DOI:
10.1039/C7RA03981F
(Paper)
RSC Adv., 2017,
7, 33194-33200
Silibinin protects Staphylococcus aureus from UVC-induced bactericide via enhanced generation of reactive oxygen species
Received
7th April 2017
, Accepted 27th June 2017
First published on 30th June 2017
Abstract
Silibinin is a major bioactive component of silymarin extracted from the milk thistle Silybum marianum. Silibinin has therapeutic potential for a wide variety of applications including anticancer, hepatoprotective and antiinflammatory medicines. There are studies reporting that silibinin has shown anti-bacterial effects, but its underlying mechanism has not yet been elucidated. In the present study, UVC inhibited growth of S. aurues in a dose-dependent manner and up-regulated production of reactive oxygen species (ROS). Silibinin treatment improved the survival of S. aureus in the presence of UVC. Interestingly, silibinin further enhanced the generation of ROS and activities of antioxidant enzymes (catalase (CAT) and glutathione peroxidase (GSH-PX)). To determine the role of ROS induced by silibinin, the scavengers (N-acetylcysteine (NAC), glutathione (GSH) and superoxide dismutase (SOD)) or donors (tBHP and H2O2) of ROS were used to treat the bacterial cells. The results showed that ROS scavengers down-regulated the protective effect of silibinin, while ROS donors up-regulated it. Therefore, ROS produced by silibinin protects S. aureus cells from UVC-induced cell death. Our findings revealed novel insights into the relationship between silibinin, bacteria and ROS. Elucidation of the relationship will contribute to the development of important applications for further use of natural products, particularly for therapeutic strategies for S. aureus-associated diseases.
Introduction
Silibinin is a popular dietary supplement and has a long history of hepatoprotective use by humans, exhibiting extremely low toxicity.1,2 In previous studies, silibinin was shown to have antibacterial effects against Bacillus subtilis and Staphylococcus epidermidis through inhibiting synthesis of macromolecules such as RNA and proteins.3 Silibinin and antibiotics (ampicillin and oxacillin) had a synergistic bactericidal effect on methicillin-resistant Staphylococcus aureus isolated from clinical specimens.4
UV irradiation induces DNA damage, such as formation of cyclobutane pyrimidine dimers (CPD).5,6 UV suppresses the transcription of target genes and induces apoptosis if unrepaired in hamster cells.7 UV is divided into three regions according to spectrum wavelength: UVA (320–400 nm), UVB (280–320 nm) and UVC (200–280 nm). Among them, UVC has the strongest bactericidal effect.
ROS are toxic byproducts of aerobic metabolism. Other stresses such as nutrient limitation and ultraviolet radiation also elicit oxidant responses.8,9 Depending on molecular sensing and efficient signaling to enhance antioxidant defense mechanisms such as eliminating/decomposing ROS or repairing the ROS-caused DNA damage, cell survival and resistance to oxidative stress are enhanced.10–12 ROS can interact with RNA, DNA, proteins and lipids.13 The cell's repairing machinery such as superoxide dismutases and catalases can regulate endogenously generated ROS and maintain them in normal level.12 On other hand, at higher concentrations, ROS could induce oxidative stress that changes the pattern of gene expression, generating more protein indirectly or directly involved in ROS scavenging or in repairing ROS-caused DNA damage.12,14
In recent years, it has been proposed that ROS are highly correlated with the cause of bacterial death.13,15 Antibiotics (norfloxacin, ampicillin and kanamycin) induce ROS by activating the electron transport chain and kill Escherichia coli (E. coli) by causing destabilization of cell structures.16 Conversely, recent report declared that ROS enhanced cephalosporin resistance in Enterococcus faecalis.17 Briefly, ROS have ambivalent effects on bacteria, leading to death or survival, implying that we need further clarification of the mechanisms that ROS affect cell life.
Many natural products and chemicals with a modulating effect on ultraviolet (UV)-induced ROS generation have been well reviewed.18 However, there are few reports on the relationship among silibinin, bacteria and ROS. Thus, in this study we investigated bioactive effects of silibinin on UVC-treated S. aureus.
In contrast to the effect of silibinin on antibiotics-induced cell death, here we found that silibinin at an optimal concentration showed a cytoprotective effect from UVC-induced cell death. ROS played an important role in this protective effect of silibinin.
Materials and methods
Bacterial strains and culture conditions
Staphylococcus aureus JCM2413T were cultured overnight on a rotary shaker (220 × g) in LB (Luria–Bertani)-rich medium (1% tryptone, 0.5% yeast extract and 1% NaCl) at 37 °C. The cells were stored at 4 °C in LB agar.
Reagents
Silibinin (99% purity) was obtained from Jurong Best Medicine Material (Zhenjiang, Jiangsu, China). It was dissolved in dimethylsulfoxide (DMSO) to make a stock solution. N-acetylcysteine (NAC), glutathione (GSH), superoxide dismutase (SOD), hydrogen peroxide (H2O2), tert-butylhydroperoxide (tBHP) and 2′,7′-dichlorofluorescein diacetate (DCFDA) were purchased from Sigma Aldrich (St Louis, MO, USA). The kits of CAT and GSH-PX were from Nanjing Jiancheng Bioengineering Institute (Nanjing, Jiangsu, China).
UVC exposure and silibinin treatment
The bacteria grown to the stationary phase (24 h) were harvested by centrifugation at 14
000 × g (10 min), followed by resuspension in fresh LB medium. Cell suspensions 0.5 mL volume were placed in sterile glass Petri dish 45 mm diameter (ensuring a bacterial monolayer) and exposed to different doses of UVC (135–1080 J m−2) with gentle shacking at room temperature. Then, the cells were cultured at 37 °C for 12 h. The radiation dose was adjusted by a UVC spectra radiometer (Lin technology Company, Shenzhen, China), with emission of UVC radiation ranging from 230 to 280 nm with a peak at 254 nm. The cells were irradiated with UVC in the presence or absence of silibinin.
Colony forming unit (CFU) assay
The survival ratios of S. aureus were determined by bacterial colony forming units (CFU). Cells subjected to the indicated treatments were collected, diluted in LB to a density of 109, and 200 μL aliquots were spread-plated (at least in triplicates) in LB medium solidified with 1.5% agar. Colonies were counted after incubation at 37 °C overnight.
Flow cytometry of DCF-DA positive cells
Intracellular production of ROS was detected using the probe 2′,7′-dichlorofluorescein diacetate (DCFDA).19 Cells subjected to the indicated treatments were collected, washed with 10 mM potassium phosphate buffer (pH 7.0), adjusted with the probe (final concentration 10 μM), and incubated at 37 °C for 60 min in dark. DCFDA-stained cells were analyzed by using a FACScan flow cytometer (Becton Dickinson, Franklin Lakes, NJ, USA).
Enzyme activity assay
Catalase (CAT) and glutathione peroxidase (GSH-PX) activities were determined with commercial kits according to the manufacturer's instructions. CAT activity was measured spectrophotometrically by monitoring the ratio of decomposition of H2O2.20 One unit of CAT was defined as the amount required to decompose 1 μmol of H2O2 per minute under the assay conditions. The GSH-PX activity was based on the principle that oxidation of glutathione (GSH) was catalyzed by GSH-PX to produce oxidized glutathione (GSSG). Decrease in the concentration of GSH that reacts with 5,5′-dithiobis(2-nitrobenzoic acid) (DTNB) yielding stable yellow substances with absorbance at 412 nm is indicative of GSH-PX activity in cells.21
Statistical analysis
All the experiments were conducted at least in triplicates (n ≥ 3). Data were expressed as the mean ± standard deviation (SD). One-way repeated analysis of variance (ANOVA) and Tukey–Kramer multiple-comparison test were used to the determine a statistical significance. GraphPad 7.0 was used for all statistical calculations. A p value of <0.05 was considered statistically significant.
Results and discussion
UVC irradiation reduced cell viability of S. aureus dose-dependently
After exposure to UVC irradiation, the numbers of S. aureus colonies were substantially reduced in a dose-dependent manner (Fig. 1A). CFU analysis also demonstrated statistically significant decreases in the bacterial loads of S. aureus after the irradiation (Fig. 1B). These results showed the bactericidal effects of UVC exposure on S. aureus. The biological effects of UV are usually attributed to enhanced production of ROS, which results in oxidative damage to lipids,22 proteins23 and DNA.24 Therefore, we moved to determine the production of ROS by monitoring the fluorescence intensity after DCFH-DA staining. Results showed that UVC increased ROS production to the maximum at 270 J m−2 (Fig. 1C).
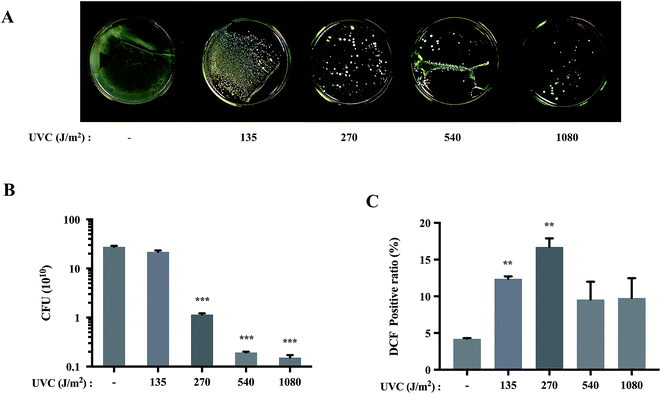 |
| Fig. 1 UVC induced the dose-dependent reduction in the viability of S. aureus cells. (A) Representative images of S. aureus following treatment with different doses of UVC. (B) CFU of S. aureus treated with different doses of UVC irradiation. (C) S. aureus cells were irradiated with different doses of UVC, re-incubated and analyzed for ROS production after 12 h. (**p < 0.01 vs. control group). Date are means value ± SD from three independent experiments. (***p < 0.001 vs. control group). | |
Silibinin protected S. aureus cells from UVC-induced cell death
Silymarin flavonolignan is a complex mixture of polyphenolic molecules in which silibinin is the major bioactive component.25,26 Silibinin and silymarin both showed antibacterial activities.27 Silymarin demonstrated antimicrobial activity against Candida albicans with a minimum inhibitory concentration (MIC) of 512 μg mL−1. Meanwhile, silibinin showed potent bactericidal activity against E. coli with a MIC of 133 μM.27 Silibinin inhibited RNA and protein synthesis in Bacillus subtilis and Staphylococcus epidermidis at the concentration of 417 μM.3
Interestingly, the present investigation provides a new role of silibinin: silibinin blocks UVC-induced S. aureus cell death. Silibinin treatment alone on S. aureus did not affect cell viability (Fig. 2A and B). However, UVC-induced S. aureus cell death was attenuated to varying degrees by silibinin administration. Silibinin exhibited the maximal effect on cell survival at 25 μM. At higher concentration (200 μM), silibinin did not reverse UVC-induced bactericidal effect (Fig. 2D and E). Silibinin plays a protective role at 25–50 μM, while it exerts bactericidal effect at concentrations higher than 100 μM.3,27 Silibinin possibly possesses bi-directional impacts on both eukaryotic and prokaryotic cells, depending on the concentrations. In human hepatocellular carcinoma cell line HepG2 cells, at a concentration of 200 μM, silibinin induces DNA lesions, generates oxidized DNA bases and reduces cell viability. However, between 10 and 100 μM, silibinin was able to reduce the genotoxic effect induced by bleomycin, benzopyrene or aflatoxinB1.28
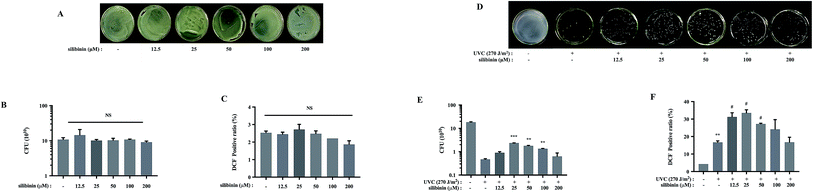 |
| Fig. 2 Silibinin was effective in protecting S. aureus cells forming UVC-induced cell death. (A) Images of S. aureus cultured following silibinin treatment at the indicated concentrations for 12 h. (B) Results of CFU in silibinin-treated S. aureus. (C) Effects of silibinin on ROS generation. (NS: nonsignificant). (D) Representative images of silibinin-treated S. aureus cultured following UVC irradiation. (E) CFU were measured after UVC irradiation in the absence or presence of silibinin. Date are means value ± SD from three independent experiments. (**p < 0.01, ***p < 0.001 vs. UVC-irradiated group). (F) UVC-irradiated S. aureus cells were post-treated with silibinin, harvested, and analyzed for ROS production. (**p < 0.01 vs. control group; #p < 0.05 vs. UVC-irradiated group). | |
It has been well documented that many polyphenols including silibinin are known to have antioxidant activity, but they can also be turned into oxidants under certain conditions.29 For example, silibinin enhances ionizing radiation-induced ROS production under prolonged oxidative stress in human prostate cancer cells.30–32 We examined the effect of silibinin on the ROS production of S. aureus, in the absence or presence of UVC. Silibinin did not increase ROS levels in S. aureus cells by itself (Fig. 2C), but it significantly enhanced ROS generation in the presence of UVC (Fig. 2F). The ROS production was dependent on silibinin dosage, increasing initially and decreasing later with a maximal enhancement with silibinin at a concentration of 25 μM. This trend was consistent with the protective efficacy of silibinin on UVC-treated S. aureus cells, suggesting a possible relationship between ROS production and cell protection with silibinin treatment.
Scavenging of ROS by NAC, GSH or SOD reduced the protective effect of silibinin on UVC-irradiated S. aureus
Programmed cell death is well established in eukaryotes. It is suggested to exist in bacteria.33–35 UVC irradiation, a kind of non-ionizing one, can induce oxidative stress, causing eukaryotic apoptosis.36 Some results suggested that UVC-induced bacterial cell death was primarily due to ROS-mediated DNA damage.37–39 Classically ROS were considered as deleterious agents, contributing to a vast range of damage in eukaryotes or prokaryotes. Consistently, in this study, high concentrations of ROS were deleterious to living cells, leading to cell death. Our results are consistent with the previous research that E. coli growth inhibition was caused by a production of ROS by silver nanoparticles.40 However, many studies implicate that ROS could function in the process of signal-transduction pathways to regulate transformation and uncontrolled growth potential of tumor cells.41,42 According to the work by Lubart and co-workers, low amounts of ROS generation could promote bacterial proliferation.43
In order to test whether ROS production was involved in the protective effect of silibinin on UVC-induced bacterial cell death, the effects of ROS scavengers were examined. It is known that NAC and GSH reduce endogenous ROS and counteract oxidative stress.44,45 GSH contains a thiol group (–SH) which is highly reactive and is the only one substrate of selenium-dependent GSH peroxidase (GSH-PX),46 and NAC is a precursor of GSH.47 SOD, an antioxidant enzyme, scavenges the excessively generated ROS, superoxide anions in particular, protecting Beauveria bassiana from damage.48 Viability of S. aureus cells was not affected by the treatment with NAC, GSH or SOD alone, as shown by the CFU assay. However, NAC, GSH and SOD down-regulated the protective effect of silibinin in UVC-irradiated S. aureus (Fig. 3A, B, E, F, I and J), suggesting a protective role of the ROS induced by silibinin treatment. NAC, GSH and SOD repressed the activities of catalase (CAT) and GSH-PX elevated by silibinin treatment, indicating a decreased oxidative stress (Fig. 3C, D, G, H, K and L). Therefore, we speculated that some kinds of ROS at a localized region in cells or for a time exerted the protective roles in UVC-treated S. aureus cell death.
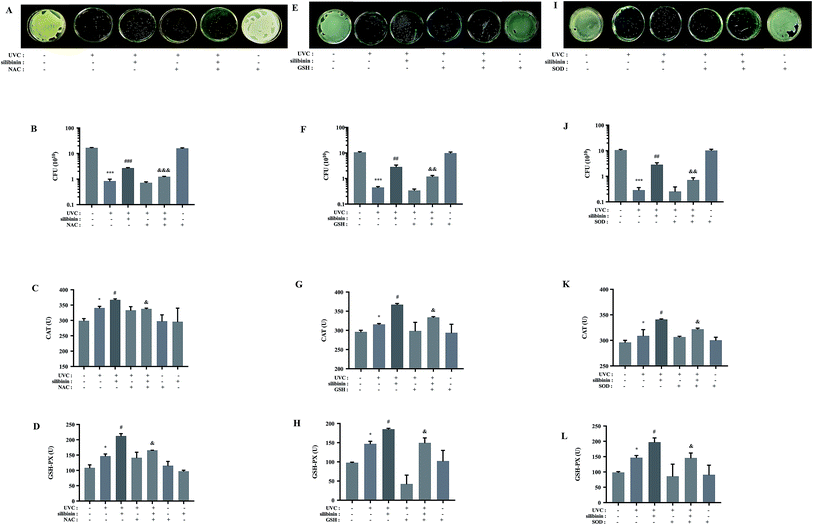 |
| Fig. 3 Scavenging of ROS by NAC, GSH or SOD reduced the protective effect of silibinin on UVC-irradiated S. aureus. In the absence or presence of NAC (8 mM), GSH (10 mM) and SOD (75 U), UVC-irradiated S. aureus were treated with silibinin. Images of representative cultures of S. aureus treated with NAC, GSH or SOD. CFU were determined (A and B, E and F, I and J). (***p < 0.001 vs. control group; ##p < 0.01, ###p < 0.001 vs. UVC-irradiated group; &p < 0.05, &&p < 0.01, &&&p < 0.001 vs. silibinin plus UVC-irradiated group). Changes of the activity of CAT (C, G and K) and GSH-PX (D, H and L) in S. aureus. (*p < 0.05 vs. control group; #p < 0.05 vs. UVC-irradiated group; &p < 0.05 vs. silibinin plus UVC-irradiated group). Date are means ± SD from three independent experiments. | |
Silibinin might enhance ROS level, protecting S. aureus from UVC irradiation
tBHP is an organic hydroperoxide which has been usually employed to induce oxidative stress in various biological systems.49 H2O2 is one type of ROS. High concentrations of ROS can cause the death of microorganisms.50 As Fig. 4A–D and G–J shows that in the absence or presence of UVC irradiation, both 80 μM tBHP and 8 mM H2O2 significantly reduced S. aureus growth ratio. But when the UVC-irradiated bacteria were treated with lower concentrations of tBHP (10 and 40 μM) or H2O2 (ranged from 0.5 to 4 mM), the growth ratio did not significantly change (Fig. 4D and J). Moreover, the growth ratio in 20 μM tBHP-treated group (4.4 ± 0.4060) was significantly higher than that in silibinin alone group (2.66 ± 0.1155) in UVC-treated S. aureus (Fig. 4E and F). Treatment with 1 mM H2O2 also increased the growth ratio to about 1.5 folds (4.17 ± 0.5657) of that treated with only silibinin (2.87 ± 0.5033) in UVC-irradiated S. aureus (Fig. 4K and L). These results suggested that silibinin and tBHP/H2O2 have a synergistic protective effect. High levels of ROS induced oxidative stress, leading to bacterial cell death. However, the certain concentration of ROS may play a potentially protective role in S. aureus.
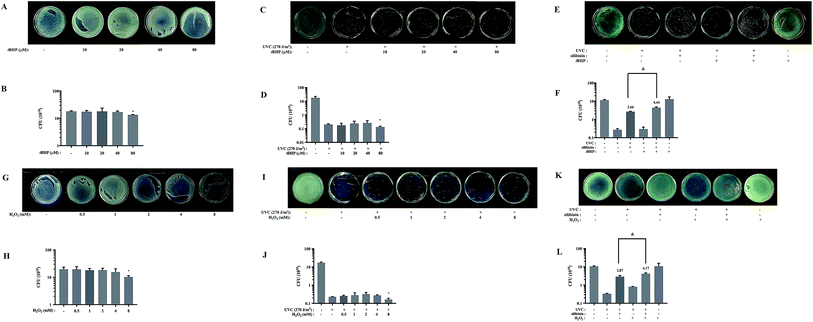 |
| Fig. 4 Silibinin might enhance ROS level, protecting S. aureus from UVC irradiation. Images of cultures as follow S. aureus were treated with tBHP from 10 to 80 μM or H2O2 from 0.5 to 8 mM were shown (A and G) and CFU were determined (B and H). (*p < 0.05 vs. control group). (C and I) Representative images of tBHP- or H2O2-treated S. aureus cultured, following UVC irradiation. (D and J) CFU were measured after UVC irradiation with or without of tBHP or H2O2. (*p < 0.05 vs. UVC-irradiated group). In the absence or presence of H2O2 or tBHP, UVC-irradiated S. aureus were treated with silibinin. Representative images of cultured S. aureus with different treatments. CFU assays were conducted (E, F, K and L). (&p < 0.05 vs. silibinin plus UVC-irradiated group). Date are means ± SD from three independent experiments. | |
UVC irradiation induces the formation of various reactive oxygen species.8,51 Low concentration of H2O2 could play a crucial role in regulation of cell metabolism and cellular signaling in response to environmental stresses such as low- or medium-pressure UV irradiation.52 Our results demonstrated that H2O2 enhanced the protective effect of silibinin on UVC-induced S. aureus cell death. We speculated that silibinin protects UVC-irradiated bacteria from cell death via enhancing production of some kinds of ROS. But the precise identification of these molecules and their mechanisms requires further investigation.
Mitochondria are evolved from endosymbiotic α-proteobacteria belonging to Rickettsia gender.53,54 They still present many similarities to prokaryotic cells such as a double membrane, and the ability to produce ATP through the generation of a proton gradient generated across the inner membrane.53,54 As toxic byproducts of aerobic metabolism, ROS are primarily formed in mitochondria and peroxisomes, but also at any other cellular compartments. They are then removed or detoxified by an array of antioxidative enzymes such as CAT and SOD, and antioxidants. Many antioxidative systems of the cells, therefore, keep ROS at a basal non-toxic level.55 In eukaryotes, mitochondrial oxidative stress leads to cell death, as 5-aminolevulinic acid enhances ionizing irradiation-induced mitochondrial oxidative stress and leads to increased glioma cell death accompanying changes of mitochondrial morphology.56 However, mitochondrial oxidative stress also activates cell survival signaling.57 Silibinin induces excess mitochondrial ROS production, resulting in increased survival of human melanoma A375-S2 cells.58 The effect of ROS in bacteria and mitochondria might potentially share some similar regulation mechanisms through ROS.
Conclusions
Oxidative stress has been implicated as one of the mechanisms whereby UVC kills bacteria. The formation of ROS may play distinct roles in bacteria killing and survival. The present study provides new facts that silibinin-induced ROS are beneficial to the survival of S. aureus under UVC-irradiated conditions. The study uncovers novel insights into the relationships between silibinin and bacteria, which has important implications for the usage of natural products in development of therapeutic strategies for S. aureus.
Acknowledgements
This research was supported by National Natural Science Foundation of China (No. 81273517).
References
- E. S. Hackett, D. C. Twedt and D. L. Gustafson, Milk thistle and its derivative compounds: a review of opportunities for treatment of liver disease, J. Vet. Intern. Med., 2013, 27, 10–16 CrossRef CAS PubMed.
- I. Anestopoulos, A. Kavo, I. Tentes, A. Kortsaris, M. Panayiotidis, A. Lazou and A. Pappa, Silibinin protects H9c2 cardiac cells from oxidative stress and inhibits phenylephrine-induced hypertrophy: potential mechanisms, J. Nutr. Biochem., 2013, 24, 586–594 CrossRef CAS PubMed.
- D. G. Lee, H. K. Kim, Y. Park, S. C. Park, E. R. Woo, H. G. Jeong and K. S. Hahm, Gram-positive bacteria specific properties of silybin derived from Silybum marianum, Arch. Pharmacal Res., 2003, 26, 597–600 CrossRef CAS.
- H. K. Kang, H. Y. Kim and J. D. Cha, Synergistic effects between silibinin and antibiotics on methicillin-resistant Staphylococcus aureus isolated from clinical specimens, Biotechnol. J., 2011, 6, 1397–1408 CrossRef CAS PubMed.
- J. L. Ravanat, T. Douki and J. Cadet, Direct and indirect effects of UV radiation on DNA and its components, J. Photochem. Photobiol., B, 2001, 63, 88–102 CrossRef CAS.
- R. P. Gallagher and T. K. Lee, Adverse effects of ultraviolet radiation: a brief review, Prog. Biophys. Mol. Biol., 2006, 92, 119–131 CrossRef CAS PubMed.
- L. Proietti De Santis, C. L. Garcia, A. S. Balajee, P. Latini, P. Pichierri, O. Nikaido, M. Stefanini and F. Palitti, Transcription coupled repair efficiency determines the cell cycle progression and apoptosis after UV exposure in hamster cells, DNA Repair, 2002, 1, 209–223 CrossRef PubMed.
- A. L. Santos, V. Oliveira, I. Baptista, I. Henriques, N. C. Gomes, A. Almeida, A. Correia and A. Cunha, Wavelength dependence of biological damage induced by UV radiation on bacteria, Arch. Microbiol., 2013, 195, 63–74 CrossRef CAS PubMed.
- M. Khakimova, H. G. Ahlgren, J. J. Harrison, A. M. English and D. Nguyen, The stringent response controls catalases in Pseudomonas aeruginosa and is required for hydrogen peroxide and antibiotic tolerance, J. Bacteriol., 2013, 195, 2011–2020 CrossRef CAS PubMed.
- A. Ikner and K. Shiozaki, Yeast signaling pathways in the oxidative stress response, Mutat. Res., 2005, 569, 13–27 CrossRef CAS PubMed.
- R. X. Santos, S. C. Melo, J. C. Cascardo, M. Brendel and C. Pungartnik, Carbon source-dependent variation of acquired mutagen resistance of Moniliophthora perniciosa: similarities in natural and artificial systems, Fungal Genet. Biol., 2008, 45, 851–860 CrossRef CAS PubMed.
- A. Boveris, Biochemistry of free radicals: from electrons to tissues, Medicina, 1998, 58, 350–356 CAS.
- B. Ezraty, A. Gennaris, F. Barras and J. F. Collet, Oxidative stress, protein damage and repair in bacteria, Nat. Rev. Microbiol., 2017, 15(7), 385–396 CrossRef CAS PubMed.
- D. Slade and M. Radman, Oxidative stress resistance in Deinococcus radiodurans, Microbiol. Mol. Biol. Rev., 2011, 75, 133–191 CrossRef CAS PubMed.
- D. J. Dwyer, M. A. Kohanski and J. J. Collins, Role of reactive oxygen species in antibiotic action and resistance, Curr. Opin. Microbiol., 2009, 12, 482–489 CrossRef CAS PubMed.
- M. A. Kohanski, D. J. Dwyer, B. Hayete, C. A. Lawrence and J. J. Collins, A common mechanism of cellular death induced by bactericidal antibiotics, Cell, 2007, 130, 797–810 CrossRef CAS PubMed.
- D. Djoric and C. J. Kristich, Oxidative stress enhances cephalosporin resistance of Enterococcus faecalis through activation of a two-component signaling system, Antimicrob. Agents Chemother., 2015, 59, 159–169 CrossRef PubMed.
- A. A. Farooqi, R. N. Li, H. W. Huang, M. Ismail, S. S. Yuan, H. M. Wang, J. R. Liu, J. Y. Tang and H. W. Chang, Natural Products Mediated Regulation of Oxidative Stress and DNA Damage in Ultraviolet Exposed Skin Cells, Curr. Pharm. Biotechnol., 2015, 16, 1078–1084 CAS.
- J. M. Perez, I. L. Calderon, F. A. Arenas, D. E. Fuentes, G. A. Pradenas, E. L. Fuentes, J. M. Sandoval, M. E. Castro, A. O. Elias and C. C. Vasquez, Bacterial toxicity of potassium tellurite: unveiling an ancient enigma, PLoS One, 2007, 2, e211 Search PubMed.
- R. F. Beers Jr and I. W. Sizer, A spectrophotometric method for measuring the breakdown of hydrogen peroxide by catalase, J. Biol. Chem., 1952, 195, 133–140 CAS.
- K. Ngamchuea, C. Batchelor-McAuley and R. G. Compton, The Copper(II)-Catalyzed Oxidation of Glutathione, Chemistry, 2016, 22, 15937–15944 CrossRef CAS PubMed.
- J. Chamberlain and S. H. Moss, Lipid peroxidation and other membrane damage produced in Escherichia coli K1060 by near-UV radiation and deuterium oxide, Photochem. Photobiol., 1987, 45, 625–630 CrossRef CAS PubMed.
- R. A. Pizarro and L. V. Orce, Membrane damage and recovery associated with growth delay induced by near-UV radiation in Escherichia coli K-12, Photochem. Photobiol., 1988, 47, 391–397 CrossRef CAS PubMed.
- G. P. Pfeifer, Formation and processing of UV photoproducts: effects of DNA sequence and chromatin environment, Photochem. Photobiol., 1997, 65, 270–283 CrossRef CAS PubMed.
- V. Kren and D. Walterova, Silybin and silymarin – new effects and applications, Biomedical Papers, 2005, 149, 29–41 CrossRef CAS.
- A. Tyagi, K. Raina, R. P. Singh, M. Gu, C. Agarwal, G. Harrison, L. M. Glode and R. Agarwal, Chemopreventive effects of silymarin and silibinin on N-butyl-N-(4-hydroxybutyl) nitrosamine induced urinary bladder carcinogenesis in male ICR mice, Mol. Cancer Ther., 2007, 6, 3248–3255 CrossRef CAS PubMed.
- D. R. de Oliveira, S. R. Tintino, M. F. Braga, A. A. Boligon, M. L. Athayde, H. D. Coutinho, I. R. de Menezes and R. Fachinetto, In vitro antimicrobial and modulatory activity of the natural products silymarin and silibinin, BioMed Res. Int., 2015, 2015, 292797 Search PubMed.
- J. P. Angeli, G. R. Barcelos, J. M. Serpeloni, F. Barbosa Jr, A. Nersesyan and M. S. Mantovani, Evaluation of the genotoxic and anti-genotoxic activities of silybin in human hepatoma cells (HepG2), Mutagenesis, 2010, 25, 223–229 CrossRef CAS PubMed.
- N. Zheng, L. Liu, W. W. Liu, F. Li, T. Hayashi, S. I. Tashiro, S. Onodera and T. Ikejima, Crosstalk of ROS/RNS and autophagy in silibinin-induced apoptosis of MCF-7 human breast cancer cells in vitro, Acta Pharmacol. Sin., 2017, 38, 277–289 CrossRef CAS PubMed.
- S. Bhaumik, R. Anjum, N. Rangaraj, B. V. Pardhasaradhi and A. Khar, Curcumin mediated apoptosis in AK-5 tumor cells involves the production of reactive oxygen intermediates, FEBS Lett., 1999, 456, 311–314 CrossRef CAS PubMed.
- G. Galati, O. Sabzevari, J. X. Wilson and P. J. O'Brien, Prooxidant activity and cellular effects of the phenoxyl radicals of dietary flavonoids and other polyphenolics, Toxicology, 2002, 177, 91–104 CrossRef CAS PubMed.
- D. K. Nambiar, P. Rajamani, G. Deep, A. K. Jain, R. Agarwal and R. P. Singh, Silibinin Preferentially Radiosensitizes Prostate Cancer by Inhibiting DNA Repair Signaling, Mol. Cancer Ther., 2015, 14, 2722–2734 CrossRef CAS PubMed.
- J. C. Ameisen, The origin of programmed cell death, Science, 1996, 272, 1278–1279 CrossRef CAS PubMed.
- J. C. Ameisen, Looking for death at the core of life in the light of evolution, Cell Death Differ., 2004, 11, 4–10 CrossRef CAS PubMed.
- K. Lewis, Programmed death in bacteria, Microbiol. Mol. Biol. Rev., 2000, 64, 503–514 CrossRef CAS PubMed.
- J. P. Blaydes, A. L. Craig, M. Wallace, H. M. Ball, N. J. Traynor, N. K. Gibbs and T. R. Hupp, Synergistic activation of p53-dependent transcription by two cooperating damage recognition pathways, Oncogene, 2000, 19, 3829–3839 CrossRef CAS PubMed.
- A. A. Gomes, A. C. Silva-Junior, E. B. Oliveira, L. M. Asad, N. C. Reis, I. Felzenszwalb, K. Kovary and N. R. Asad, Reactive oxygen species mediate lethality induced by far-UV in Escherichia coli cells, Redox Rep., 2005, 10, 91–95 CrossRef CAS PubMed.
- A. Krisko and M. Radman, Protein damage and death by radiation in Escherichia coli and Deinococcus radiodurans, Proc. Natl. Acad. Sci. U. S. A., 2010, 107, 14373–14377 CrossRef CAS PubMed.
- A. C. Silva-Junior, L. M. Asad, I. Felzenszwalb and N. R. Asad, Mutagenicity induced by UVC in Escherichia coli cells: reactive oxygen species involvement, Redox Rep., 2011, 16, 187–192 CrossRef CAS PubMed.
- M. A. Quinteros, V. Cano Aristizabal, P. R. Dalmasso, M. G. Paraje and P. L. Paez, Oxidative stress generation of silver nanoparticles in three bacterial genera and its relationship with the antimicrobial activity, Toxicol. In Vitro, 2016, 36, 216–223 CrossRef CAS PubMed.
- C. Xia, Q. Meng, L. Z. Liu, Y. Rojanasakul, X. R. Wang and B. H. Jiang, Reactive oxygen species regulate angiogenesis and tumor growth through vascular endothelial growth factor, Cancer Res., 2007, 67, 10823–10830 CrossRef CAS PubMed.
- J. E. Klaunig and L. M. Kamendulis, The role of oxidative stress in carcinogenesis, Annu. Rev. Pharmacol. Toxicol., 2004, 44, 239–267 CrossRef CAS PubMed.
- R. Lubart, A. Lipovski, Y. Nitzan and H. Friedmann, A possible mechanism for the bactericidal effect of visible light, Laser Therapy, 2011, 20, 17–22 CrossRef CAS PubMed.
- N. M. Reddy, S. R. Kleeberger, J. H. Bream, P. G. Fallon, T. W. Kensler, M. Yamamoto and S. P. Reddy, Genetic disruption of the Nrf2 compromises cell-cycle progression by impairing GSH-induced redox signaling, Oncogene, 2008, 27, 5821–5832 CrossRef CAS PubMed.
- I. Pocsi, R. A. Prade and M. J. Penninckx, Glutathione, altruistic metabolite in fungi, Adv. Microb. Physiol., 2004, 49, 1–76 CrossRef CAS PubMed.
- L. Flohe, The glutathione peroxidase reaction: molecular basis of the antioxidant function of selenium in mammals, Curr. Top. Cell. Regul., 1985, 27, 473–478 CAS.
- I. Downs, J. Liu, T. Y. Aw, P. A. Adegboyega and M. N. Ajuebor, The ROS scavenger, NAC, regulates hepatic Valpha14iNKT cells signaling during Fas mAb-dependent fulminant liver failure, PLoS One, 2012, 7, e38051 CAS.
- F. Martins, J. A. Pereira and P. Baptista, Oxidative stress response of Beauveria bassiana to Bordeaux mixture and its influence on fungus growth and development, Pest Manage. Sci., 2014, 70, 1220–1227 CrossRef CAS PubMed.
- T. R. Kumar and Muralidhara, Induction of oxidative stress by organic hydroperoxides in testis and epididymal sperm of rats in vivo, J. Androl., 2007, 28, 77–85 CrossRef CAS PubMed.
- P. L. Paez, M. C. Becerra and I. Albesa, Comparison of macromolecular oxidation by reactive oxygen species in three bacterial genera exposed to different antibiotics, Cell Biochem. Biophys., 2011, 61, 467–472 CrossRef CAS PubMed.
- Z. B. Alam, M. Otaki, H. Furumai and S. Ohgaki, Direct and indirect inactivation of Microcystis aeruginosa by UV-radiation, Water Res., 2001, 35, 1008–1014 CrossRef CAS PubMed.
- I. Slesak, M. Libik, B. Karpinska, S. Karpinski and Z. Miszalski, The role of hydrogen peroxide in regulation of plant metabolism and cellular signalling in response to environmental stresses, Acta Biochim. Pol., 2007, 54, 39–50 CAS.
- M. J. Pallen, Time to recognise that mitochondria are bacteria?, Trends Microbiol., 2011, 19, 58–64 CrossRef CAS PubMed.
- E. Lobet, J. J. Letesson and T. Arnould, Mitochondria: a target for bacteria, Biochem. Pharmacol., 2015, 94, 173–185 CrossRef CAS PubMed.
- R. Mittler, S. Vanderauwera, M. Gollery and F. Van Breusegem, Reactive oxygen gene network of plants, Trends Plant Sci., 2004, 9, 490–498 CrossRef CAS PubMed.
- K. Ueta, J. Yamamoto, T. Tanaka, Y. Nakano, T. Kitagawa and S. Nishizawa, 5-Aminolevulinic acid enhances mitochondrial stress upon ionizing irradiation exposure and increases delayed production of reactive oxygen species and cell death in glioma cells, Int. J. Mol. Med., 2017, 39, 387–398 Search PubMed.
- T. Chao, H. Wang and P. C. Ho, Mitochondrial Control and Guidance of Cellular Activities of T Cells, Front. Immunol., 2017, 8, 473 CrossRef PubMed.
- Y. Y. Jiang, H. Huang, H. J. Wang, D. Wu, R. Yang, S. Tashiro, S. Onodera and T. Ikejima, Interruption of mitochondrial complex IV activity and cytochrome c expression activated O(2). (−)-Mediated cell survival in silibinin-treated human melanoma A375-S2 cells via IGF-1R-PI3K-Akt and IGF-1R-PLC gamma-PKC pathways, Eur. J. Pharmacol., 2011, 668, 78–87 CrossRef CAS PubMed.
|
This journal is © The Royal Society of Chemistry 2017 |