω, and the Rct value was 0.468 Ω. In the low frequency range, the slope of the curve approaching 90° suggests negligible diffusive resistance for the VO2 NF@3DG hybrid. Therefore, these results confirms that the VO2 NF@3DG hybrid as the supercapacitor electrode has excellent electrochemical performances.
Table 1 The electrochemical performance comparison of our results with other works
Material |
Electrolyte |
Current density |
Specific capacitance |
Stability (cycle) |
Ref. |
V4O9 yolk–shell microspheres |
1.5 M KOH |
0.5 A g−1 |
392 F g−1 |
75% (2000) |
37 |
VO2@PANi nanobelts |
0.5 M Na2SO4 |
0.5 A g−1 |
246 F g−1 |
28.6% (1000) |
25 |
Graphene/VO2 nanobelts |
0.5 M K2SO4 |
1 A g−1 |
426 F g−1 |
82% (5000) |
13 |
Graphene/VO2 particles |
0.5 M K2SO4 |
0.25 A g−1 |
225 F g−1 |
81% (1000) |
38 |
Graphene/VOx nanotubes |
1 M Na2SO4 |
1 A g−1 |
210 F g−1 |
48% (5000) |
39 |
V2O5 3D nanosheets |
1 M Na2SO4 |
0.5 A g−1 |
451 F g−1 |
90% (4000) |
40 |
V2O5 microspheres/rGO |
8 M LiCl |
1 A g−1 |
537 F g−1 |
84% (1000) |
36 |
VO2 nanoflowers@3DG |
0.5 M K2SO4 |
∼3.3 A g−1 |
507 F g−1 |
63.5% (3000) |
Our work |
To check the practical electrochemical behaviors of the VO2 NF@3DG hybrid in devices, a symmetrical supercapacitor was assembled by using two pieces of VO2 NF@3DG hybrids. Fig. 6a shows typical CV curves of two-electrode configuration at various scan rates for potentials between −0.6 and 0.6 V. The obtained CV curves exhibit rectangular-like shapes without obvious redox peaks, indicating an ideal capacitive behavior. The GCD measurements at different current densities were measured and shown in Fig. 6b. Herein, the areal-specific capacitance (Cas, mF cm−2), energy density (E), and power density (P) of the two-electrode configuration were calculated according to the eqn (5)–(7), respectively.43,44
|
 | (5) |
|
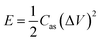 | (6) |
|
 | (7) |
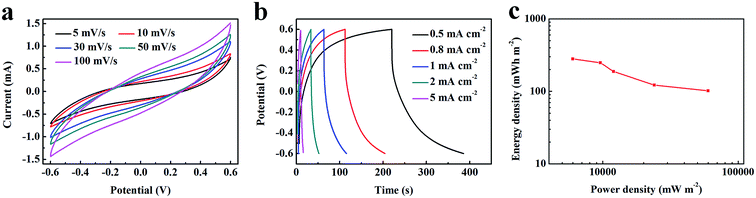 |
| Fig. 6 (a) CV curves, (b) GCD curves and (c) Ragone plot of the symmetrical supercapacitor device assembled by two pieces of VO2 NF@3DG hybrids. | |
The supercapacitor device exhibited an areal specific capacitance of 70.8 mF cm−2 at 0.5 mA cm−2. The calculated results are shown in the Ragone plots (Fig. 6c). It is found that the symmetrical supercapacitors exhibits a high energy density of 279.6 mW h m−2 at a power density of 6000 mW m−2 and a high power density of 60
000 mW m−2 at a energy density of 102 mW h m−2. Therefore, the measurement results in two-electrode configuration have proved the possibility of the practical application for the VO2 NF@3DG hybrid as the supercapacitor electrode.
4. Conclusions
In this work, the lightweight, flexible and interconnected VO2 nanoflowers was successfully grown on 3DG networks with high specific surface area and high conductivity, directly served as supercapacitor electrodes. The large specific capacitance (466 mF cm−2), long cycling stability (63.5% after 3000 cycles), high energy density (279.6 mW h m−2) and high power density (60
000 mW m−2) were achieved in the VO2 NF@3DG hybrid electrodes. This study provides an effective strategy to improve the performance of electrode materials for supercapacitors with a wide potential windows and long life, which makes them promising candidates for future energy-storage applications.
Acknowledgements
This work was supported by Natural Science Foundation of Gansu Province (No. 1208RJZA199), the fund of the State Key Laboratory of Advanced Processing and Recycling of Non-ferrous Metals, Lanzhou University of Technology (SKLAB02014003), the Fundamental Research Funds for the Central Universities (lzujbky-2017-k21), and the Project-sponsored by SRF for ROCS, SEM.
References
- E. Frackowiak and F. Béguin, Carbon materials for the electrochemical storage of energy in capacitors, Carbon, 2001, 39, 937–950 CrossRef CAS.
- Y. Zhu, S. Murali, M. D. Stoller, K. J. Ganesh, W. Cai, P. J. Ferreira, A. Pirkle, R. M. Wallace, K. A. Cychosz, M. Thommes, D. Su, E. A. Stach and R. S. Ruoff, Carbon-based supercapacitors produced by activation of graphene, Science, 2011, 332, 1537–1541 CrossRef CAS PubMed.
- F. Béguin, V. Presser, A. Balducci and E. Frackowiak, Carbons and electrolytes for advanced supercapacitors, Adv. Mater., 2014, 26, 2219–2251 CrossRef PubMed.
- F. Wolfart, D. P. Dubal, M. Vidotti and P. Gómez-Romero, Hybrid core–shell nanostructured electrodes made of polypyrrole nanotubes coated with Ni(OH)2 nanoflakes for high energy-density supercapacitors, RSC Adv., 2016, 6, 15062–15070 RSC.
- S. Liu, K. S. Hui and K. N. Hui, Flower-like Copper Cobaltite Nanosheets on Graphite Paper as High-Performance Supercapacitor Electrodes and Enzymeless Glucose Sensors, ACS Appl. Mater. Interfaces, 2016, 8, 3258–3267 CAS.
- S. Zhang, H. Gao, M. Huang and J. Zhou, One-step hydrothermal synthesis of nitrogen doping graphene based cobalt oxide and its supercapacitive properties, J. Alloys Compd., 2017, 705, 801–805 CrossRef CAS.
- J. Patiño, N. López-Salas, M. C. Gutiérrez, D. Carriazo and M. L. Ferrer, Phosphorus-doped carbon–carbon nanotube hierarchical monoliths as true three-dimensional electrodes in supercapacitor cells, J. Mater. Chem. A, 2016, 4, 1251–1263 Search PubMed.
- X. T. Hong, K. S. Hui, Z. Zeng, K. N. Hui, L. J. Zhang, M. Y. Mo and M. Li, Hierarchical nitrogen-doped porous carbon with high surface area derived from endothelium corneum gigeriae galli for high-performance supercapacitor, Electrochim. Acta, 2014, 130, 464–469 CrossRef CAS.
- Y. Qian, R. Liu, Q. F. Wang, J. Xu, D. Chen and G. Z. Shen, Efficient synthesis of hierarchical NiO nanosheets for high-performance flexible all-solid-state supercapacitors, J. Mater. Chem. A, 2014, 2, 10917–10922 CAS.
- Q. Mahmood, H. J. Yun, W. S. Kim and H. S. Park, Highly uniform deposition of MoO3 nanodots on multiwalled carbon nanotubes for improved performance of supercapacitors, J. Power Sources, 2013, 235, 187–192 CrossRef CAS.
- L. Yuan, X. H. Lu, X. Xiao, T. Zhai, J. Dai, F. Zhang, B. Hu, X. Wang, L. Gong, J. Chen, C. Hu, Y. Tong, J. Zhou and Z. Wang, Flexible solid-state supercapacitors based on carbon nanoparticles/MnO2 nanorods hybrid structure, ACS Nano, 2012, 6, 656–661 CrossRef CAS PubMed.
- C. Guan, J. Liu, Y. Wang, L. Mao, Z. Fan, Z. Shen, H. Zhang and J. Wang, Iron oxide-decorated carbon for supercapacitor anodes with ultrahigh energy density and outstanding cycling stability, ACS Nano, 2015, 9, 5198–5207 CrossRef CAS PubMed.
- H. Wang, H. Yi, X. Chen and X. Wang, One-step strategy to three-dimensional graphene/VO2 nanobelt composite hydrogels for high performance supercapacitors, J. Mater. Chem. A, 2014, 2, 1165–1173 CAS.
- J. Wang, W. Dou, X. Zhang, W. Han, X. Mu, Y. Zhang, X. Zhao, Y. Chen, Z. Yanga, Q. Su, E. Xie, W. Lan and X. Wang, Embedded Ag quantum dots into interconnected Co3O4 nanosheets grown on 3D graphene networks for high stable and flexible supercapacitors, Electrochim. Acta, 2017, 224, 260–268 CrossRef CAS.
- Q. Qu, Y. Zhu, X. Gao and Y. Wu, Core–shell structure of polypyrrole grown on V2O5 nanoribbon as high performance anode material for supercapacitors, Adv. Energy Mater., 2012, 2, 950–955 CrossRef CAS.
- D. J. Yan, X. D. Zhu, K. X. Wang, X. T. Gao, Y. J. Feng, K. N. Sun and Y. T. Liu, Facile and elegant self-organization of Ag nanoparticles and TiO2 nanorods on V2O5 nanosheets as a superior cathode material for lithium-ion batteries, J. Mater. Chem. A, 2016, 4, 4900–4907 CAS.
- M. Lee, B. H. Wee and J. D. Hong, High Performance Flexible Supercapacitor Electrodes Composed of Ultralarge Graphene Sheets and Vanadium Dioxide, Adv. Energy Mater., 2015, 5, 1401890 CrossRef.
- X. Xia, D. Chao, C. F. Ng, J. Lin, Z. Fan, H. Zhang, Z. Shen and H. Fan, VO2 nanoflake arrays for supercapacitor and Li-ion battery electrodes: performance enhancement by hydrogen molybdenum bronze as an efficient shell material, Mater. Horiz., 2015, 2, 237–244 RSC.
- X. T. Gao, X. D. Zhu, S. R. Le, D. J. Yan, C. Y. Qu, Y. J. Feng and Y. T. Liu, Boosting High-Rate Lithium Storage of V2O5 Nanowires by Self-Assembly on N-Doped Graphene Nanosheets, ChemElectroChem, 2016, 3, 1730–1736 CrossRef CAS.
- Y. L. Ding, Y. Wen, C. Wu, P. A. van Aken, J. Maier and Y. Yu, 3D V6O13 nanotextiles assembled from interconnected nanogrooves as cathode materials for high-energy lithium ion batteries, Nano Lett., 2015, 15, 1388–1394 CrossRef CAS PubMed.
- T. Qian, N. Xu, J. Zhou, T. Yang, X. Liu, X. Shen, J. Liang and C. Yan, Interconnected three-dimensional V2O5/polypyrrole network nanostructures for high performance solid-state supercapacitors, J. Mater. Chem. A, 2015, 3, 488–493 CAS.
- A. Geim, Graphene: status and prospects, Science, 2009, 324, 1530–1534 CrossRef CAS PubMed.
- W. Deng, W. Lan, Y. Sun, Q. Su and E. Xie, Porous CoO nanostructures grown on three-dimension graphene foams for supercapacitors electrodes, Appl. Surf. Sci., 2014, 305, 433–438 CrossRef CAS.
- W. Lan, Y. Sun, Y. Chen, J. Wang, G. Tang, W. Dou and E. Xie, Ultralight and flexible supercapacitor electrodes made from Ni(OH)2 nanosheets doped with Ag nanoparticle/3D graphene composite, RSC Adv., 2015, 5, 20878–20883 RSC.
- G. Nie, X. Lu, Y. Zhu, M. Chi, M. Gao, S. Chen, M. Chi and C. Wang, Reactive Template Synthesis of Inorganic/Organic VO2@Polyaniline Coaxial Nanobelts for High-Performance Supercapacitors, ChemElectroChem, 2017, 4, 1095–1100 CrossRef CAS.
- S. J. Chae, F. Guenes, K. K. Kim, E. S. Kim and G. H. Han, Synthesis of large-area graphene layers on poly-nickel substrate by chemical vapor deposition: wrinkle formation, Adv. Mater., 2009, 21, 2328–2333 CrossRef CAS.
- A. C. Ferrari, J. C. Meyer, V. Scardaci, C. Casiraghi, M. Lazzeri and F. Mauri, Raman spectrum of graphene and graphene layers, Phys. Rev. Lett., 2006, 97, 187401 CrossRef CAS PubMed.
- C. Zhu, S. Guo, Y. Fang and S. Dong, Reducing sugar: new functional molecules for the green synthesis of graphene nanosheets, ACS Nano, 2010, 4, 2429–2437 CrossRef CAS PubMed.
- S. Stankovich, D. Dikin, R. Piner, K. Kohlhaas, A. Kleinhammes, Y. Jia, Y. Wu, S. Nguyen and R. Ruoff, Synthesis of graphene-based nanosheets via chemical reduction of exfoliated graphite oxide, Carbon, 2007, 45, 1558–1565 CrossRef CAS.
- M. Sathiya, A. Prakash, K. Ramesha, J.-M. Tarascon and A. Shukla, V2O-anchored carbon nanotubes for enhanced electrochemical energy storage, J. Am. Chem. Soc., 2011, 133, 16291–16299 CrossRef CAS PubMed.
- C. Hu, H. Xu, X. Liu, F. Zou, L. Qie, Y. Huang and X. Hu, VO2/TiO2 nanosponges as binder-free electrodes for high-performance supercapacitors, Sci. Rep., 2015, 5, 16012 CrossRef PubMed.
- T. Zhai, X. Lu, Y. Ling, M. Yu, G. Wang, T. Liu and Y. Li, A New Benchmark Capacitance for Supercapacitor Anodes by Mixed-Valence Sulfur-Doped V6O13−x, Adv. Mater., 2014, 26, 5869–5875 CrossRef CAS PubMed.
- N. Alov, D. Kutsko, I. Spirovová and Z. Bastl, XPS study of vanadium surface oxidation by oxygen ion bombardment, Surf. Sci., 2006, 600, 1628–1631 CrossRef CAS.
- X. J. Ma, W. B. Zhang, L. B. Kong, Y. C. Luo and L. Kang, VO2: from negative electrode material to symmetric electrochemical capacitor, RSC Adv., 2015, 5, 97239–97247 RSC.
- J. Zheng, Y. Zhang, X. Jing, X. Liu, T. Hu, T. Lv, S. Zhang and C. Meng, Synthesis of amorphous carbon coated on V2O3 core–shell composites for enhancing the electrochemical properties of V2O3 as supercapacitor electrode, Colloids Surf., A, 2017, 518, 188–196 CrossRef CAS.
- M. Li, G. Sun, P. Yin, C. Ruan and K. Ai, Controlling the formation of rodlike V2O5 nanocrystals on reduced graphene oxide for high-performance supercapacitors, ACS Appl. Mater. Interfaces, 2013, 5, 11462–11470 CAS.
- H. Pang, Y. Dong, S. L. Ting, J. Lu, C. Li, D. H. Kima and P. Chen, 2D single- or double-layered vanadium oxide nanosheet assembled 3D microflowers: controlled synthesis, growth mechanism, and applications, Nanoscale, 2013, 5, 7790–7794 RSC.
- L. Deng, G. Zhang, L. Kang, Z. Lei, C. Liu and Z. H. Liu, Graphene/VO2 hybrid material for high performance electrochemical capacitor, Electrochim. Acta, 2013, 112, 448–457 CrossRef CAS.
- M. Fu, C. Ge, Z. Hou, J. Cao, B. He, F. Zeng and Y. Kuang, Graphene/vanadium oxide nanotubes composite as electrode material for electrochemical capacitors, Phys. B, 2013, 421, 77–82 CrossRef CAS.
- J. Zhu, L. Cao, Y. Wu, Y. Gong, Z. Liu, H. E. Hoster, Y. Zhang, S. Zhang, S. Yang, Q. Yan, P. M. Ajayan and R. Vajtai, Building 3D structures of vanadium pentoxide nanosheets and application as electrodes in supercapacitors, Nano Lett., 2013, 13, 5408–5413 CrossRef CAS PubMed.
- J. Liu, J. Jiang, M. Bosman and H. Fan, Three-dimensional tubular arrays of MnO2–NiO nanoflakes with high areal pseudocapacitance, J. Mater. Chem., 2012, 22, 2419–2426 RSC.
- J. Shi, X. Li, G. He, L. Zhang and M. Li, Electrodeposition of high-capacitance 3D CoS/graphene nanosheets on nickel foam for high-performance aqueous asymmetric supercapacitors, J. Mater. Chem. A, 2015, 3, 20619–20626 CAS.
- M. D. Stoller and R. S. Ruoff, Best Practice Methods for Determining an Electrode Material's Performance for Ultracapacitors, Energy Environ. Sci., 2010, 3, 1294–1301 CAS.
- L. Zhang and G. Shi, Preparation of Highly Conductive Graphene Hydrogels for Fabricating Supercapacitors with High Rate Capability, J. Phys. Chem. C, 2011, 115, 17206–17212 CAS.
Footnote |
† Electronic supplementary information (ESI) available. See DOI: 10.1039/c7ra04376g |
|
This journal is © The Royal Society of Chemistry 2017 |
Click here to see how this site uses Cookies. View our privacy policy here.