DOI:
10.1039/C7RA02133J
(Paper)
RSC Adv., 2017,
7, 25325-25333
Synthesis and adsorption properties of [Cu(L)2(H2O)]H2[Cu(L)2(P2Mo5O23)]·4H2O/Fe3O4 nanocomposites†
Received
21st February 2017
, Accepted 12th April 2017
First published on 12th May 2017
Abstract
Multifunctional [Cu(L)2(H2O)]H2[Cu(L)2(P2Mo5O23)]·4H2O/Fe3O4 (HL = pyridine-2-carboxamide) nanocomposites were successfully synthesized by combining [Cu(L)2(H2O)]H2[Cu(L)2(P2Mo5O23)]·4H2O and Fe3O4 nanoparticles. The characterization was performed by Fourier transform infrared spectroscopy (FTIR), transmission electron microscopy (TEM), vibrating sample magnetometry (VSM), X-ray powder diffraction (XRD) and ultraviolet-visible light absorbance spectrometry (UV-vis). The XRD and TEM analyses reveal that the nanocomposites possess high crystallinity with an average particle size of ∼19.43 nm. The VSM and UV-vis demonstrate excellent superparamagnetic behavior and two well-behaved absorption bands of the nanocomposites. The adsorption activity of the nanocomposites was investigated using methylene blue, gentian violet, safranine T, fuchsin basic, methyl orange and Sudan red (III) as probe molecules, and the results reveal that the [Cu(L)2(H2O)]H2[Cu(L)2(P2Mo5O23)]·4H2O/Fe3O4 nanocomposites have selective adsorption behavior for organic dyes. The recycling performance was observed using basic fuchsin, and the results demonstrate that the nanocomposites exhibit good recyclability and high stability. The [Cu(L)2(H2O)]H2[Cu(L)2(P2Mo5O23)]·4H2O/Fe3O4 nanocomposites have a promising future for magnetic, optical and biomedical applications.
1 Introduction
In recent years, polyoxometalate/nanoparticle nanocomposites (POMs/NPs) based on polyoxometalates and nanoparticles have attracted considerable interest due to their unique properties resulting from the combination of the polyoxometalates and the nanoparticles. Research has been dedicated to the fabrication of POMs/NPs with a wide variety of POMs and NPs such as α-K6P2W18O62/Pt, Na12P2W15O56·18H2O/Pt, Na9EuW10O36·32H2O/Pt, (NH4)14NaP5W30O110·31H2O/Fe3O4, H3PW12O40/TiO2, H6P2Mo18O62/TiO2, [SiW9V3O40]7−/Bi2O3, and α-SiW12O404−/Ag to explore the synergistic properties arising from the nanocomposites.1–5 POMs/NPs have successfully been employed for biocatalysis,6 electrocatalysis,7 photocatalysis,8 oxidation of alkenes,9 bio-sensing,10 and medicinal chemistry.11 The assembly of POMs/NPs can provide a path for exhibiting their unique properties in many fields, and the complex structure makes them attractive for future study.
Polyoxometalates (POMs) are a well-known class of inorganic clusters consisting of a metal–oxygen framework, which have shown unique physicochemical properties and various applications owing to their highly electronegative and oxo-enriched surfaces, controllable shape and size, tunable acid–base, redox, magnetic, catalytic, and photochemical properties.12–17 On the other hand, magnetic Fe3O4 nanoparticles are most widely known for their exceptional physicochemical properties, low cost, non-toxic nature as well as environmentally benign for industrial scale synthesis of fine chemicals.18 The interdisciplinary integration of POMs and Fe3O4 nanoparticles has accelerated with fundamental curiosity and promising applications. Previously we have prepared Fe3O4 nanoparticles and their hybrids for the promising applications of heterogeneous catalysis, biological medicine, magnetofection, photo-therapy after magnetic separation, simultaneous photo-therapy and hyperthermia.19–22 Herein, we report a facile route for the synthesis of [Cu(L)2(H2O)]H2[Cu(L)2(P2Mo5O23)]·4H2O/Fe3O4 nanocomposites using the ultrasonic technique.
Now, a large number of dye-waste water discharged pose a significant threat to the water environment and human health due to their toxicity and even carcinogenicity.23 It is urgently-needed to find desirable adsorption materials, which not only reduce the pollutant organic dyes with high efficiency and low lost, but also realize selective separation and recovery. The use of nanomaterials as efficient catalysts in aqueous medium has attracted considerable interests.24,25 We investigated the adsorption of [Cu(L)2(H2O)]H2[Cu(L)2(P2Mo5O23)]·4H2O/Fe3O4 nanocomposites using organic dyes such as methylene blue, gentian violet, safranine T, fuchsin basic, methyl orange and Sudan red (III) as probe molecules. The result reveals that the prepared [Cu(L)2(H2O)]H2[Cu(L)2(P2Mo5O23)]·4H2O/Fe3O4 nanocomposites have selective adsorption behavior for organic dyes. The reusability of [Cu(L)2(H2O)]H2[Cu(L)2(P2Mo5O23)]·4H2O/Fe3O4 nanocomposites as adsorbent is studied as well, and the results demonstrate that the [Cu(L)2(H2O)]H2[Cu(L)2(P2Mo5O23)]·4H2O/Fe3O4 nanocomposites unveil good recyclability and high stability. Based on the experimental observations, the as-synthesized [Cu(L)2(H2O)]H2[Cu(L)2(P2Mo5O23)]·4H2O/Fe3O4 nanocomposites could be treated as a promising adsorbent candidate. The outcomes prove that the new [Cu(L)2(H2O)]H2[Cu(L)2(P2Mo5O23)]·4H2O/Fe3O4 nanocomposites could be of interest for fundamental studies and potential applications in magnetic, optical and biomedical filed.
2 Materials and methods
2.1 Materials
Copper(II) perchlorate hexahydrate (Cu(ClO4)2·6H2O, 98%), pyridine-2-carboxamide (C22H17ClN2, 98%), sodium molybdate dihydrate (Na2MoO4·2H2O, 99%) and phosphoric acid (H3PO4, 85%) were purchased from J&K Scientific Ltd. Iron(II) acetylacetonate (Fe(acac)2, 99.9%), poly(ethylene glycol)-block-poly(propylene glycol)-block-poly(ethylene glycol) (PEO–PPO–PEO, Mn = 5800), octyl ether (C8H17OC8H17, 99%), 1,2-hexadecanediol (C14H29CH(OH)CH2(OH), 90%), DMF (HCON(CH3)2, 99.5%) and solvents such as hexane and ethanol were purchased from Aldrich. All materials were used as received without further processing. Distilled water was used throughout.
2.2 Synthesis of Fe3O4 nanoparticles
The Fe3O4 nanoparticles were synthesized according to the reported literature method.19–22 A typical experiment was carried out in a 100 mL flask, 0.2541 g of iron(II) acetylacetonate, 0.7859 g of PEO–PPO–PEO as the surfactant, and 0.6468 g of 1,2-hexadecanediol were dissolved in 10 mL octyl ether under vigorous stirring. Subsequently, the reaction mixture was slowly heated to 125 °C within 1 h, homogenized for 1 h at 125 °C, then rapidly heated to 280 °C within 15 min and refluxed at the temperature for 1 h to complete the reaction. After cooling down to room temperature, the black precipitate products was washed with mixed solvents of ethanol/hexane (Vethanol/hexane is 2
:
1) several times, and re-dispersed in hexane for further use.
2.3 Synthesis of [Cu(L)2(H2O)]H2[Cu(L)2(P2Mo5O23)]·4H2O
The [Cu(L)2(H2O)]H2[Cu(L)2(P2Mo5O23)]·4H2O was synthesized using the method similar as the literature method.26–28 An aqueous solution containing Cu(ClO4)2·6H2O (0.093 g, 0.25 mmol) and pyridine-2-carboxamide (0.061 g, 0.5 mmol) was stirred at 50 °C for 0.5 h. After cooling to room temperature the solution was added to a 10 mL aqueous solution of Na2MoO4·2H2O (0.242 g, 1.0 mmol), the pH value was maintained at 3 by dropwise adding of concentrated H3PO4 under continuous stirring. Then the mixture was stirred for 0.5 h and then filtered. By filtering the mixture, the powders were stored for the following synthesis. The filtrate was allowed to evaporate at room temperature. After 3 days blue crystals suitable for X-ray studies were filtered off, washed with distilled water and dried in a desiccator at room temperature to give a yield of 38.4% based on Mo. Anal calc. for C24H34Cu2Mo5N8O32P2 (1615.30): C, 17.85; H, 2.12; N, 6.94; Cu, 7.87; Mo, 29.70. Found: C, 17.85; H, 2.14; N, 6.92; Cu, 7.88; Mo, 29.66. IR (KBr, cm−1): 3430 (w), 3082 (m), 1684 (s), 1633 (w), 1565 (m), 1497 (m), 1440 (m), 1307 (w), 1278 (w), 1169 (w), 1133 (m), 1058 (s), 1035 (m), 1003 (m), 928 (s), 904 (s), 758 (m), 682 (s), 525 (w).
2.4 Synthesis of [Cu(L)2(H2O)]H2[Cu(L)2(P2Mo5O23)]·4H2O/Fe3O4
[Cu(L)2(H2O)]H2[Cu(L)2(P2Mo5O23)]·4H2O/Fe3O4 nanocomposites were prepared by an ultrasonic procedure. A typical synthesis was carried out in a 50 mL beaker, Fe3O4 nanoparticles (5 mg), [Cu(L)2(H2O)]H2[Cu(L)2(P2Mo5O23)]·4H2O powders (50 mg) were added in a beaker containing water (10 mL) and ethanol (10 mL) in sequence and obtained an uniform and turbid liquid by ultrasound about 10 h. After the completion of the reaction, the resulting products was collected using a magnet putting on one side of the beaker to seperate them from the turbid liquid. The magnetic products were [Cu(L)2(H2O)]H2[Cu(L)2(P2Mo5O23)]·4H2O/Fe3O4 nanocomposites, which was washed with water several times.
2.5 Structural characterization and measurements of the nanocomposites
The structures of the synthesized Fe3O4 nanoparticles, [Cu(L)2(H2O)]H2[Cu(L)2(P2Mo5O23)]·4H2O and [Cu(L)2(H2O)]H2[Cu(L)2(P2Mo5O23)]·4H2O/Fe3O4 nanocomposites were analyzed by X-ray powder diffraction (XRD, X'Pert Pro) and transmission electron microscopy (TEM, JEOL2010F). The UV-vis spectra were measured by an ultraviolet-visible light absorbance spectrometry (UV-vis, HitachiU4100). In the Fourier transform infrared spectroscopy (FTIR) studies, Fe3O4 nanoparticles, [Cu(L)2(H2O)]H2[Cu(L)2(P2Mo5O23)]·4H2O and [Cu(L)2(H2O)]H2[Cu(L)2(P2Mo5O23)]·4H2O/Fe3O4 nanocomposites were separately crushed with a pestle in an agate mortar. The individually crushed material was mixed with KBr in about 1
:
100 proportion. The mixture was then compressed into a 2 mm semitransparent disk by applying a force of 10 T for 2 min. The Fourier transform infrared spectra were recorded in the wavelength range of 500–4000 cm−1 using an Avatar 360 FTIR spectrometer (FTIR, Nicolet Company, USA). The magnetic properties were subsequently investigated by a vibrating sample magnetometry (VSM, Lakeshore 7300).
2.6 Crystallography
Crystallographic data were collected with a Bruker SMART-CCD APEX II diffractometer with graphite-monochromated Mo Kα radiation (λ = 0.71073 Å). The structures were solved by direct methods and refined by full-matrix least squares on F2 with anisotropic displacement parameters for all non-hydrogen atoms using SHELXTL.29 The hydrogen atoms are added in idealized geometrical positions. Crystal data, experimental details, and refinement results are listed in Table 1.
Table 1 Summary of crystal data and refinement results for [Cu(L)2(H2O)]H2[Cu(L)2(P2Mo5O23)]·4H2O
Crystal data |
[Cu(L)2(H2O)]H2[Cu(L)2(P2Mo5O23)]·4H2O |
Empirical formula |
C24H34Cu2Mo5N8O32P2 |
fw |
1615.30 |
Cryst syst |
Triclinic |
Space group |
P![[1 with combining macron]](https://www.rsc.org/images/entities/char_0031_0304.gif) |
T (K) |
296(2) |
a (Å) |
10.187(3) |
b (Å) |
10.918(3) |
c (Å) |
20.739(5) |
α (deg) |
82.052(4) |
β (deg) |
89.700(5) |
γ (deg) |
88.467(5) |
V (Å3) |
2283.6(10) |
Z |
2 |
Dc (g cm−3) |
2.335 |
μ (mm−1) |
2.423 |
Crystal size (mm) |
0.27 × 0.18 × 0.15 |
θ (deg) |
1.983–25.098 |
F(000) |
1556 |
hkl range |
−12 ≤ h ≤ 9, |
−12 ≤ k ≤ 12, |
−24 ≤ l ≤ 24 |
GOF on F2 |
0.999 |
Rint |
0.0433 |
No. param |
671 |
Refl. measured/unique |
8012/5810 |
R1, wR2 [I ≥ 2σ(I)] |
0.0551, 0.1396 |
R1, wR2 (all data) |
0.0818, 0.1601 |
Δρmax, Δρmin (e Å−3) |
1.821, −1.678 |
2.7 Characterization of adsorption activity
The adsorption activities of the nanocomposites were performed in the dark by measuring the adsorption rate of different dye solutions at room temperature. The dye solutions such as methylene blue, gentian violet, safranine T and fuchsin basic, methyl orange and Sudan red (III) were prepared with a concentration of 15 mg L−1 by dissolving the dye powder in distilled water. The reaction was conducted with 5 mg of [Cu(L)2(H2O)]H2[Cu(L)2(P2Mo5O23)]·4H2O/Fe3O4 nanocomposites which is the adsorbents dispersed in 30 mL of 15 mg L−1 each dye solutions. After stirring, the samples (volume of each is about 5 mL) were drawn out from the reaction beaker every a period of time, centrifuged at 4500 rpm for 10 min and filtered to remove the particles. The filtrate was then analyzed using a UV-vis spectrophotometer (Beijingpuxitongyong TU-1900) to measure the absorption of different dyes.
2.8 Characterization of desorption, reusability activities
In order to investigate the regeneration of the adsorbents during the adsorption experiments, a solvent DMF is chosen as the eluents. The stability and reusability of the nanocomposites on removing fuchsin basic were investigated through cycle tests. The fuchsin basic solution was prepared with a concentration of 15 mg L−1 by dissolving the dye powder in distilled water. The reaction was conducted with 50 mg of the adsorbents dispersed in 20 mL of 15 mg L−1 fuchsin basic. After each cycle, the adsorbents were separated from aqueous solution by a piece of magnet and washed with a solvent DMF about 20 mL at room temperature to remove the adsorbed fuchsin basic. Then, the regenerated [Cu(L)2(H2O)]H2[Cu(L)2(P2Mo5O23)]·4H2O/Fe3O4 nanocomposites were added to the initial dye solution of fuchsin basic (20 mL of 15 mg L−1). The concentrations of fuchsin basic solution were determined by measuring the absorbance through a UV-vis spectrophotometer (Beijingpuxitongyong TU-1900).
3 Results and discussion
3.1 Crystal structure description of compound [Cu(L)2(H2O)]H2[Cu(L)2(P2Mo5O23)]·4H2O
Selected bond distances and angles of [Cu(L)2(H2O)]H2[Cu(L)2(P2Mo5O23)]·4H2O are given in Table 2. The molecular structures along with the atom numbering scheme are depicted in Fig. 1.
Table 2 Selected bond lengths (Å) and angles (deg) for [Cu(L)2(H2O)]H2[Cu(L)2(P2Mo5O23)]·4H2O
[Cu(L)2(H2O)]H2[Cu(L)2(P2Mo5O23)]·4H2O |
Cu(1)–O(1) |
1.967(6) |
Cu(2)–O(3) |
1.929(6) |
Cu(1)–O(2) |
1.959(6) |
Cu(2)–O(4) |
1.949(6) |
Cu(1)–N(1) |
1.964(7) |
Cu(2)–O(5) |
2.236(6) |
Cu(1)–N(3) |
1.987(8) |
Cu(2)–N(5) |
1.985(7) |
Cu(1)–O(1W) |
2.171(6) |
Cu(2)–N(7) |
1.958(7) |
O(1)–Cu(1)–N(3) |
97.1(3) |
O(3)–Cu(2)–O(4) |
168.9(3) |
O(1)–Cu(1)–O(1W) |
98.6(3) |
O(3)–Cu(2)–O(5) |
94.5(3) |
O(2)–Cu(1)–O(1) |
164.5(3) |
O(3)–Cu(2)–N(5) |
82.4(3) |
O(2)–Cu(1)–N(1) |
96.0(3) |
O(3)–Cu(2)–N(7) |
95.4(3) |
N(1)–Cu(1)–N(3) |
82.6(3) |
O(4)–Cu(2)–O(5) |
96.5(3) |
O(2)–Cu(1)–O(1W) |
96.8(3) |
O(4)–Cu(2)–N(5) |
98.2(3) |
N(1)–Cu(1)–O(1) |
82.5(3) |
O(4)–Cu(2)–N(7) |
82.7(3) |
N(1)–Cu(1)–N(3) |
173.2(3) |
N(5)–Cu(2)–O(5) |
81.7(3) |
N(1)–Cu(1)–O(1W) |
92.1(3) |
N(7)–Cu(2)–O(5) |
105.7(3) |
N(3)–Cu(1)–O(1W) |
94.6(3) |
N(7)–Cu(2)–N(5) |
172.4(3) |
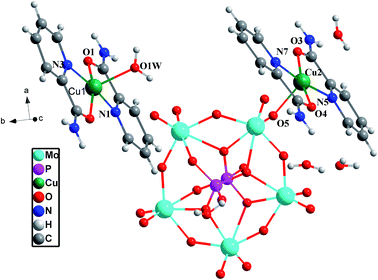 |
| Fig. 1 Structure of [Cu(L)2(H2O)]H2[Cu(L)2(P2Mo5O23)]·4H2O with atomic numbering scheme. | |
Single-crystal X-ray structural analysis exhibits that [(CuL2)(H2O)]H2[(CuL2) (P2Mo5O23)]·4H2O crystallizes in P
space group. In addition, Fig. 1a shows that compound [(CuL2)(H2O)]H2[(CuL2) (P2Mo5O23)]·4H2O consists of [(CuL2) (P2Mo5O23)]4− polyanion, [CuL2]2+ complex fragments and four water molecules in the structural unit of compound [(CuL2)(H2O)]H2[(CuL2) (P2Mo5O23)]·4H2O. As is well known, the P2Mo5 cluster can be viewed as two {PO4} tetrahedral capping either side of an irregular ring of five distorted {MoO6} octahedral linked by one corner-shared and four edge-shared contacts. Each phosphate subunit shares three oxo-groups with the molybdate ring. One of these oxo-groups adopts a μ2-bridging mode, linking one molybdenum atom and a phosphorus atom; while the other two adopt a μ3-bridging mode, linking two molybdenum atoms and a phosphorus atom.30 Each of two Cu atoms adopts a five-coordinated distorted {CuN2O3} square-pyramidal geometry surrounded by two pyridine-2-carboxamide ligands and one O atom from P2Mo5 cluster or one water, respectively. The Cu(1) is coordinated by two N (amide) atoms with Cu–N bond lengths of 1.968(9)–1.970(9) Å, two O (amide) atoms with Cu–O bond lengths of 1.958(7)–1.960(7) Å and one O (aqua) atom with Cu–Ow bond lengths of 2.169(7) Å. The Cu(2) atom is coordinated by two N (amide) atoms with Cu–N bond lengths of 1.961 (9)–1.978(9) Å, one O atom of {MoO6} octahedron with Cu–O bond lengths of 2.231(7) Å, and two O (amide) atom with Cu–O bond lengths of 1.930(7)–1.945(7) Å.31
3.2 IR spectroscopy
Fig. 2 compares the FTIR spectra of Fe3O4 nanoparticles, [Cu(L)2(H2O)]H2[Cu(L)2(P2Mo5O23)]·4H2O and [Cu(L)2(H2O)]H2[Cu(L)2(P2Mo5O23)]·4H2O/Fe3O4 nanocomposites. In Fig. 2b, the Fe3O4 nanoparticles show one strong characteristic band at the position of 600 cm−1 which is associated with the stretching vibration mode of Fe–O.32–34 The IR spectrum of [Cu(L)2(H2O)]H2[Cu(L)2(P2Mo5O23)]·4H2O has been depicted in Fig. 2c. It is evident that the characteristic band at 3378 cm−1 is attributed to the O–H stretching vibration of water, while the peaks at 3072 cm−1 are related to the ν(N–H) of pyridine-2-carboxamide. A series of strong bands in the range of 1684–1133 cm−1 is also associated with pyridine-2-carboxamide. Bands in the region of 1120–1008 cm−1 are assigned to ν(P–O) stretching vibration.35,36 The peaks at 908 cm−1 and 672 cm−1 are attributed to ν(Mo = Od) and ν(Mo–O–Mo), respectively.36 As given in Fig. 2a, these characteristic vibration and bending modes reappear in the FTIR spectrum of the [Cu(L)2(H2O)]H2[Cu(L)2(P2Mo5O23)]·4H2O/Fe3O4, but instead shifting to the positions of 3389 cm−1 for the O–H stretching vibration and 3174 cm−1 for the ν(N–H) of pyridine-2-carboxamide. The result is that Fe3O4 and [Cu(L)2(H2O)]H2[Cu(L)2(P2Mo5O23)]·4H2O are existed in [Cu(L)2(H2O)]H2[Cu(L)2(P2Mo5O23)]·4H2O/Fe3O4 nanocomposites, which demonstrates the synthesis of [Cu(L)2(H2O)]H2[Cu(L)2(P2Mo5O23)]·4H2O/Fe3O4 nanocomposites.
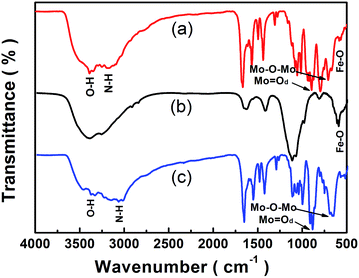 |
| Fig. 2 FTIR spectra of [Cu(L)2(H2O)]H2[Cu(L)2(P2Mo5O23)]·4H2O/Fe3O4 (a), Fe3O4 (b) and [Cu(L)2(H2O)]H2[Cu(L)2(P2Mo5O23)]·4H2O (c). | |
3.3 UV-vis spectroscopy
The optical property of [Cu(L)2(H2O)]H2[Cu(L)2(P2Mo5O23)]·4H2O/Fe3O4 were assessed by UV-visible absorption spectroscopy. Fig. 3a–c shows the UV-vis spectra of [Cu(L)2(H2O)]H2[Cu(L)2(P2Mo5O23)]·4H2O/Fe3O4 nanocomposites, [Cu(L)2(H2O)]H2[Cu(L)2(P2Mo5O23)]·4H2O and Fe3O4 dispersed in H2O. Apparently, in Fig. 3b, [Cu(L)2(H2O)]H2[Cu(L)2(P2Mo5O23)]·4H2O shows two of absorption bands, and the absorption bands observed around 211 nm and 264 nm, which are attributed to the charge-transfer of Ot → Mo and Ob → Mo, respectively.37 As given in Fig. 3c, in the ultraviolet and visible light area, Fe3O4 nanoparticles have no obvious absorption bands. Clearly, in Fig. 3a, there are two kinds of absorption bands, one at about 210 nm and the other at about 265 nm, both from [Cu(L)2(H2O)]H2[Cu(L)2(P2Mo5O23)]·4H2O, the peak pattern of [Cu(L)2(H2O)]H2[Cu(L)2(P2Mo5O23)]·4H2O/Fe3O4 nanocomposites is similar to that of [Cu(L)2(H2O)]H2[Cu(L)2(P2Mo5O23)]·4H2O, and the position of the two peaks is nearly alike, and coming about shift slightly is due to the existence of Fe3O4 nanoparticles.
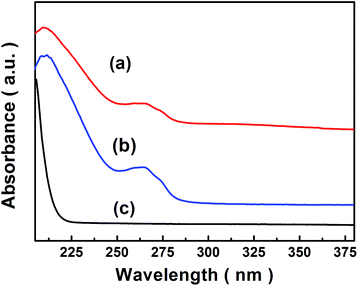 |
| Fig. 3 UV-visible absorbance spectra of [Cu(L)2(H2O)]H2[Cu(L)2(P2Mo5O23)]·4H2O/Fe3O4 (a), [Cu(L)2(H2O)]H2[Cu(L)2(P2Mo5O23)]·4H2O (b) and Fe3O4 (c) dispersed in H2O. | |
3.4 TEM morphology and particle size distribution of [Cu(L)2(H2O)]H2[Cu(L)2(P2Mo5O23)]·4H2O/Fe3O4
The morphology, nanostructure, particle size and size distribution of the prepared [Cu(L)2(H2O)]H2[Cu(L)2(P2Mo5O23)]·4H2O/Fe3O4 nanocomposites were recorded by TEM and HRTEM. As given in Fig. 4a, the obtained [Cu(L)2(H2O)]H2[Cu(L)2(P2Mo5O23)]·4H2O/Fe3O4 nanocomposites are virtually uniform and nearly spherical in shape with seldom aggregation. The histograms in Fig. 4b shows the size distribution of [Cu(L)2(H2O)]H2[Cu(L)2(P2Mo5O23)]·4H2O/Fe3O4 nanocomposites, which are reasonably described by the Gaussian function, showing tight size distribution with average sizes of approximately 19.43 nm in diameter. Fig. 4c represents the HRTEM image of a single [Cu(L)2(H2O)]H2[Cu(L)2(P2Mo5O23)]·4H2O/Fe3O4 nanocomposite. As labeled, the spacing of 2.85 Å corresponds to the (220) reflection of the Fe3O4 phase. Moreover, with a higher magnification than in Fig. 4a of the same sample, a thin layer with ∼1 nm uneven thickness is clearly observed in Fig. 4c. We think that this similarly may be the example of observation of such kind of [Cu(L)2(H2O)]H2[Cu(L)2(P2Mo5O23)]·4H2O/Fe3O4 core–shell structure.38,39
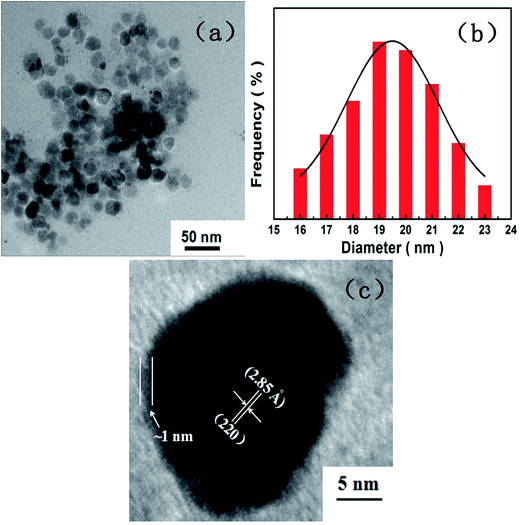 |
| Fig. 4 TEM analyses (a), particle size histogram with Gaussian fit (b) and HRTEM analyses of [Cu(L)2(H2O)]H2[Cu(L)2(P2Mo5O23)]·4H2O/Fe3O4 nanocomposites (c). | |
3.5 XRD patterns
As shown in Fig. 5, the structure of the [Cu(L)2(H2O)]H2[Cu(L)2(P2Mo5O23)]·4H2O/Fe3O4 nanocomposites was recorded by XRD and analyzed together with the results of Fe3O4 and [Cu(L)2(H2O)]H2[Cu(L)2(P2Mo5O23)]·4H2O. Fig. 5a represents the diffraction pattern obtained from the [Cu(L)2(H2O)]H2[Cu(L)2(P2Mo5O23)]·4H2O/Fe3O4 nanocomposites, in match to the standard diffraction peaks of the corresponding Fe3O4 (JCPDS No.88-0315), the diffraction peaks positioning at 30.2°, 35.6°, 43.2°, 57.3° and 62.7° are indexed to the (220), (311), (400), (511) and (440) planes of the Fe3O4 nanoparticles. Fig. 5b represents the diffraction pattern obtained from the [Cu(L)2(H2O)]H2[Cu(L)2(P2Mo5O23)]·4H2O, the diffraction peaks positioning at 8.8°, 12.9°, 23.8° and 27.1° also appear in Fig. 5a which are indicated by arrows. In conclusion, [Cu(L)2(H2O)]H2[Cu(L)2(P2Mo5O23)]·4H2O and Fe3O4 are included in [Cu(L)2(H2O)]H2[Cu(L)2(P2Mo5O23)]·4H2O/Fe3O4 nanocomposites.
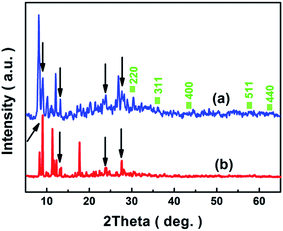 |
| Fig. 5 XRD patterns for [Cu(L)2(H2O)]H2[Cu(L)2(P2Mo5O23)]·4H2O/Fe3O4 (a), [Cu(L)2(H2O)]H2[Cu(L)2(P2Mo5O23)]·4H2O (b) and squares for the JCPDS of Fe3O4. | |
3.6 Magnetic property of [Cu(L)2(H2O)]H2[Cu(L)2(P2Mo5O23)]·4H2O/Fe3O4
The magnetic property of [Cu(L)2(H2O)]H2[Cu(L)2(P2Mo5O23)]·4H2O/Fe3O4 nanocomposites was studied by VSM. Fig. 6a and b show the hysteresis curves of Fe3O4 nanoparticles and [Cu(L)2(H2O)]H2[Cu(L)2(P2Mo5O23)]·4H2O/Fe3O4 nanocomposites at 300 K, respectively. It is clear that [Cu(L)2(H2O)]H2[Cu(L)2(P2Mo5O23)]·4H2O/Fe3O4 nanocomposites show superparamagnetic behavior with a coercivity tend to ∼9.0 Oe and magnetization of ∼2.37 emu g−1 comparing with a coercivity tend to ∼0 Oe and magnetization of ∼16.10 emu g−1 of Fe3O4 nanoparticles.
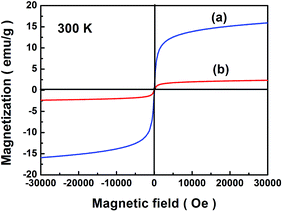 |
| Fig. 6 Magnetic measurements of Fe3O4 nanoparticles (a) and [Cu(L)2(H2O)]H2[Cu(L)2(P2Mo5O23)]·4H2O/Fe3O4 nanocomposites (b). | |
3.7 Separation and aggregation process of [Cu(L)2(H2O)]H2[Cu(L)2(P2Mo5O23)·4H2O/Fe3O4
Fig. 7 visually demonstrates the separation and aggregation process of the [Cu(L)2(H2O)]H2[Cu(L)2(P2Mo5O23)]·4H2O/Fe3O4 nanocomposites in water.40 Under the influence of an external magnetic field, [Cu(L)2(H2O)]H2[Cu(L)2(P2Mo5O23)]·4H2O/Fe3O4 nanocomposites in water change from a purplish grey, homogeneous dispersion (Fig. 7a) to a clear, transparent solution, with the nanocomposites collected by a piece of magnet (Fig. 7b). The collected nanocomposites can be easily and reversibly dispersed by agitation after removal of the magnetic field and the above process can be repeated. The finding that all [Cu(L)2(H2O)]H2[Cu(L)2(P2Mo5O23)]·4H2O/Fe3O4 nanocomposites as prepared could be collected by a magnet, leaving no free [Cu(L)2(H2O)]H2[Cu(L)2(P2Mo5O23)]·4H2O visible. In other words, we performed magnetic separation and found that all nanocomposites were collected by the magnet because the [Cu(L)2(H2O)]H2[Cu(L)2(P2Mo5O23)]·4H2O/Fe3O4 nanocomposites are magnetic and there is no more nanoparticles left-over, so nonmagnetic [Cu(L)2(H2O)]H2[Cu(L)2(P2Mo5O23)]·4H2O are not present in the samples.
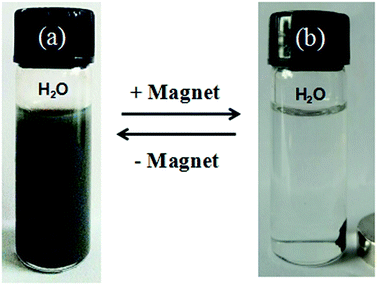 |
| Fig. 7 Photo images of solvent of H2O dispersion-collection process of the [Cu(L)2(H2O)]H2[Cu(L)2(P2Mo5O23)]·4H2O/Fe3O4 nanocomposites. | |
3.8 Adsorption properties
Organic dyes such as methylene blue, gentian violet, safranine T and fuchsin basic are important organic dyes which are widely used as a colorant in textiles and food stuffs the dyes are harmful if swallowed by human beings and animals, causing irritation to the skin, eye and respiratory tract.41–43 Their breakdown products in the water may be toxic, carcinogenic or mutagenic to life forms, and they have been shown to cause retching, stun, cyanosis, jaundice and tissue necrosis in human. Therefore, an effective and optimal strategy to degrade the dyes rapidly must be developed. In the design of an economical wastewater disposal system, fast adsorption rate is also an important parameter for design and synthesis of the efficient adsorbent.
We investigated the adsorption of [Cu(L)2(H2O)]H2[Cu(L)2(P2Mo5O23)]·4H2O/Fe3O4 nanocomposites using organic dyes such as methylene blue, gentian violet, safranine T, fuchsin basic, methyl orange and Sudan red (III) as probe molecules. Fig. 8 show that [Cu(L)2(H2O)]H2[Cu(L)2(P2Mo5O23)]·4H2O/Fe3O4 nanocomposites were able to adsorb the methylene blue, gentian violet, safranine T and fuchsin basic efficiently in the dark. The intensity of UV-visible absorption peak of each dyes decreased with the increase of time due to the existence of [Cu(L)2(H2O)]H2[Cu(L)2(P2Mo5O23)]·4H2O/Fe3O4 nanocomposites, and the adsorption efficiency of methylene blue, gentian violet, safranine T and fuchsin basic were 79%, 91%, 64% and 83% in 12 min, 45 min, 60 min and 120 min, respectively. In addition, the peak patterns of each dyes are sightly similar and the peak positions are the same except gentian violet which may be due to itself of gentian violet. Fig. 9 show that [Cu(L)2(H2O)]H2[Cu(L)2(P2Mo5O23)]·4H2O/Fe3O4 nanocomposites were not able to adsorb the methyl orange, Sudan red (III) efficiently in the dark. The intensity of UV-visible absorption peak of methyl orange and Sudan red (III) both are almost constant. The reason of the same adsorbent with different effects on removal of dyes is related to the structure of the dye molecules and the structure of the [Cu(L)2(H2O)]H2[Cu(L)2(P2Mo5O23)]·4H2O/Fe3O4 nanocomposites.
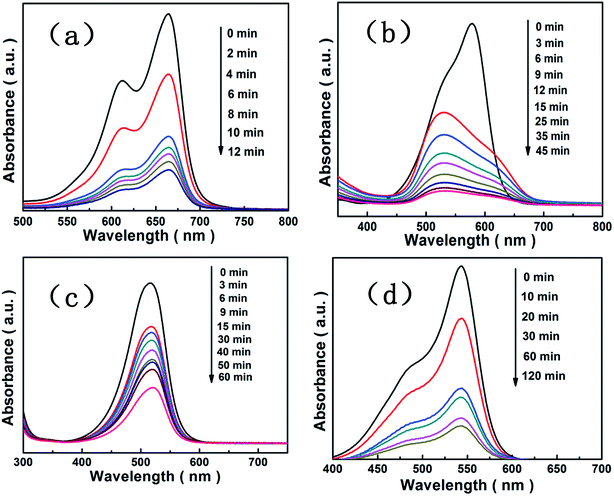 |
| Fig. 8 Adsorption spectra of the methylene blue solution (a), the gentian violet solution (b), the safranine T solution (c) and the fuchsin basic solution (d) under the dark in presence of the [Cu(L)2(H2O)]H2[Cu(L)2(P2Mo5O23)]·4H2O/Fe3O4 nanocomposites. | |
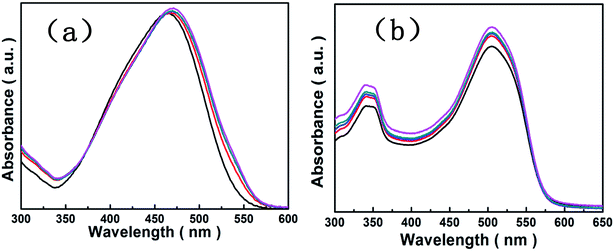 |
| Fig. 9 Adsorption spectra of the methyl orange solution (a) and the Sudan red (III) solution (b) under the dark in presence of the [Cu(L)2(H2O)]H2[Cu(L)2(P2Mo5O23)]·4H2O/Fe3O4 nanocomposites. | |
POMs, as an outstanding family of metal-oxide clusters with controllable shape and size, highly electronegative, and oxo-enriched surfaces, are expected to exhibit good adsorption towards cationic dyes.44 Firstly, POMs are a kind of hydrophilic metal-oxo cluster compounds. The hydrophilic/hydrophobic property of the framework is modulated by encapsulating the POM molecules, which allows the ingress and egress of the dye molecules. Secondly, POMs with a large number of negative charges encapsulated in the neutral framework may have a stronger force with the positive charges of dyes. The approving result is that [Cu(L)2(H2O)]H2[Cu(L)2(P2Mo5O23)]·4H2O/Fe3O4 nanocomposites exhibit rapid adsorption efficiency and high uptake capacity towards methylene blue, gentian violet, safranine T and fuchsin basic than methyl orange, Sudan red (III), which is in accord with literature.45 The magnetic [Cu(L)2(H2O)]H2[Cu(L)2(P2Mo5O23)]·4H2O/Fe3O4 nanocomposites combining [Cu(L)2(H2O)]H2[Cu(L)2(P2Mo5O23)]·4H2O and Fe3O4 nanoparticles could be collected in adsorption process preferably.
3.9 Stability and reusability behaviours
The stability and reusability of the nanocomposites are another important standard for practical application. It can be seen from the process of magnetic aggregation and dispersion that the [Cu(L)2(H2O)]H2[Cu(L)2(P2Mo5O23)]·4H2O/Fe3O4 are not the mixture of [Cu(L)2(H2O)]H2[Cu(L)2(P2Mo5O23)]·4H2O and Fe3O4 nanoparticles but a new nanocomposite, although it is still a challenge to identify the interaction between POM species and magnetic particles. The in-depth study is on the way. As shown in Fig. 10, the IR patterns of the adsorbents regenerated from adsorption and desorption experiments match well with the as-synthesized product, indicating that the structure of the nanocomposites remain intact, which confirms their good stability and recyclability. The properties are significant in improving the use of material without loss of their structure and reducing the adsorption cost. In a word, the nanocomposites are of great significance for the practical use of the absorbents and exhibit good reproducibility.
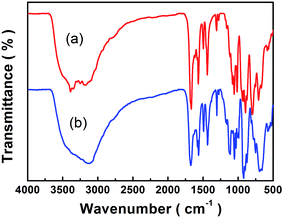 |
| Fig. 10 FTIR spectra as-synthesized (a) and after adsorption and desorption tests (b) of the [Cu(L)2(H2O)]H2[Cu(L)2(P2Mo5O23)]·4H2O/Fe3O4 nanocomposites. | |
After adsorption experiments, the adsorbed fuchsin basic can be removed by a solvent DMF. In Fig. 11, it shows that the photographs of three adsorption and desorption cycles. When a solution of DMF was added, digital images show that fuchsin basic molecules in the nanocomposites can be rapidly released. Then, the regenerated [Cu(L)2(H2O)]H2[Cu(L)2(P2Mo5O23)]·4H2O/Fe3O4 nanocomposites were added to the initial fuchsin basic solution (20 mL of 15 mg L−1), the adsorbents are still capable of removing fuchsin basic up to 91%, 93% and 90% over one cycles, two cycle, three cycle, respectively, as shown in Fig. 12. This means that the dye release is also an ion-exchange process, which also further demonstrate that ionic interaction between dyes and anionic framework is main determinant for selective adsorption and separation of dyes.46
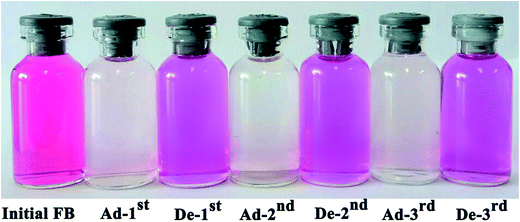 |
| Fig. 11 The photographs of three adsorption and desorption cycles, the first cycle: Ad-1st and De-1st; the second cycle: Ad-2nd and De-2nd; the third cycle: Ad-3rd and De-3rd. | |
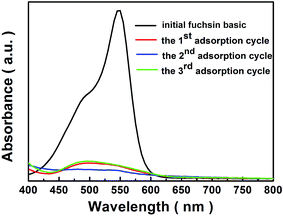 |
| Fig. 12 The UV-vis spectra of initial fuchsin basic, the 1st adsorption cycle, the 2nd adsorption cycle and the 3rd adsorption cycle. | |
The new [Cu(L)2(H2O)]H2[Cu(L)2(P2Mo5O23)]·4H2O/Fe3O4 nanocomposites have been successfully synthesized, and were adopted as adsorbents for the removal of dyes in aqueous solution. Interestingly, they not only exhibit rapid adsorption rate and high uptake capacity towards fuchsin basic, but also realize the recycling performance. This work opens up a useful, economical adsorption and desorption, which can improve the recycling of raw material in wastewater.
4 Conclusions
In summary, we have successfully synthesized the nanocomposites of [Cu(L)2(H2O)]H2[Cu(L)2(P2Mo5O23)]·4H2O/Fe3O4 that combining [Cu(L)2(H2O)]H2[Cu(L)2(P2Mo5O23)]·4H2O and Fe3O4 nanoparticles. The morphology and structural analyses reveal the narrow particle size distribution with an average diameter ∼19.43 nm and high crystallinity of the nanocomposite. The FTIR and XRD assessments confirm [Cu(L)2(H2O)]H2[Cu(L)2(P2Mo5O23)]·4H2O and Fe3O4 nanoparticles are existed in [Cu(L)2(H2O)]H2[Cu(L)2(P2Mo5O23)]·4H2O/Fe3O4 nanocomposites. The UV-vis measurement shows the well-defined optical absorption with the nanocomposites dispersed in H2O. The magnetic characterization shows that [Cu(L)2(H2O)]H2[Cu(L)2(P2Mo5O23)]·4H2O/Fe3O4 nanocomposites have superparamagnetic behavior. The dispersion-collection processes in H2O of the [Cu(L)2(H2O)]H2[Cu(L)2(P2Mo5O23)]·4H2O/Fe3O4 nanocomposites were demonstrated for application readiness. [Cu(L)2(H2O)]H2[Cu(L)2(P2Mo5O23)]·4H2O/Fe3O4 nanocomposites have selective adsorption behavior for organic dyes. Meanwhile, the stable and reusable [Cu(L)2(H2O)]H2[Cu(L)2(P2Mo5O23)]·4H2O/Fe3O4 nanocomposites were found to be a good adsorbent and could be employed as an effective adsorbent applicable in adsorption field.
Acknowledgements
This work was supported in part by the National Natural Science Foundation of China (no. 5117206 and 21671055) and the Key Scientific Research Projects of Henan Province Colleges and Universities, Foundation of Education Department of Henan Province, China (no. 16A150002).
References
- Z. Ma, Q. Liu, Z. M. Cui, S. W. Bian and W. G. Song, J. Phys. Chem. C, 2008, 112, 8875–8880 CAS.
- M. Huang, L. Bi, Y. Shen, B. Liu and S. Dong, J. Phys. Chem. B, 2004, 108, 9780–9786 CrossRef CAS.
- Z. Sun, L. Xu, W. Guo, B. Xu, S. Liu and F. Li, J. Phys. Chem. C, 2010, 114(11), 5211–5216 CAS.
- C. Li, K. P. O'Halloran, H. Ma and S. Shi, J. Phys. Chem. B, 2009, 113, 8043–8048 CrossRef CAS PubMed.
- J. Kim, L. Lee, B. K. Niece, J. X. Wang and A. A. Gewirth, J. Phys. Chem. B, 2004, 108, 7927–7933 CrossRef CAS.
- D. An, A. Ye, W. Deng, Q. Zhang and Y. Wang, Chem.–Eur. J., 2012, 18, 2938–2947 CrossRef CAS PubMed.
- S. Li, X. Yu, G. Zhang, Y. Ma, J. Yao and P. de Oliveira, Carbon, 2011, 49, 1906–1911 CrossRef CAS.
- A. Troupis, A. Hiskia and E. Papaconstantinou, Angew. Chem., Int. Ed., 2002, 41(11), 1911–1914 CrossRef CAS PubMed.
- E. Tebandeke, C. Coman, K. Guillois, G. Canning, E. Ataman, J. Knudsen, L. R. Wallenberg, H. Ssekaalo, J. Schnadt and O. F. Wendt, Green Chem., 2014, 16, 1586–1593 RSC.
- A. Babakhanian, S. Kaki, M. Ahmadi, H. Ehzari and A. Pashabadi, Biosens. Bioelectron., 2014, 60, 185–190 CrossRef CAS PubMed.
- H. K. Daima, P. R. Selvakannan, R. Shukla, S. K. Bhargava and V. Bansal, PLoS One, 2013, 8, e79676 CAS.
- W. Zheng, L. Yang, Y. Liu, X. Qin, Y. Zhou, Y. Zhou and J. Liu, Sci. Technol. Adv. Mater., 2014, 15, 035010 CrossRef PubMed.
- Y. Kikukawa, Y. Kuroda, K. Yamaguchi and N. Mizuno, Angew. Chem., 2012, 124, 2484–2487 CrossRef.
- U. Kortz, A. Müeller, J. van Slageren, J. Schnack, N. S. Dalal and M. Dressel, Coord. Chem. Rev., 2009, 253, 2315–2327 CrossRef CAS.
- H. N. Miras, J. Yan, D. L. Long and L. Cronin, Chem. Soc. Rev., 2012, 41, 7403–7430 RSC.
- K. M. Seemann, M. Luysberg, Z. Révay, P. Kudejova, B. Sanz, N. Cassinelli, A. Loidl, K. Llicic, G. Multhoff and T. E. Schmid, J. Controlled Release, 2015, 197, 131–137 CrossRef CAS PubMed.
- Z. You, Y. Chen, T. Liu, Z. Yang, F. Xie and Y. Sun, Inorg. Chim. Acta, 2014, 421, 160–168 CrossRef CAS.
- S. Saif, A. Tahir and Y. Chen, Nanomaterials, 2016, 6, 209 CrossRef PubMed.
- H. L. Liu, J. H. Wu, J. H. Min and Y. K. Kim, J. Alloys Compd., 2012, 537, 60–64 CrossRef CAS.
- H. L. Liu, J. H. Wu, J. H. Min, X. Y. Zhang and Y. K. Kim, Mater. Res. Bull., 2013, 48, 551–558 CrossRef CAS.
- H. L. Liu, P. Hou, W. X. Zhang and J. H. Wu, Colloids Surf., 2010, 356(1), 21–27 CrossRef CAS.
- H. L. Liu, S. P. Ko, J. H. Wu, M. H. Jung, J. H. Min, J. H. Lee, B. H. An and Y. K. Kim, J. Magn. Magn. Mater., 2007, 310(2), e815–e817 CrossRef CAS.
- S. Lin, Z. Song, G. Che, A. Ren, P. Li, C. Liu and J. Zhang, Microporous Mesoporous Mater., 2014, 193, 27–34 CrossRef CAS.
- A. Dandia, V. Parewa, A. K. Jain and K. S. Rathore, Green Chem., 2011, 13, 2135 RSC.
- B. Adhikari, G. Palui and A. Banerjee, Soft Matter, 2009, 5, 3452–3460 RSC.
- J. Thomas and A. Ramanan, Inorg. Chim. Acta, 2011, 372, 243–249 CrossRef CAS.
- M. Ðaković, Z. Popović, G. Giester and M. Rajić-Linarić, Polyhedron, 2008, 27, 210–222 CrossRef.
- H. Paşaoğlu, S. Güven, Z. Heren and O. Büyükgüngör, J. Mol. Struct., 2006, 794, 270–276 CrossRef.
- G. M. Sheldrick, Acta Crystallogr., Sect. A: Found. Crystallogr., 2008, 64(1), 112–122 CrossRef CAS PubMed.
- J. X. Meng, Y. Lu, Y. G. Li, H. Fu and E. B. Wang, CrystEngComm, 2011, 13, 2479–2486 RSC.
- H. J. Jin, B. B. Zhou, Y. Yu, Z. F. Zhao and Z. H. Su, CrystEngComm, 2011, 13, 585–590 RSC.
- R. Yuvakkumar and S. I. Hong, Adv. Mater. Res., 2014, 1051, 39–42 CrossRef.
- Y. P. Yew, K. Shameli, M. Miyake, N. Kuwano, N. B. B. A. Khairudin, S. E. B. Mohamad and K. X. Lee, Nanoscale Res. Lett., 2016, 11(1), 1–7 CrossRef CAS PubMed.
- S. H. Ahmadi, P. Davar and A. Manbohi, Iran. J. Chem. Chem. Eng., 2016, 35, 63–73 CAS.
- Z. L. Li, Y. Wang, L. C. Zhang, J. P. Wang, W. S. You and Z. M. Zhu, Dalton Trans., 2014, 43, 5840–5846 RSC.
- B. D'Cruz, J. Samuel and L. George, Thermochim. Acta, 2014, 596, 29–36 CrossRef.
- L. H. Wei, Z. L. Wang, J. W. Zhao and J. P. Wang, Chin. J. Struct. Chem., 2010, 29, 784–788 CAS.
- G. Zhang, B. Keita, A. Dolbecq, P. Mialane, F. Sécheresse, F. Miserque and L. Nadjo, Chem. Mater., 2007, 19, 5821–5823 CrossRef CAS.
- A. Neyman, L. Meshi, L. Zeiri and I. A. Weinstock, J. Am. Chem. Soc., 2008, 130, 16480–16481 CrossRef CAS PubMed.
- H. L. Liu, J. H. Wu, J. H. Min, P. Hou, A. Y. Song and Y. K. Kim, Nanotechnology, 2010, 22, 055701 CrossRef PubMed.
- S. Thakur, S. Pandey and O. A. Arotiba, Carbohydr. Polym., 2016, 153, 34–46 CrossRef CAS PubMed.
- K. A. G. Gusmão, L. V. A. Gurgel, T. M. S. Melo and L. F. Gil, J. Environ. Manage., 2013, 118, 135–143 CrossRef PubMed.
- X. Xu, B. Bai, H. Wang and Y. Suo, J. Phys. Chem. Solids, 2015, 87, 23–31 CrossRef CAS.
- A. X. Yan, S. Yao, Y. G. Li, Z. M. Zhang, Y. Lu, W. L. Chen and E. B. Wang, Chem.–Eur. J., 2014, 20, 6927–6933 CrossRef CAS PubMed.
- F. Y. Yi, W. Zhu, S. Dang, J. P. Li, D. Wu, Y. H. Li and Z. M. Sun, Chem. Commun., 2015, 51, 3336–3339 RSC.
- J. S. Qin, S. R. Zhang, D. Y. Du, P. Shen, S. J. Bao, Y. Q. Lan and Z. M. Su, Chem.–Eur. J., 2014, 20, 5625–5630 CrossRef CAS PubMed.
|
This journal is © The Royal Society of Chemistry 2017 |