DOI:
10.1039/C7RA02376F
(Paper)
RSC Adv., 2017,
7, 26250-26255
Investigating resonance energy transfer from protein molecules to van der Waals nanosheets
Received
25th February 2017
, Accepted 5th May 2017
First published on 17th May 2017
Abstract
The resonance energy transfer (RET) from tryptophan present in bovine serum albumin (BSA) to two dimensional (2D) nanomaterials has been reported. In these bio-nano systems, BSA molecules act as efficient donors while 2D van der Waals nanosheets of graphene, molybdenum disulfide (MoS2) and tungsten disulfide (WS2) act as acceptors. The fluorescence emission from tryptophan arises at 350 nm, in presence of 2D nanosheets, the fluorescence intensity of BSA decreases. The time resolved fluorescence study shows that there is decrease in the fluorescence lifetime of BSA molecules in presence of 2D nanosheets. The decrease in fluorescence lifetime of BSA confirms the energy transfer phenomenon in bio-nano interactions.
1 Introduction
In recent years, two dimensional (2D) nanosheets of van der Waals (vdW) materials have been extensively studied due to their intriguing optical, electronic, structural and chemical properties.1–5 These materials include a wide range of nanomaterials – zero band gap to non-zero band gap. Among various types of vdW nanomaterials, graphene and transition metal dichalcogenides (TMDCs) nanosheets of the MX2 type (M = Mo, W; X = S, Se, Te), are the most studied materials.6–9 These materials have the advantage over other nanomaterials due to their high stability, high surface to volume ratio and a number of layer dependent electronic and optical properties.10–12 Graphene consists of carbon atoms, arranged in a regular atomic-scale chicken wire (hexagonal) pattern. The emergence of graphene helped further the exploration of other two dimensional nanomaterials like, 2D sheets of TMDCs.13,14 Among various TMDCs nanosheets, MoS2 and WS2 have been lately used in various optoelectronic devices. In bulk form, these TMDCs are found to be indirect band gap semiconductors, while for single layers, they are direct band gap semiconductors with different band gap energies, e.g., MoS2 has 1.2 eV indirect band gap in bulk form, while for single layer it has direct band gap of 1.8 eV, and similarly, WS2 has 1.4 eV indirect band gap in bulk form, while in case of single layer it is a 2.1 eV direct band gap semiconductor.15–19 Recently, graphene as well as TMDC nanosheets have been used in resonance energy transfer (RET) phenomenon as acceptors of energy/charge.20–25 The RET phenomenon is observed in various physical, chemical and biological systems.26,27 In order to observe RET, one material acts as a donor and the other material as an acceptor of energy. Very recently, Sampat et al. has studied RET from quantum dots to MoS2 nanosheets, Goodfellow et al. used CdSe/CdS QDs as donors and WS2 nanosheets as acceptor in RET.20,24 Similarly, Swathi et al. used a fluorescent dye as a donor and graphene as an acceptor for resonance energy transfer.28 There are fewer studies on RET in biomolecules like protein and nanomaterials. Sen et al. have investigated RET in human serum albumin (HSA) protein molecules and gold nanoparticles.29 Recently, Kuchlyan et al. have studied the interaction of BSA molecules with graphene oxide nanosheets.30 Prior to application of nanomaterials in biological systems, testing the biocompatibility of the nanomaterials is essential. In this regard, the interaction of two dimensional nanosheets with biomolecules needs to be explored before their use in diagnosis and biomedical applications. The present study is important in the context of application of two dimensional nanosheets in bio-related fields, as for the first time here, we have investigated the bio-nano interaction in 2D nanosheets and bovine serum albumin molecules by means of resonance energy transfer.
2 Materials and methods
2.1 Materials
Bovine serum albumin, graphite powder, molybdenum disulfide (MoS2), tungsten disulfide (WS2) were obtained from Sigma-Aldrich and used without further purification. In all the experiments, ultrapure double distilled water was used.
2.2 Synthesis of van der Waals nanosheets
In this study, the van der Waals (vdW) nanosheets were synthesized by using the liquid exfoliation method. In a typical synthesis procedure, 50 mg of vdW powder was dispersed in 10 mL of aqueous solution containing 10 mg BSA powder. Further the solution was ultrasonicated for 20 h using 25 W ultrasonication bath. After ultrasonication, the color of the solution changed, e.g., for MoS2 from black to green, which confirmed the formation of nanosheets from bulk powder. Similarly, the color of WS2 changed to brown, while that of graphene remained black. The supernatant was then collected and re-dispersed in water to prepare diluted stock solutions (0.05 mg mL−1) of vdW nanosheets. The schematic diagram of the chemical exfoliation process is shown in Fig. 1.
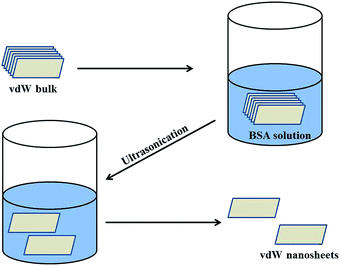 |
| Fig. 1 Schematic diagram for synthesis of van der Waals nanosheets. | |
2.3 Characterization
The solutions were drop casted on silicon substrates and dried at room temperature for Raman spectroscopic studies. These nanosheets were characterized by transmission electron microscopy (TEM), Raman spectroscopy and absorption spectroscopy. The Raman spectra of graphene, MoS2 and WS2 nanosheets were recorded by Renishaw inVia confocal Raman spectrometer using 532 nm excitation source. For TEM study, the aqueous dispersions of the nanosheets were put on carbon coated copper grids and dried at room temperature. The TEM images of these nanosheets were recorded using JEOL-2010 TEM setup, which was operated at an accelerating voltage of 200 kV. The elemental analysis of the fluorescent protein was done using energy dispersive X-ray (EDX) measurement, which was carried out on ZEISS EVO40-scanning electron microscopy (SEM) set up with energy 15 keV. The absorption spectra of these nanosheets were recorded using Shimadzu (UV-2450) UV-Vis spectrophotometer. In order to study RET in BSA and the van der Waals nanosheets, different quantities of nanosheets were mixed with a fixed quantity of BSA containing aqueous solution. All samples were prepared such that the amount of BSA molecules remains fixed (0.5 mg in 3.5 mL solution) in all sets, while only the quantities of the nanosheets were varied in different samples. All samples were incubated for 24 h to achieve equilibrium. The steady state fluorescence emissions of BSA, in absence and presence of nanosheets, were recorded using fluorimeter with excitation wavelength of 280 nm. The time resolved fluorescence study was performed using time correlated single photon counting (TCSPC) setup (FL920, Edinburgh Instruments, UK) with 284 nm light emitting diode as the excitation source.
3 Results and discussion
3.1 Raman study
The Raman spectra of the van der Waals nanosheets are shown in Fig. 2. Fig. 2a shows the Raman spectrum of graphene nanosheet. In case of graphene, three distinct Raman peaks are observed: 1350, 1582 and 2700 cm−1; these peaks are known as D, G and 2D peaks, respectively.31 D and G peaks originate due to the breathing modes of sp2 carbon atoms in the ring and stretching of carbon–carbon bonds respectively. The Raman spectrum of MoS2 nanosheet is shown in Fig. 2b. There are two distinct Raman peaks: one at 385 cm−1 and the other at 405 cm−1. These peaks arise due to in-plane and out-of-plane vibrations of S–Mo–S atoms, and are known as E2g1 and A1g vibrational modes, respectively.32 In case of WS2 nanosheets, E2g1 and A1g Raman peaks are observed at 351 cm−1 and 420 cm−1, respectively.
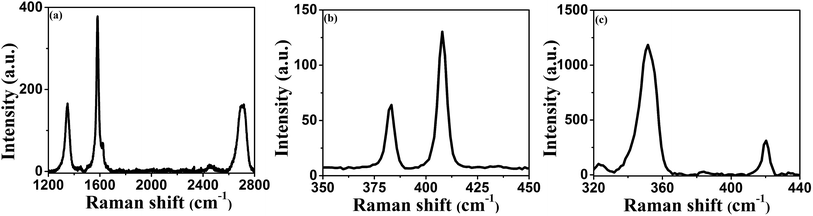 |
| Fig. 2 Raman spectra of van der Waals nanosheets of (a) graphene, (b) MoS2, and (c) WS2. | |
3.2 TEM analysis
The morphologies of two dimensional vdW nanosheets of graphene, MoS2 and WS2, were analyzed by transmission electron microscopy (TEM). Fig. 3a shows the TEM image of graphene nanosheets. The selective area electron diffraction (SAED) of graphene is shown in Fig. 3b. Similarly, the TEM images of MoS2 and WS2 are shown in Fig. 3c and e, respectively, and the Fig. 3d and f show the selective area electron diffraction (SAED) patterns of MoS2 and WS2 nanosheets, respectively.
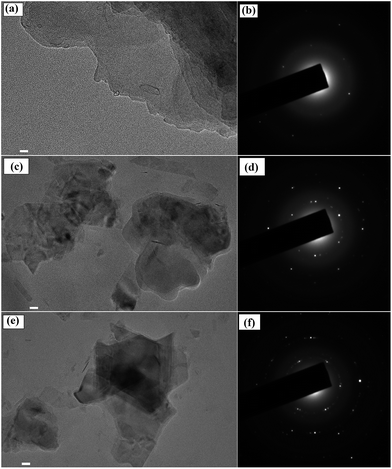 |
| Fig. 3 TEM images and SAED patterns of (a) and (b) graphene, (c) and (d) MoS2, (e) and (f) WS2 nanosheets, respectively. Scale bar equals to 20 nm. | |
3.3 EDX analysis
The elemental analysis of the fluorophore (BSA protein) molecules was done by using EDX study. The EDX spectrum of BSA is shown in Fig. 4. The spectrum consists of peaks corresponding to different elements present in BSA molecules. In EDX spectrum the peaks corresponding to carbon, nitrogen, oxygen, sodium and sulfur are observed at 0.277, 0.392, 0.525, 1.041 and 2.307 keV, respectively. Additional peak corresponding to silicon is observed at 1.739 keV, which originates due to the silicon substrate.
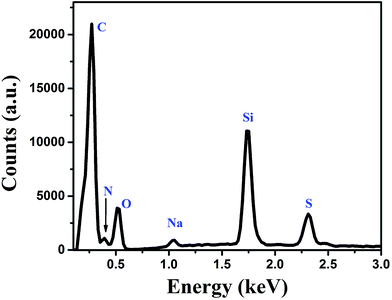 |
| Fig. 4 Energy dispersive X-ray spectrum of the fluorescent BSA molecules. | |
3.4 UV-visible absorption
The optical absorbance spectra of vdW nanosheets were recorded by the absorption spectrophotometer, and are shown in Fig. 5.
 |
| Fig. 5 Absorption spectra of graphene, MoS2 nanosheets, and WS2 nanosheets, along with the emission spectrum of BSA. Inset: schematic representation of energy transfer between BSA and the van der Waals nanosheets. | |
The absorption spectrum of graphene shows an absorption peak around 280 nm. This absorption peak arises due to π-conjugation, which is partially restored within the graphene sheets. In case of MoS2, two absorption peaks are observed; one at 620 nm and other at 670 nm. These peaks are the resultant of transitions between valence band and conduction band of MoS2. The absorption peaks observed at 620 nm and 670 nm are assigned as B and A exciton peaks, respectively. Similarly, for WS2 nanosheets the absorption peaks are observed at 530 nm and 630 nm. The absorption peaks are similar to the previous ones reported.33
3.5 Fluorescence study
The interaction of these nanosheets with BSA molecules was studied by using fluorescence spectroscopic techniques. The steady state fluorescence spectra of BSA molecules in absence and presence of 2D nanosheets are recorded by fluorimeter using 280 nm excitation wavelength, and emission was recorded in wavelength range of 290–500 nm. The steady state fluorescence spectra of BSA molecules in presence of different concentration of 2D nanosheets are shown in Fig. 6. BSA shows fluorescence emission at 350 nm, which arises due to tryptophan present in BSA molecules.34 In Fig. 6a, it is found that with increase in the concentration of graphene nanosheets, the fluorescence (Fl) intensity of BSA decreases. The decrease in Fl intensity confirms quenching due to graphene nanosheets. In Fig. 6b fluorescence quenching of BSA molecules in presence of MoS2 nanosheets is shown. Similarly, for WS2 nanosheets, the quenching of BSA fluorescence is shown in Fig. 6c. The fluorescence quenching of BSA molecules is confirmed, but in order to ascertain the quenching nature (static or dynamic), time resolved fluorescence study was done using time correlated single photon counting technique. It is observed that the fluorescence lifetime of BSA decays, in absence and presence of different concentrations of vdW nanosheets. The fluorescence lifetime decay spectra of BSA, in the absence and presence of 2D nanosheets, are shown in Fig. 7. Fig. 7a shows the Fl lifetime decay curve of BSA molecules in presence of graphene nanosheets. It is observed that with increase in the quantity of graphene, the fluorescence decay curves are steeper in comparison to pure BSA. There is a systematic change in the decay curves with increase in the quantity of graphene nanosheets. Similarly, Fig. 7b and c show the fluorescence lifetime decays of BSA, in presence of MoS2 and WS2 nanosheets, respectively. These decay curves were fitted with exponential decaying functions of following form:35 |
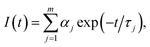 | (1) |
where m is the number of discrete decay components, τj are the decay times, and αj represents the weighing parameter associated with the jth decay. The decay curve of BSA in absence of nanosheets is fitted with bi-exponential (m = 2) decaying function, while in presence of nanosheets the curves are fitted with triple-exponential decaying function (fitted curves not shown). The average fluorescence lifetimes of BSA in absence, as well as in presence of nanosheets, were calculated using following equation:36 |
 | (2) |
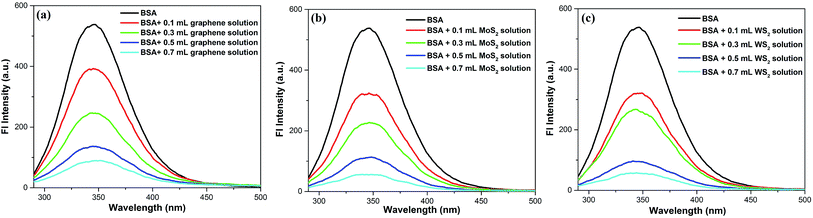 |
| Fig. 6 Fluorescence spectra of BSA in absence and presence of different quantities of (a) graphene, (b) MoS2, and (c) WS2 nanosheets, respectively. | |
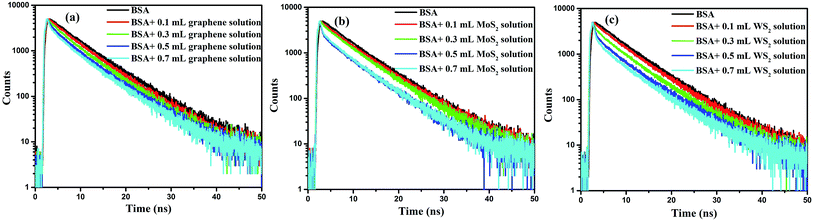 |
| Fig. 7 Fluorescence lifetime decay curves of BSA, in the absence and in the presence of different quantities of (a) graphene, (b) MoS2, and (c) WS2 nanosheets, respectively. | |
The average fluorescence lifetime of pure BSA was found to be 6.41 ns. In presence of different quantities of graphene, the Fl lifetime decreases – for 0.1, 0.3, 0.5 and 0.7 mL of graphene solutions, the Fl lifetimes are found to be 6.08, 5.89, 5.66 and 5.55 ns, respectively. Thus, there is a systematic decrease in the Fl lifetimes of BSA molecules in presence of different quantities of graphene. Similarly, for MoS2 nanosheets, the respective Fl lifetimes are found to be 6.39, 6.22, 5.70 and 5.62 ns in presence of 0.1, 0.3, 0.5 and 0.7 mL of MoS2 nanosheets. For WS2 the values are 6.26, 5.93, 5.61, 5.44 ns with 0.1, 0.3, 0.5 and 0.7 mL of WS2 solutions, respectively. In all cases, with increase in the quantity of vdW nanosheets, there is a corresponding decrease in the Fl lifetime of BSA molecules. In presence of 2D nanosheets, the fluorescence intensity as well as the fluorescence lifetime of BSA molecules are found to decrease. In Fig. 8, variation of Fl intensity and Fl lifetime of BSA with different quantities of 2D nanosheets are shown. The decrease in lifetime indicates that there is some energy transfer from the BSA molecules to 2D nanosheets. In Fig. 5, it is shown that the emission spectrum of BSA overlaps with the absorption spectra of vdW nanosheets. This results in transfer of some energy from BSA molecules to the nanosheets. In the process of energy transfer, the fluorophores show quenching in the Fl intensity, as well as Fl lifetimes. Using Fl lifetime data, the energy transfer efficiency from BSA molecules to 2D nanosheets has been calculated using following equation:
|
 | (3) |
Here,
τD and
τDA are fluorescence lifetimes of BSA, in absence and presence of 2D nanosheets (acceptors), respectively. It is found that with increase in the concentration of acceptors the energy transfer efficiency increases. The energy transfer efficiencies, in case of graphene are found to be 5, 8, 12 and 13% for 0.1, 0.3, 0.5 and 0.7 mL of graphene solutions, respectively. For MoS
2 the efficiencies are found to be 0.5, 3, 11 and 12% with 0.1, 0.3, 0.5, and 0.7 mL of MoS
2 solutions, respectively. Finally, in the case of WS
2 nanosheets, the efficiencies were 2, 7, 12 and 15% for 0.1, 0.3, 0.5 and 0.7 mL of the solutions, respectively.
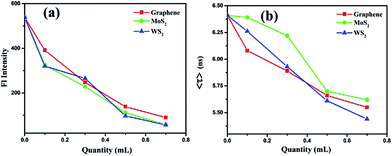 |
| Fig. 8 Variation of: (a) fluorescence intensity and (b) fluorescence lifetime of BSA molecules, in presence of different quantities of 2D nanosheets. | |
The energy transfer efficiency was also calculated using steady state fluorescence emission data of BSA, in absence as well as presence of different vdWs nanosheets, using following equation:
|
 | (4) |
where
FD is fluorescence intensity of BSA in absence of acceptors, while
FDA is intensity in presence of acceptors. The energy transfer efficiencies were found to be 27, 54, 74 and 83% for 0.1, 0.3, 0.5 and 0.7 mL of graphene solutions, respectively. In case of MoS
2, the efficiencies were 40, 58, 79 and 89% for 0.1, 0.3, 0.5 and 0.7 mL of MoS
2 solutions, respectively. Similarly, for WS
2, the efficiencies were found to be 40, 51, 82 and 89% for 0.1, 0.3, 0.5 and 0.7 mL of the solutions, respectively.
There is a difference in the energy transfer efficiency values for Fl intensity data and Fl lifetime data, which indicates possibilities of two kinds of Fl quenching mechanisms, viz., static and dynamic. The decrease in fluorescence lifetimes normally corresponds to the dynamic nature of Fl quenching, and possibility of the resonance energy transfer from BSA to 2D nanosheets, while quicker decay in the Fl intensity confirms the static nature of quenching. In Fig. 5, it has been shown that the fluorescence emission of BSA molecules overlaps with some fraction of the absorption spectrum of the 2D nanosheets. The overlapping fluorescence of BSA and absorption of nanosheets causes transfer of energy from BSA molecules to the nanosheets. When the BSA molecules are excited by 280 nm excitation light, they emit at higher wavelength of 350 nm. In presence of nanosheets, some fraction of this emission gets absorbed by the nanosheets, which results in decrease in fluorescence intensity, as well as the fluorescence lifetimes of the BSA molecules. At the same time, the BSA molecules are attached to the nanosheets by van der Waals interaction and form BSA-nanosheets complexes, which is not fluorescent. Therefore, there is a steeper decrease in the fluorescence intensity of BSA molecules, in presence of nanosheets, as compared to decrease in the Fl lifetimes. The quenching of Fl intensity as well as Fl lifetimes can play important role for detection of foreign molecules/ions. The addition of probe molecules/ions can affect the fluorescence intensity or fluorescence lifetimes, depending upon the nature of fluorescence intensity the BSA-TMDCs composites can be used as fluorescence turn ON/OFF sensor.
4 Conclusions
In summary, we have studied the resonance energy transfer between BSA molecules and two dimensional nanosheets of graphene, MoS2 and WS2. This study confirms that these nanosheets can be used as efficient acceptors for energy transfer studied in biomolecules, which can be further explored to study the effect of 2D nanomaterials on biomolecules, prior to application of these nanomaterials in biological applications. The resonance energy transfer can be explored further to detect molecules/ions which have effect on the RET efficiency. This study opens a new set of bio-nano composites, which can be used as sensor of molecules/ions.
Acknowledgements
AC and ASP acknowledge financial support from grant number BT/BI/03/004/2003(C) of Government of India, Ministry of Science and Technology, Department of Biotechnology, Bioinformatics division, and the DST-PURSE grant given to Jawaharlal Nehru University by the Department of Science and Technology, Government of India. Authors thank the AIRF, JNU for TEM, EDX, TRFS characterizations and the FIST (DST, Govt. of India) UFO scheme of IIT Delhi for Raman/PL facility.
References
- W. Chen, E. J. Santos, W. Zhu, E. Kaxiras and Z. Zhang, Nano Lett., 2013, 13, 509–514 CrossRef CAS PubMed
. - Y. Shi, J.-K. Huang, L. Jin, Y.-T. Hsu, S. F. Yu, L.-J. Li and H. Y. Yang, Sci. Rep., 2013, 3, 1839 CrossRef PubMed
. - K. F. Mak, K. He, C. Lee, G. H. Lee, J. Hone, T. F. Heinz and J. Shan, Nat. Mater., 2013, 12, 207–211 CrossRef CAS PubMed
. - L. Falkovsky and S. Pershoguba, Phys. Rev. B: Condens. Matter Mater. Phys., 2007, 76, 153410 CrossRef
. - Y. Ding, Y. Wang, J. Ni, L. Shi, S. Shi and W. Tang, Phys. B, 2011, 406, 2254–2260 CrossRef CAS
. - A. K. Geim and K. S. Novoselov, Nat. Mater., 2007, 6, 183–191 CrossRef CAS PubMed
. - B. Radisavljevic, A. Radenovic, J. Brivio, I. V. Giacometti and A. Kis, Nat. Nanotechnol., 2011, 6, 147–150 CrossRef CAS PubMed
. - H. Ramakrishna Matte, A. Gomathi, A. K. Manna, D. J. Late, R. Datta, S. K. Pati and C. Rao, Angew. Chem., 2010, 122, 4153–4156 CrossRef
. - Q. H. Wang, K. Kalantar-Zadeh, A. Kis, J. N. Coleman and M. S. Strano, Nat. Nanotechnol., 2012, 7, 699–712 CrossRef CAS PubMed
. - H. Hibino, H. Kageshima, M. Kotsugi, F. Maeda, F.-Z. Guo and Y. Watanabe, Phys. Rev. B: Condens. Matter Mater. Phys., 2009, 79, 125437 CrossRef
. - C. Lee, H. Yan, L. E. Brus, T. F. Heinz, J. Hone and S. Ryu, ACS Nano, 2010, 4, 2695–2700 CrossRef CAS PubMed
. - W. Zhao, R. M. Ribeiro, M. Toh, A. Carvalho, C. Kloc, A. Castro Neto and G. Eda, Nano Lett., 2013, 13, 5627–5634 CrossRef CAS PubMed
. - M. Chhowalla, H. S. Shin, G. Eda, L.-J. Li, K. P. Loh and H. Zhang, Nat. Chem., 2013, 5, 263–275 CrossRef PubMed
. - C. Zhang, Y. Chen, J.-K. Huang, X. Wu, L.-J. Li, W. Yao, J. Tersoff and C.-K. Shih, Nat. Commun., 2016, 7, 10349 CrossRef PubMed
. - A. Chakraborti, A. S. Patel, P. K. Kanaujia, P. Nath, G. V. Prakash and D. Sanyal, Phys. Lett. A, 2016, 380, 4057–4061 CrossRef CAS
. - K. F. Mak, C. Lee, J. Hone, J. Shan and T. F. Heinz, Phys. Rev. Lett., 2010, 105, 136805 CrossRef PubMed
. - R. Ganatra and Q. Zhang, ACS Nano, 2014, 8, 4074–4099 CrossRef CAS PubMed
. - J. Shakya, A. S. Patel, F. Singh and T. Mohanty, Appl. Phys. Lett., 2016, 108, 013103 CrossRef
. - A. Berkdemir, H. R. Gutiérrez, A. R. Botello-Méndez, N. Perea-López, A. L. Elías, C.-I. Chia, B. Wang, V. H. Crespi, F. López-Urías, J.-C. Charlier and A. Berkdemir, Sci. Rep., 2013, 3, 1755 CrossRef
. - S. Sampat, T. Guo, K. Zhang, J. A. Robinson, Y. Ghosh, K. P. Acharya, H. Htoon, J. A. Hollingsworth, Y. N. Gartstein and A. V. Malko, ACS Photonics, 2016, 3, 708–715 CrossRef CAS
. - H. Zang, P. K. Routh, Y. Huang, J.-S. Chen, E. Sutter, P. Sutter and M. Cotlet, ACS Nano, 2016, 10, 4790–4796 CrossRef CAS PubMed
. - D. Kufer, T. Lasanta, M. Bernechea, F. Koppens and G. Konstantatos, ACS Photonics, 2016, 3, 1324–1330 CrossRef CAS
. - D. Kufer, I. Nikitskiy, T. Lasanta, G. Navickaite, F. H. Koppens and G. Konstantatos, Adv. Mater., 2015, 27, 176–180 CrossRef CAS PubMed
. - K. M. Goodfellow, C. Chakraborty, K. Sowers, P. Waduge, M. Wanunu, T. Krauss, K. Driscoll and A. N. Vamivakas, Appl. Phys. Lett., 2016, 108, 021101 CrossRef
. - F. Prins, A. J. Goodman and W. A. Tisdale, Nano Lett., 2014, 14, 6087–6091 CrossRef CAS PubMed
. - P. R. Selvin, Nat. Struct. Biol., 2000, 7, 730–734 CrossRef CAS PubMed
. - K. Shankar, X. Feng and C. A. Grimes, ACS Nano, 2009, 3, 788–794 CrossRef CAS PubMed
. - R. Swathi and K. Sebastian, J. Chem. Phys., 2009, 130, 086101 CrossRef CAS PubMed
. - T. Sen, S. Mandal, S. Haldar, K. Chattopadhyay and A. Patra, J. Phys. Chem. C, 2011, 115, 24037–24044 CAS
. - J. Kuchlyan, N. Kundu, D. Banik, A. Roy and N. Sarkar, Langmuir, 2015, 31, 13793–13801 CrossRef CAS PubMed
. - W. Zhao, M. Fang, F. Wu, H. Wu, L. Wang and G. Chen, J. Mater. Chem., 2010, 20, 5817–5819 RSC
. - L. Yang, X. Cui, J. Zhang, K. Wang, M. Shen, S. Zeng, S. A. Dayeh, L. Feng and B. Xiang, Sci. Rep., 2014, 4, 5649 CrossRef CAS PubMed
. - T. P. Nguyen, W. Sohn, J. H. Oh, H. W. Jang and S. Y. Kim, J. Phys. Chem. C, 2016, 120, 10078–10085 CAS
. - N. Tayeh, T. Rungassamy and J. R. Albani, J. Pharm. Biomed. Anal., 2009, 50, 107–116 CrossRef CAS PubMed
. - A. S. Patel, H. Sahoo and T. Mohanty, Appl. Phys. Lett., 2014, 105, 063112 CrossRef
. - A. S. Patel, H. Sahoo and T. Mohanty, J. Fluoresc., 2016, 26, 1849–1855 CrossRef CAS PubMed
.
|
This journal is © The Royal Society of Chemistry 2017 |