DOI:
10.1039/C7RA02092A
(Paper)
RSC Adv., 2017,
7, 21422-21429
Effect of post-heat treatment on the photocatalytic activity of titanium dioxide nanowire membranes deposited on a Ti substrate
Received
20th February 2017
, Accepted 10th April 2017
First published on 18th April 2017
Abstract
Titanium dioxide nanowire membranes have been synthesized by a hydrothermal growth on the surfaces of Ti substrates in a 12 M NaOH aqueous solution at 160 °C for 24 h, followed by ion-exchange with 0.5 M HCl aqueous solution and subsequent heat treatment such as calcination or a second hydrothermal treatment. The as-prepared TiO2 nanowires as well as their precursor were characterized by field emission scanning electron microscopy (FE-SEM), thermogravimetry-differential thermal analysis (TG-DTA), X-ray diffraction (XRD), the Brunauer–Emmett–Teller (BET) method, ultraviolet-visible (UV-Vis) spectrophotometry, transmission electron microscopy (TEM) and energy dispersive X-ray (EDX) spectrometry. FE-SEM observations indicated that the TiO2 nanowires were 50–250 nm in diameter and up to several dozens of microns in length. TG-DTA and XRD results demonstrated that the crystalline phases of the nanowires obtained from calcinations of their precursor at different temperatures above 350 °C consisted mostly of anatase. BET, UV-Vis, TEM and EDX results showed that the nanowires obtained upon calcination of their precursor at 550 °C had the greatest degradation efficiency for Rhodamine-B, and that, at the same temperature of 250 °C, the hydrothermal treatment process of TiO2-precursor nanowires had a more significant effect on the photocatalytic activity of the resulting TiO2 nanowires than the calcination.
Introduction
Due to economic disparity, rapid urbanization, industrialization, and population growth,1 there are growing concerns about water availability and the strategies necessary to deliver potable water with the growing demands for clean water sources.2,3 Industrial waste water is an extremely serious problem of water pollution,2 as it contains various compositions of constituent pollutants which are often unknown. Usually, these pollutants cannot be self-cleaned in the environment,4 the artificial treatment of which is complicated and costly.
TiO2 is a wide-band semiconductor material with gaps of 3.2 eV for anatase and 3.0 eV for rutile.5–7 Degussa P25, a commercial TiO2 polycrystalline nanopowder,8 is chemically inert and cost-effective, and exhibits satisfactory photocatalytic activity.9 Therefore, it has widely been used in photocatalytic water-treatment, although the separation of TiO2 nanoparticles from the treated water has high energy costs.10 Recently, more focus has been directed to the technology of preparing TiO2 nanowire/nanobelt thin membranes, which show superior performance to TiO2 nanoparticles.11,12 For example, TiO2 nanowires/nanobelts can minimize the release of nanomaterials into effluents during their self-assembled formation of membranes.13 As more and more studies are focused on this new technology,14 various methods to obtain TiO2 nanowire/nanobelt membranes have been developing and improving.15 In the past decade, great efforts1–19 were focused on the effects of hydrothermal parameters on the compositions and morphologies and clarification of the sequential events in the formation process of titanate nanotubes/nanowires/nanobelts. However, to our knowledge, nobody particularly investigated the preparation of TiO2 nanowires via a second hydrothermal process.
In this work, we report the synthesis of TiO2-precursor nanowires by a hydrothermal growth on the surfaces of Ti substrates,20 followed by the use of different heat-treatment methods (i.e. calcination and a second hydrothermal) on the synthesized precursor to obtain TiO2 nanowires. Analysis of the experimental results demonstrates that the TiO2 nanowires obtained upon the second hydrothermal treatment of their precursor possess higher photocatalytic activity than upon the calcination.
Experimental
Materials
Titanium foil with purity greater than 99.5%, NaOH, HCl and ethanol were purchased from Sinopharm Chemical Reagent Co., Ltd, China and used as starting materials without further purification, and deionized water purified by ion exchange was used for solution preparation and synthesis.
Preparation
TiO2 nanowires were synthesized in a NaOH aqueous solution via hydrothermal growth on the surfaces of titanium foil (1 mm thick). In detail, a 20 × 20 mm2 piece of titanium foil was washed sequentially with ethanol and deionized water, then placed in a 100 mL Teflon-lined pressure vessel filled with 50 mL of 12 M NaOH aqueous solution. After heating the vessel at 160 °C for 24 h, a thick, white cotton-like membrane of sodium titanate nanowires was obtained on each side of the titanium foil surface.6 The nanowires were randomly oriented and entangled together (i.e. intertwined) to form macroporous structured membranes. The titanium foil covered with the nanowire membranes was washed in deionized water, and then immersed in a 0.5 M HCl aqueous solution at 50 °C for 12 h to effectively transform Na2Ti3O7 nanowires into H2Ti3O7 nanowires via ion-exchange of Na+ with H+.6 The membranes were then peeled off the Ti foil using a knife and again washed in deionized water until the pH value of the washing solution was neutral. Finally, both the TiO2-precursor nanowire membranes obtained were air dried at 70 °C overnight (denoted as Sample A), and then heated with different post-heat treatments to obtain the resulting TiO2 nanowire membranes. The different post-heat treatments included direct calcination at different temperatures (250, 350, 450, 550 and 650 °C) for 2 h (denoted as Samples B, C, D, E and F, respectively), and a second hydrothermal treatment at 250 °C for 2 h (denoted as Sample G) which was similar to the previous hydrothermal procedure except without NaOH.
Characterizations
Surface morphologies of the as-prepared H2Ti3O7 precursor nanowires and of the TiO2 nanowires upon the post-heat treatments of the precursor nanowires were analyzed on a FEI, Quanta 450 field-emission scanning electron microscope (FE-SEM), operating at 7 kV. Data, obtained from a Shimadzu DTG-50H thermogravimetry-differential thermal analysis (TG-DTA) thermal analyzer, revealed the phase transformation temperature ranges required for designing the sintering program. Powder X-ray diffraction (XRD) patterns of TiO2 nanowires as well as of their precursor nanowires were recorded on a Bruker, D8 Advance powder X-ray diffractometer with CuKα radiation (Si internal standard method), at a 2θ scan range of 5–60° and a scan speed of 2° min−1. The specific surface areas of the samples were determined with Quantachrome instruments, NOVA 2200 specific surface area analyzer by the Brunauer–Emmett–Teller (BET) method using the N2 adsorption data (as shown in Table 1). Absorption spectra of the samples were recorded with a Hitachi, U-3900 ultraviolet-visible (UV-Vis) spectrophotometer using BaSO4 as a reflectance standard. High resolution transmission electron microscopy (HRTEM) observation and selected area electron diffraction (SAED) were carried out with a JOEL JEM 2100 microscope. To prepare the HRTEM specimens, the powder samples were first dispersed ultrasonically in acetone, and then one drop of the suspension was placed on a carbon-film-coated copper grid and allowed to dry in air before the specimens were finally transferred onto the microscope. An Oxford energy dispersive X-ray (EDX) spectrometer was used to analyze the elemental composition of the TiO2 nanowire membranes prepared.
Table 1 Specific surface areas measured by the BET method for the H2Ti3O7 precursor (Sample A), the TiO2 nanowires synthesized by calcinations for 2 h at temperatures of 250, 350, 450, 550 and 650 °C (Samples B, C, D, E and F, respectively) and by hydrothermal treatment for 2 h at 250 °C (Sample G)
Samples |
Specific surface areas (m2 g−1) |
A |
19.205 |
B |
18.706 |
C |
17.926 |
D |
16.823 |
E |
15.621 |
F |
12.988 |
G |
21.868 |
Photocatalytic activity measurements
The photocatalytic activity of the TiO2 nanowire membranes was evaluated by monitoring the degradation efficiency of a Rhodamine-B (RhB) dye aqueous solution (20 mL of 5 mg L−1). All the tests were performed using a 300 W Xenon lamp, which emits both ultraviolet (UV) and visible light; during the assay, 2 mL of the solution was extracted at 10 min intervals, the RhB concentration of which was then measured using a UV-Vis spectrophotometer (JASCO, V-560). The degradation efficiency was calculated as (C0 − C)/C0, where C0 is the initial concentration of the RhB solution (i.e. 5 mg L−1) and C the remaining concentration of RhB upon degradation for 1 h.
Results and discussion
Effect of post-heat treatment on specific surface area of TiO2 nanowires
Fig. 1 shows the FE-SEM images of the as-synthesized TiO2-precursor nanowires (Fig. 1a) as well as of the TiO2 nanowires obtained upon two different post-heat treatments (i.e. calcinations and hydrothermal) of the precursor at the same temperature and time (250 °C for 2 h). As expected, fibrous structures with a typical diameter of 50–250 nm and a length of several dozens of microns were observed for both the TiO2 and its precursor nanowires. We found that the structure of both the TiO2 nanowires underwent little changes after post-heat treatments of their precursor. Compared with the TiO2 nanowires obtained from the direct calcination of the precursor (Sample B) (Fig. 1b and d), the TiO2 nanowires obtained from the hydrothermal treatment of the precursor (Sample G) were quite cleaner with less contamination on their surfaces and smaller in diameter (Fig. 1c and e). At some locations in Sample B, a fraction of the TiO2 nanowires seemed to be joined (or aggregated) with each other, which might decrease their specific surface area (Fig. 1d); apparently, this aggregation behavior occurred little in Sample G. Moreover, the nanowires in Sample G exhibited a distinctly smaller diameter than those in Sample B. These jointly demonstrated that the specific surface area of the TiO2 nanowires was largely dictated by the preparation method used, in that the hydrothermal treatment was beneficial to attain TiO2 nanowires with smaller diameter (Fig. 1e vs. d) and thus higher specific surface area (cf. Table 1).
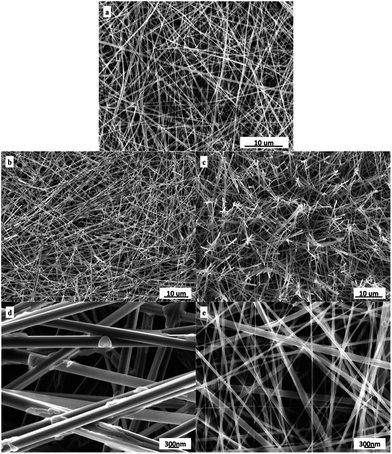 |
| Fig. 1 Morphologies observed by FE-SEM of (a) the as-synthesized H2Ti3O7 (precursor of TiO2 nanowires) and the TiO2 nanowires prepared from (b and d) the calcination and from (c and e) the hydrothermal treatment of the TiO2 precursor nanowires at 250 °C for 2 h. | |
Effect of post-heat treatment on crystalline structure of TiO2 nanowires
To optimize the calcination temperature in the post-heat treatment, TG-DTA combined analysis was performed on the TiO2 precursor nanowires, which are shown in Fig. 2. The initial weight loss until ca. 145 °C in the TG curve might be attributed to the evaporation of adsorbed water onto H2Ti3O7; accordingly, the DTA curve showed a significant endothermic peak ranging from ca. 60 to 145 °C (peaking at 130.5 °C). The rest of weight loss occurred between 145 and 700 °C, which may primarily be due to the decomposition of H2Ti3O7 or further TiO2 and hydrated water, followed by the crystallization of the TiO2 nanowires. As the temperature was increased to ∼503.2 °C, a weak exothermic peak was noted, probably representing the crystallization that led to the formation of the TiO2-B. Furthermore, an exothermic peak appeared at 647.8 °C, which was likely caused by the formation of the crystal structure of anatase phase. More specifically, the TG curve clearly demonstrates that there was a noticeable weight loss between 145 and 300 °C during the decomposition process, which presumably resulted from the dehydration of H2Ti3O7, as typically represented by eqn (1).
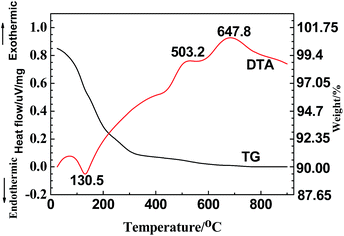 |
| Fig. 2 Typical TG-DTA curves of the H2Ti3O7 (precursor of TiO2) nanowires. | |
According to eqn (1), the weight loss due to the H2Ti3O7 transformation into TiO2 should be 6.7%; however, the actual weight loss from room temperature to 300 °C was ∼9% measured from the TG curve, which means that the content of the adsorbed water evaporated between 60 and 145 °C was about 2.3% in the TiO2-precursor nanowires. At higher temperatures above 300 °C, the TG curve nearly leveled off due to the negligible weight loss during crystal growth and/or transformation of TiO2 nanowires.
To determine the structure of crystalline phase(s) of the H2Ti3O7/TiO2 nanowires, we performed XRD on the TiO2 precursor (H2Ti3O7) nanowires (Sample A) (Fig. 3a), as well as on the TiO2 nanowires obtained from calcinations of the TiO2 precursor at 350 °C (Sample C), 450 °C (Sample D), 550 °C (Sample E) and 650 °C (Sample F) (Fig. 3b–e, respectively), as shown in Fig. 3. Fig. 3a indicates that the main crystallite of the precursor was the layered H2Ti3O7 structure(s),21 which could be converted to a TiO2 polymorph (TiO2-B) upon post-heat treatments22 as shown in Fig. 3b; further, the broadening of the diffraction peaks of the TiO2-precursor nanowires in Fig. 3a was presumably due to the small nanometer size of their crystallites, and/or to the bending of some atomic crystallographic plane(s) of the nanowires.21 Two new broad peaks emerged at 2θ values of 28.5° and 43.8°, revealing the collapse (i.e. destruction) of the layered structure(s) of hydrogen titanates and the formation of TiO2-B23,24 (Fig. 3b and c). Compared with Fig. 3b and c, there appeared a pronounced new peak at 2θ = 37.721° in Fig. 3d, which, matching the (004) lattice planes of anatase, indicates the transformation from TiO2-B to anatase.25,26 In addition, the obvious diffraction peaks at 25.221°, 36.861°, 37.721° and 38.641° in Fig. 3d, respectively, corresponded to the (101), (103), (004) and (112) lattice planes of anatase, indicating the coexistence of TiO2-B and anatase at 550 °C. These agree well with the TG-DTA observation (from Fig. 2) where a small exothermic peak located at 503.2 °C occurred in the DTA curve, which is characteristic of the TiO2-B crystal formation. Finally, with the further development of phase transformation upon an increase in the sintering temperature, as shown in Fig. 3e, the sharpened peak at 25.221° was attributable to the strongest reflection from lattice-plane (101) of the TiO2-anatase phase, which suggests that Sample F primarily contained highly crystalline anatase.
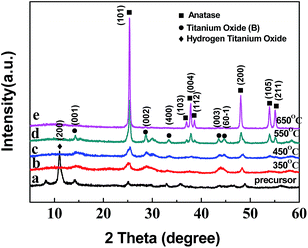 |
| Fig. 3 XRD patterns of (a) the H2Ti3O7 (precursor of TiO2) nanowires and the TiO2 nanowires prepared from calcinations of the TiO2 precursor for 2 h at different temperatures (°C): (b) 350; (c) 450; (d) 550; (e) 650. | |
In addition, Fig. 4 shows XRD patterns a and b of the TiO2 nanowires prepared from different post-heat treatments (calcination and hydrothermal, respectively) of the TiO2 precursor at 250 °C for 2 h. The sharper diffraction peaks in Fig. 4b demonstrate that, in comparison to the calcination (Fig. 4a), the hydrothermal treatment was more favorable for the formation and growth of anatase crystals.
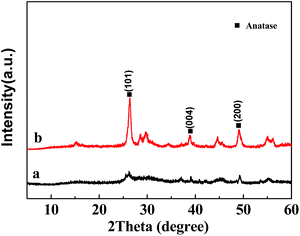 |
| Fig. 4 XRD patterns of the TiO2 nanowires prepared by (a) the calcination and (b) the hydrothermal treatment of the H2Ti3O7 (precursor of TiO2) nanowires at 250 °C for 2 h. | |
Effect of post-heat treatment on UV-Vis absorption
Fig. 5a shows the UV-Vis absorbance spectra of Samples C, D, E and F. The band gap energy (Eg) for direct gap semiconductors can be evaluated by using the Tauc equation,27,28 |
(αEp)0.5 = k(Ep − Eg)
| (2) |
where α is the absorption coefficient, Ep the discrete photon energy (hν), and k absorption constants for indirect transitions.29,30 Plots of (αEp)0.5 versus Ep for the TiO2 nanowire membranes are shown in Fig. 5b; the corresponding Eg values were 2.98, 2.94,2.90 and 2.93 eV, respectively. When the temperature was increased from 350 to 550 °C, the absorbance of UV and visible light was steadily enhanced (Fig. 5a). As shown in Fig. 3, the major crystalline phase of the TiO2 nanowires prepared from calcination of the TiO2 precursor at 350–550 °C was TiO2-B phase, with a small amount of anatase phase, and the content of anatase increased with an increase in the sintering temperature. Therefore, the increase in the absorption intensities of the TiO2 nanowires can be attributed to the increase in the content of anatase phase, which is consistent with other studies.2,5–8,15 With a further increase of the calcination temperature from 550 to 650 °C, Sample F against Sample E possessed a significantly higher level of anatase (cf. Fig. 3e vs. d), nevertheless with a relative reduction in its specific surface area (cf. Table 1) which results in decreases in the UV-Vis absorption intensities as shown in Fig. 5a (550 °C vs. 650 °C). Given that a higher calcination temperature induces an increase in the content of anatase while a decrease in the specific surface area, we can come to the conclusion that the higher UV-Vis absorbances of Sample F than Sample E may be determined by the interactions of the two competing effects: that is, the effect of specific-surface-area decrease predominated over that of anatase-content increase.
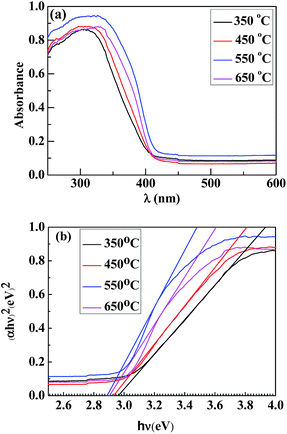 |
| Fig. 5 (a) UV-Vis absorption spectra and (b) plots of (αhν)2 vs. hν for the TiO2 nanowires prepared from calcinations of the H2Ti3O7 (precursor of TiO2) nanowires for 2 h at different temperatures of 350, 450, 550 and 650 °C. | |
The UV-Vis absorption spectra (Fig. 6a) were taken of the TiO2 nanowires obtained by the different post-heat treatments of the TiO2 precursor at 250 °C, with Spectrum 1 representing the calcination and Spectrum 2 the hydrothermal treatment, from which the band-gap energy profiles were calculated (as shown in Fig. 6b). It is seen that, compared to the calcination method, the hydrothermal treatment of the TiO2 precursor resulted in a stronger light-absorption ability (Fig. 6a) and a narrower band gap (Fig. 6b) of the TiO2 nanowires, which could be attributed to a higher content of their highly crystalline anatase phase (Fig. 4) as well as their higher specific surface area (Fig. 1 and Table 1).
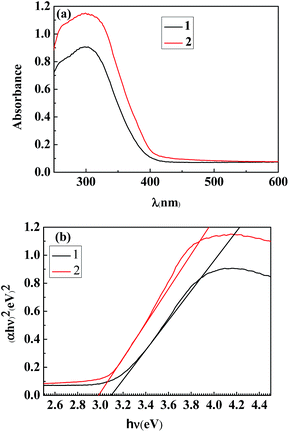 |
| Fig. 6 (a) UV-Vis absorption spectra and (b) plots of (αhν)2 vs. hν for the TiO2 nanowires obtained by (1) the calcination and (2) the hydrothermal treatment of the H2Ti3O7 (precursor of TiO2) nanowires at 250 °C for 2 h. | |
Effect of post-heat treatment on photocatalytic activity of TiO2 nanowires
The degradation curves (Fig. 7a), as well as kinetic curves (Fig. 7b) analyzed from Fig. 7a, of the TiO2 nanowires were measured by monitoring the photocatalytic degradation of the RhB aqueous solution with Samples C, D, E and F, respectively, as the photocatalysts. Prior to the measurements, the solution containing the catalyst sample was stirred at room temperature in the dark for 10 min to establish an adsorption/desorption equilibrium between the RhB-dye and the sample surfaces, and the photo-degradation efficiencies of the samples were found to be 44%, 50%, 85% and 64%, respectively. The kinetic analysis by Fig. 7b indicates that the photocatalytic reaction of Samples C, D and F followed a pseudo-first-order reaction,31 and the rate constants of RhB degradation by them were estimated to be 0.01127 min−1, 0.01515 min−1, and 0.03228 min−1, respectively. It is notable that the photocatalytic activity of Sample E, exhibiting a maximum in the degradation efficiency, did not follow a pseudo-first-order mechanism. According to the previous studies,32 the photocatalytic activity of TiO2 can be identified as significant for aqueous pharmaceutical solutions interacting with nanomaterials through surface adsorption and photocatalytic degradation of the former by the latter; further, the surface adsorption for most of pharmaceuticals follows a pseudo-second-order model, whereas the photocatalytic degradation complies with a pseudo-first-order model.33 As held by the researchers, adsorption by TiO2 nanomaterials actually is not only surface adsorption behavior, which also depends on complicated factors such as surface charge of nanomaterials, adsorbent masses, ambient pH values, etc.; generally, the adsorptive capacity of TiO2 nanomaterials is nearly saturated during 30 min.34 But notably, the time intervals of adsorption/desorption equilibrium between the RhB-dye and the surfaces of Samples C, D, E and F were invariably 10 min in this study, which indicates that it was possible for some kinds of samples (Samples C, D and F) to obtain an adsorption/desorption equilibrium in the 10 min, whereas that the other sample (Sample E) did not. Due to its unsaturated adsorptive capacity, the photocatalytic activity of Sample E after the treatment in the dark for 10 min might probably be determined by a coupling of the surface adsorption (ruled by the pseudo-second-order model) and the photocatalytic degradation (ruled by the pseudo-first-order model). The synergistic decoloration of the RhB aqueous solution by both the surface adsorption and the photocatalytic degradation resulted in the inappropriateness to plot linearly as a pseudo-first-order model. However, the data beyond 30 min shown in Fig. 7b, which reflects the near saturation of adsorptive capacity of Sample E, can be well fitted by the pseudo-first-order formula.
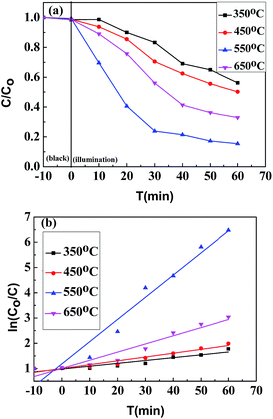 |
| Fig. 7 (a) Degradation curves and (b) kinetic curves of a RhB aqueous solution of 5 mg L−1 that is photocatalytically degraded by the TiO2 nanowires prepared from the calcinations of the H2Ti3O7 (precursor of TiO2) nanowires for 2 h at different temperatures of 350 (Sample C), 450 (Sample D), 550 (Sample E) and 650 °C (Sample F). | |
Again, degradation curves (Fig. 8a), and kinetic curves (Fig. 8b) obtained from Fig. 8a, were plotted to compare RhB degradation efficiencies by the TiO2 nanowires obtained by the different post-heat treatments (calcinations vs. hydrothermal) of the TiO2 precursor at 250 °C. Similarly, the hydrothermal kinetic plot of Sample G (Fig. 8b) was apparently nonlinear, the rationale behind which was also the synergistic decoloration of the RhB aqueous solution through both the surface adsorption and the photocatalytic degradation of RhB by Sample G. Compared to the calcination at 250 °C, the hydrothermal treatment at the same temperature led to a higher RhB degradation efficiency (60% vs. 38%), which was comparable to that (64%) of Sample F that was formed from calcination of the precursor at 650 °C. This probably is due to the fact that the sample treated with the hydrothermal method (Sample G) had a higher proportion of anatase phase (cf. Fig. 4) as well as a higher specific surface area (cf. Fig. 1 and Table 1), which favored an enhancement of photocatalytic activity relative to the calcinated sample (Sample B) (cf. Fig. 8).
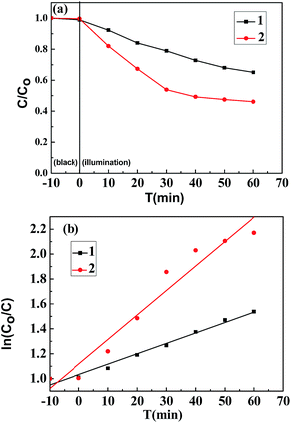 |
| Fig. 8 (a) Degradation curves and (b) kinetic curves of a RhB aqueous solution of 5 mg L−1 that is photocatalytically degraded by the TiO2 nanowires prepared from (1) the calcinations (Sample B) and (2) the hydrothermal treatment (Sample G) of the H2Ti3O7 (precursor of TiO2) nanowires at 250 °C for 2 h. | |
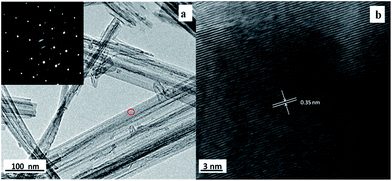 |
| Fig. 9 (a) A TEM image of Sample G (prepared by hydrothermal treatment of the TiO2 precursor at 250 °C), where the inset shows an SAED image of the TiO2 nanowires; (b) the corresponding HRTEM image of the area red-circled in (a), from which an interspacing of 0.35 nm was resolved for the (101) crystallographic plane of TiO2 anatase phase. | |
Moreover, TEM and HRTEM were also used to study the fine structure of Sample G as shown in Fig. 9a and b, respectively. Fig. 9a unveils a typical morphology of the TiO2 nanowires: the average diameter of the nanowire sample was approximately 60 nm; and the corresponding SAED (the inset in Fig. 9a) reveals that the TiO2 nanowires had a near single crystal anatase structure, which was consistent with the XRD result (Pattern b of Fig. 4). Furthermore, the near single-crystalline structure was further confirmed by the HRTEM image (shown in Fig. 9b): Fig. 9b shows that the distance between adjacent lattice fringes was ca. 0.35 nm, which could be assigned to the interplanar spacing of the TiO2 anatase (101) planes; this corroborates the formation of anatase TiO2 nanowires in our experiments.
In addition, as shown in Fig. 10, Sample G against Sample B had a remarkably higher ratio of the Ti and O combined content to the Na level; this suggests that the hydrothermal method, making the Na+-ion impurity diffuse effectively into the water phase, increased the purity of the formed TiO2 nanowires, which also contributed to the increase of their photocatalytic activity.
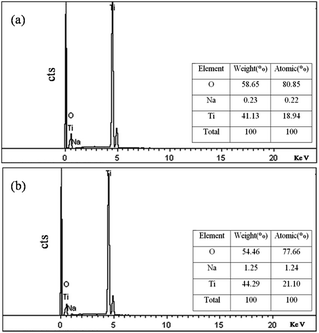 |
| Fig. 10 Energy dispersive X-ray (EDX) spectra of the TiO2 nanowires prepared from the different post-heat treatments of the H2Ti3O7 (precursor of TiO2) nanowires at 250 °C for 2 h: (a) the hydrothermal treatment; (b) the calcination. | |
Conclusions
This work has detailed the synthesis of TiO2 nanowires (membranes) on a titanium foil substrate via a three-step route: (1) hydrothermal growth of Na2Ti3O7 from Ti substrate in a concentrated NaOH aqueous solution; (2) exchange of Na+ ions with H+ to form H2Ti3O7; and (3) post-heat treatment (i.e. annealing) of the H2Ti3O7 precursor by calcination at different temperatures (250, 350, 450, 550 and 650 °C) or by hydrothermal treatment (250 °C).
Morphological characterizations by the FE-SEM have revealed that the TiO2 nanowires synthesized from the hydrothermal have a significantly smaller diameter and hence a higher specific surface area than those from the calcination. The TG-DTA, TEM and XRD results have indicated that a calcination temperature of ca. 550 °C maximally contributes to the formation and growth of anatase crystals with a relative reduction in the specific surface area of the TiO2 nanowires, and that the hydrothermal favors an enhancement of TiO2-nanowire crystallinity, especially for the anatase phase. UV-Vis absorption spectra and their associated band-gap energy profiles have uncovered that the TiO2 nanowires, prepared from calcination of the TiO2 precursor at 550 °C, have maxima in UV-Vis absorption intensities as well as the narrowest band-gap among those calcinated at the other temperatures. EDX spectrometry has demonstrated that, due to the diffusion of Na+-impurity into the water phase, the hydrothermal forms much purer TiO2 nanowires than the calcination.
The photocatalytic degradation of an RhB aqueous solution has been investigated by the TiO2 nanowires synthesized from the calcination and the hydrothermal methods. Assays have demonstrated that the TiO2 nanowires obtained by calcination at an optimum temperature of 550 °C exhibit a maximum in photocatalytic activity compared with the other TiO2 nanowires calcinated at 350, 450 and 650 °C, presumably owing to a combination of the effects of anatase-content increase and of specific-surface-area decrease with increasing the calcination temperature. At the same temperature of 250 °C, the hydrothermal produces TiO2 nanowires of markedly higher photocatalytic activity than the calcinations, primarily due to their significantly higher specific surface area, degree of crystallinity, and purity (from a much decreased Na+-impurity content).
Acknowledgements
The authors gratefully acknowledge the financial support for this work from the State Key Development Program for Basic Research of China (Contract no. 2010CB635107), the National Natural Science Foundation of China (NSFC) (Contract no. 51004046, 51202064 and 51302073), the Natural Science Foundation of the Hubei province of China (Contract no. 2010CDB05806), the Wuhan Youth Chenguang Program of Science and Technology (Contract no. 2013070104010016) and Middle-aged and Young program of Educational Commission of Hubei Province (Q20101409).
References
- A. Kubacka, M. Fernández-García and G. Colón, Chem. Rev., 2011, 112, 1555 CrossRef PubMed.
- M. A. Henderson and I. Lyubinetsky, Chem. Rev., 2013, 113, 4428 CrossRef CAS PubMed.
- Y. Du, R. Z. Chen, J. F. Yao and H. T. Wang, J. Alloys Compd., 2013, 551, 125 CrossRef CAS.
- Y. Liao, C. Xie, Y. Liu, H. Chen, H. Li and J. Wu, Ceram. Int., 2012, 38, 443 CrossRef.
- W. J. Ong, L. L. Tan, S. P. Chai, S. T. Yong and A. R. Mohamed, ChemSusChem, 2014, 7, 690 CrossRef CAS PubMed.
- A. Hu, X. Zhang, K. D. Oakes, P. Peng, Y. N. Zhou and M. R. Servos, J. Hazard. Mater., 2011, 189, 278 CrossRef CAS PubMed.
- X. Chen and A. Selloni, Chem. Rev., 2014, 114, 9281 CrossRef CAS PubMed.
- K. Nakata and A. Fujishima, J. Photochem. Photobiol., C, 2012, 13, 169 CrossRef CAS.
- H. S. Kim, J. W. Lee, N. Yantara, P. P. Boix, S. A. Kulkarni, S. Mhaisalkar, M. Grätzel and N. G. Park, Nano Lett., 2013, 13, 2412 CrossRef CAS PubMed.
- Q. Xiang, J. Yu and M. Jaroniec, J. Am. Chem. Soc., 2012, 134, 6575 CrossRef CAS PubMed.
- K. Shankar, J. Basham, N. Allam, O. Varghese, G. Mor, X. Feng, M. Paulose, J. Seabold, K. Choi and C. Grimes, J. Phys. Chem. C, 2009, 113, 6327 CAS.
- D. R. Baker and P. V. Kamat, J. Phys. Chem. C, 2009, 113, 17967 CAS.
- S. Banerjee, S. K. Mohapatra and M. Misra, J. Phys. Chem. C, 2011, 115, 12643 CAS.
- X. Lu, G. Wang, T. Zhai, M. Yu, J. Gan, Y. Tong and Y. Li, Nano Lett., 2012, 12, 1690 CrossRef CAS PubMed.
- J. Pan, G. Liu, G. Q. Lu and H. M. Cheng, Angew. Chem., Int. Ed., 2011, 50, 2133 CrossRef CAS PubMed.
- H. Li, X. F. Li, C. Y. Zhang, S. L. Tie and S. Lan, Appl. Surf. Sci., 2017, 396, 221 CrossRef CAS.
- Y. C. Yao, X. R. Dai, X. Y. Hu, S. Z. Huang and Z. Jin, Appl. Surf. Sci., 2016, 387, 469 CrossRef CAS.
- Y. Q. Wang, W. H. Han, B. Zhao, L. L. Chen, F. Teng, X. D. Li, C. T. Gao, J. Y. Zhou and E. Q. Xie, Sol. Energy Mater. Sol. Cells, 2015, 140, 376 CrossRef CAS.
- L. F. Que, Z. Lan, W. X. Wu, J. H. Wu, J. M. Lin and M. L. Huang, J. Power Sources, 2014, 266, 440 CrossRef CAS.
- Y. Q. Wang, L. Gu, Y. G. Guo, H. Li, X. Q. He, S. Tsukimoto, Y. Ikuhara and L. J. Wan, J. Am. Chem. Soc., 2012, 134, 7874 CrossRef CAS PubMed.
- Q. Chen, G. H. Du, S. Zhang and L. M. Peng, Acta Crystallogr., Sect. B: Struct. Sci., 2002, 58, 587 CrossRef CAS.
- T. P. Feist and P. K. Davies, J. Solid State Chem., 1992, 101, 275 CrossRef CAS.
- S. Pavasupree, Y. Suzuki, S. Yoshikawa and R. Kawahata, J. Solid State Chem., 2005, 178, 3110 CrossRef CAS.
- R. Ma, Y. Bando and T. Sasaki, J. Phys. Chem. B, 2004, 108, 2115 CrossRef CAS.
- A. R. Armstrong, G. Armstrong, J. Canales and P. G. Bruce, Angew. Chem., Int. Ed., 2004, 43, 2286 CrossRef CAS PubMed.
- Z. Yuan and B. Su, Colloids Surf., A, 2004, 241, 173 CrossRef CAS.
- Y. Ren, Z. Liu, F. Pourpoint, A. R. Armstrong, C. P. Grey and P. G. Bruce, Angew. Chem., 2012, 124, 2206 CrossRef.
- M. Ye, J. Gong, Y. Lai, C. Lin and Z. Lin, J. Am. Chem. Soc., 2012, 134, 15720 CrossRef CAS PubMed.
- N. Serpone, D. Lawless and R. Khairutdinov, J. Phys. Chem., 1995, 99, 16646 CrossRef CAS.
- X. H. Wang, J. G. Li, H. Kamiyama, M. Katada, N. Ohashi, Y. Moriyoshi and T. Ishigaki, J. Am. Chem. Soc., 2005, 127, 10982 CrossRef CAS PubMed.
- P. V. Kamat, J. Phys. Chem. C, 2012, 116, 11849 CAS.
- W. Fan, Q. Lai, Q. Zhang and Y. Wang, J. Phys. Chem. C, 2011, 115, 10694 CAS.
- R. Liang, A. Hu, W. Li and Y. Zhou, J. Nanopart. Res., 2013, 15, 1990 CrossRef.
- A. Hu, R. Liang, X. Zhang, S. Kurdi, D. Luong, H. Huang, P. Peng, E. Marzbanrad, K. D. Oakes, Y. Zhou and M. R. Servos, J. Photochem. Photobiol., A, 2013, 256, 7 CrossRef CAS.
|
This journal is © The Royal Society of Chemistry 2017 |
Click here to see how this site uses Cookies. View our privacy policy here.