DOI:
10.1039/C7RA01479A
(Paper)
RSC Adv., 2017,
7, 15817-15822
A cancer cell-specific two-photon fluorescent probe for imaging hydrogen sulfide in living cells†
Received
5th February 2017
, Accepted 2nd March 2017
First published on 10th March 2017
Abstract
Hydrogen sulfide (H2S) could induce the proliferation of cancer cells in a concentration-dependent manner, and has close relation with the tumor growth. Monitoring the H2S level in real-time is of great important for understanding its roles in the cancer cell proliferation and the diagnosis of the tumor. Herein, a novel cancer cell-specific two-photon fluorescent probe BN-H2S for detecting H2S in cancer cells was designed and synthesized. Biotin was selected as the cancer cell-specific group and the azide group was employed as the response site for H2S. When BN-H2S responded to H2S, a turn-on fluorescence at 544 nm was observed clearly. BN-H2S exhibited high selectivity for H2S over other relative species. Under the guidance of the biotin group, BN-H2S can be successfully used for the two-photon imaging of H2S in cancer cells, while BN-H2S showed relatively weak response for H2S in normal cells. We expect that this design concept can be further developed for selectively detecting other biomolecules in living cancer cells.
1. Introduction
Hydrogen sulfide (H2S) is a crucial gasotransmitter besides nitric oxide and carbon monoxide.1,2 At normal levels, H2S plays important roles in many physiological processes, such as relaxation of vascular smooth muscles,3–5 inhibition of insulin signaling,6 mediation of neurotransmission,7–10 and suppress inflammation against oxidative stress.11,12 Recent studies indicate that H2S affects the proliferation of cancer cell in a concentration-dependent manner, and has closely relation with the cancer cell growth.13,14 For example, Cao et al. demonstrated that H2S could be endogenously produced in colon cancer cells (WiDr), and the exogenous H2S at physiologically relevant concentrations could reduce the cell viability.13 These studies suggest that H2S is a proliferative factor in human colon cancer cells, and can lead to an in-depth understanding of cancer development. Therefore, monitoring H2S level in real-time is of great important for the further understanding of its roles in the cancer cell proliferation, and also could provide novel H2S-based strategy for the cancer therapy.
Traditionally, the most widely applied methods for detecting H2S are methylene blue method,15 the electrode method16 and the monobromobimane method,17,18 but these methods need destructive sampling and have limitation for the real-time detection of H2S in living cells or tissues. Fluorescence imaging is a more attractive approach for detecting H2S because of its numerous advantages, such as imperial spatiotemporal resolution, non-destructive testing and real-time detection.19–21 So far, many fluorescent probes for H2S have been reported.22,23 However, to the best of our knowledge, these fluorescent probes all had no cancer cell-specific group and cannot distinguish cancer cells from normal cells. In addition, the two-photon imaging utilizes the long-wavelength light as excitation source, and generally has numerous advantages relative to one-photon imaging, such as reduced photodamage to biosamples and negligible background fluorescence.24,25 Therefore, the construction of cancer cell-specific two-photon fluorescent probe for imaging H2S in living system is still in demand.
In this work, we report a novel cancer cell-specific two-photon fluorescent probe (BN-H2S) for detecting H2S. In this probe, biotin was selected as the cancer cell-specific group, because the receptors related to the uptake of biotin are generally overexpressed on the cancer cell surface. Accordingly, BN-H2S could discriminate the cancer cells from normal cells under the guidance of biotin group. The probe BN-H2S has excellent sensitivity and selectivity for detecting H2S. The bioimaging experiments demonstrate that BN-H2S could be applied for the two-photon fluorescence imaging of H2S in living cancer cells.
2. Experimental
2.1 Materials and instruments
All chemical reagents were commercial. Twice-distilled water was used in all the experiments. Thin Layer Chromatography (TLC) analysis was performed on the silica gel plates and the chromatography was performed using silica gel (mesh 200–300, Qingdao Ocean Chemicals). NMR spectra were obtained on an AVANCE III 400 MHz Digital NMR Spectrometer, and tetramethylsilane (TMS) was used as internal reference. High-resolution electronspray mass spectra (HRMS) were obtained from a Bruker APEX IV-FTMS 7.0T mass spectrometer. pH values were determined on a Mettler-Toledo Delta 320 pH meter. UV-vis absorption spectra were measured on a Shimadzu UV-2600 spectrophotometer. Fluorescence spectra were obtained with a Hitachi F4600 fluorescence spectrophotometer. HeLa cells, NIH 3T3 cells and calf bovine serum were obtained from the College of Life Science, Nankai University (Tianjin, China).
2.2 Synthesis of compound 2
Compound 1 was synthesized according to the previous report.26 A mixture of compound 1 (636 mg, 2 mmol), biotin (488.62 mg, 2 mmol), EDCl (576 mg, 3 mmol), HOBt (675 g, 5 mmol) and DIEA (1 mL) in DMF (3 mL) was stirred for 12 h at room temperature. Then 6 mL water was added into the mixture and extracted with CH2Cl2, washed three times with water, dried over Na2SO4 and evaporated under reduced pressure to provide crude compound 2. Then the crude product was purified by silica column chromatography (CH2Cl2
:
MeOH = 10
:
1) to afford compound 2 (380 mg, yield 35%). 1H NMR (DMSO-d6, 400 MHz): δ 8.54–8.57 (m, 2H), 8.33–8.35 (d, J = 8.0 Hz, 1H), 8.22–8.24 (d, J = 8.0 Hz, 1H), 8.01–8.03 (t, 1H), 7.91–7.99 (t, 1H), 6.38–6.42 (d, J = 20.0 Hz, 2H), 4.29–4.32 (m, 1H) 4.11–4.14 (t, 2H), 4.04–4.07 (m, 1H), 2.94–2.99 (m, 1H), 2.78–2.82 (m, 1H), 2.56–2.59 (m, 1H), 1.91–1.94 (m, 2H), 1.55–1.56 (m, 1H), 1.38–1.40 (m, 3H), 1.19–1.21 (m, 3H). 13C NMR (DMSO-d6, 100 MHz): 172.77, 163.60, 163.55, 163.15, 132.96, 131.96, 131.82, 131.35, 130.29, 129.42, 129.29, 128.95, 123.48, 122.71, 61.43, 59.66, 55.81, 36.68, 35.69, 28.55, 28.44, 25.61. HRMS (ESI): m/z calculated for C24H25BrN4O5S [M + H]+ 545.0780, found: 545.0826.
2.3 Synthesis of BN-H2S
Compound 2 (272 mg, 0.5 mmol), NaN3 (65 mg, 1 mmol) were mixed in DMF (5.0 mL) and stirred at 50 °C for 4 h. Then 5 mL water was added into the mixture and extracted with CH2Cl2, washed three times with water, dried over Na2SO4 and evaporated under reduced pressure. The crude product was purified by silica column chromatography (CH2Cl2
:
MeOH = 5
:
1) to afford BN-H2S (147 mg, yield 58%) as an orange-yellow solid. 1H NMR (DMSO-d6, 400 MHz): δ 8.56–8.59 (m, 1H), 8.43–8.48 (m, 1H), 8.23–8.36 (m, 1H), 7.84–8.04 (m, 2H), 7.27–7.38 (m, 1H), 6.38–6.42 (d, J = 20.0 Hz, 2H), 4.29–4.32 (t, 1H), 4.11–4.14 (t, 2H), 4.05–4.06 (s, 1H), 2.97–2.99 (t, 1H), 2.78–2.82 (m, 1H), 1.91–1.94 (m, 2H), 1.48–1.53 (m, 1H), 1.35–1.39 (m, 3H), 1.18–1.24 (m, 4H), 0.82–0.87 (m, 1H). 13C NMR (DMSO-d6, 100 MHz): 172.65, 164.48, 163.58, 163.20, 153.10, 134.32, 131.38, 130.28, 129.72, 124.39, 122.41, 119.82, 108.53, 108.15, 61.40, 59.66, 55.81, 36.98, 35.72, 34.64, 28.53, 28.41, 25.62. HRMS (ESI): m/z calculated for C24H25N7O4S [M + H]+ 508.1761, found 508.1758.
2.4 Synthesis of BN-NH2
The mixture of BN-H2S (51 mg, 0.1 mmol) and Na2S (39 mg, 0.5 mmol) in 2 mL DMF was stirred to room temperature for 6 h. Then 5 mL water was added into the mixture and extracted with CH2Cl2, washed three times with water, dried over Na2SO4 and evaporated under reduced pressure. The solid residue was purified by flash chromatography column using methanol/dichloromethane (v/v 1
:
10) to afford a yellow solid as BN-NH2 (36 mg, yield 75%). 1H NMR (DMSO-d6, 400 MHz): δ 8.61–8.63 (d, 1H), 8.42–8.44 (d, 1H), 8.18–8.20 (d, 1H), 7.87–7.90 (t, 1H), 7.64–7.68 (t, 1H), 7.45 (s, 2H), 6.83–6.85 (d, J = 8.0 Hz, 1H), 6.38–6.43 (d, J = 20.0 Hz, 2H), 4.29–4.32 (t, 1H), 4.03–4.12 (m, 3H), 2.95–2.99 (m, 1H), 2.78–2.82 (m, 1H), 2.58 (s, 1H), 1.93–1.97 (t, 2H), 1.50–1.53 (m, 1H), 1.38–1.44 (m, 3H), 1.19–1.22 (m, 3H), 0.82–0.87 (m, 1H). 13C NMR (DMSO-d6, 100 MHz): 172.74, 163.93, 163.56, 163.17, 143.18, 132.98, 131.97, 131.37, 128.93, 127.77, 124.00, 122.84, 118.86, 116.40, 63.43, 59.65, 55.82, 36.73, 35.69, 30.23, 29.10, 23.46, 23.09. HRMS (ESI): m/z calculated for C24H27N5O4S [M + H]+ 482.1857, found 482.1861; [M + Na]+ 504.1681, found 504.1675.
2.5 Determination of fluorescence quantum yield
Fluorescence quantum yields were determined using Rhodamine-6G as reference (Φf = 0.94 in ethanol). The quantum yields were calculated following the equation: ΦS = ΦR × (IS/IR) × (AR/AS) × (ηS/ηR)2. Here, ΦS and ΦR are the quantum yields of sample and reference, IS and IR are the integrated emission intensities of the corrected spectra for the sample and reference, AR and AS stand for the absorbance of the reference and sample at the excitation wavelength, ηS/ηR are the values of refractive index for the respective solvent used for the sample and reference.
2.6 Measurements of two-photon cross section
Two-photon cross sections (δ) for BN-H2S in absence and presence of Na2S in PBS (20 mM, pH = 7.4, 5% MeOH) were determined following the previously reported methods, and Rhodamine 6G was selected as the reference.27 The two-photon absorption cross section (δ) was calculated using the equation: δ = δr(SsΦrϕrcr)/(SrΦsϕscs), where the subscripts s and r stand for the sample and reference molecule, S is the intensity of the signal collected using a CCD detector. Φ is the fluorescence quantum yield, and ϕ stands for the overall fluorescence collection efficiency of the experimental apparatus. c is the concentration, and δr is the two-photon absorption cross section of Rhodamine 6G.
2.7 Cytotoxicity experiments
Cytotoxic effects of BN-H2S were evaluated using the MTT assay. HeLa and NIH 3T3 cells were seeded into 96 well-plates at the density of 3000 cells per well. After 24 h of cell attachment, various concentrations of BN-H2S were added into wells for the further cultured of 24 h. Then, 10 μL of MTT (5 μg mL−1) were mixed into cells for incubated another 4 h. After that, 100 μL of DMSO were used to resolve the formazan. The plate was shaken for 20 min, and then the absorbance was determined at 570 nm by a microplate reader (Thermo Fisher Scientific). Cell viability was expressed as a percentage of the control culture value.
2.8 Cell culture and fluorescence imaging
HeLa and NIH 3T3 cells were cultured in modified Eagle's medium supplemented with 10% calf bovine serum in an atmosphere of 5% CO2 and 95% air at 37 °C. The cells were seeded into the glass-bottom culture dishes and cultured for 24 h. For the imaging of Na2S, the cells were incubated with 10 μM BN-H2S for 15 min at 37 °C, then the media was replaced with PBS. The cells were then incubated with Na2S for another 30 min, and then the imaging was performed using a Nikon A1R MP+ confocal microscope.
3. Results and discussion
3.1 Design and synthesis of BN-H2S
As shown in Scheme 1, the designed probe BN-H2S was made of three factors including cancer cell-specific group, two-photon fluorophore and H2S response site. Initially, biotin was selected as the cancer cell-specific group, because the receptors related to the uptake of biotin are generally overexpressed on the cancer cell surface to sustain the rapid growth of the cancer cells, and the biotin can be chemically modified easily. Previously, many studies also demonstrated that biotin could be used as a cancer-targeting molecule.28,29 Naphthalimide fluorophore was employed as the two-photon fluorophore due to its significant advantages in optical properties, including excellent photostability, high fluorescence quantum yield and desirable two-photon emission properties. In addition, the azide group was employed as the response site because of its highly selective and sensitive response for H2S. With these considerations in mind, we constructed a biotin-guided two-photon fluorescent probe BN-H2S for detecting H2S. NA-H2S has no biotin group was selected as the control compound, and was synthesized according to the previous report.30 The synthesis route of BN-H2S was shown in Scheme 1, and the structure was determined by HRMS, 1H NMR and 13C NMR spectra (ESI†).
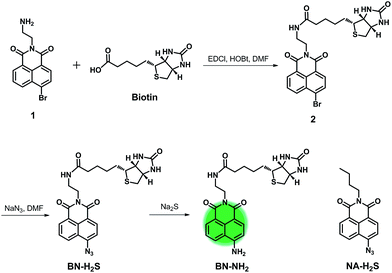 |
| Scheme 1 Synthesis route of BN-H2S and chemical structure of NA-H2S. | |
3.2 Optical response of BN-H2S to H2S
Initially, we determined the optical response of BN-H2S to H2S in PBS (20 mM, pH = 7.4, 5% MeOH) using UV-vis absorption spectra. Na2S was selected as H2S source. As shown in Fig. 1, BN-H2S exhibited a main absorption maximum at 366 nm with molar absorption coefficient (ε) of 0.77 × 104 M−1 cm−1 arised from the naphthalimide fluorophore. Upon the addition of Na2S, the absorbance at 366 nm decreased and a new absorption at 432 nm appeared, indicating that the reaction between BN-H2S and H2S indeed occurred.
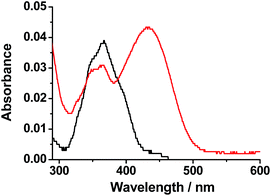 |
| Fig. 1 Absorption spectra of 5 μM BN-H2S in absence (black) and presence (red) of 100 μM Na2S in PBS (20 mM, pH = 7.4, 5% MeOH). | |
Subsequently, the optical response of BN-H2S to H2S in PBS was investigated using fluorescence spectra. As shown in Fig. 2, BN-H2S itself showed weak fluorescence under the excitation at 440 nm with the fluorescence quantum yield (Φ) of 0.01. Upon the addition Na2S, the fluorescence intensity at 544 nm increased gradually with raising the Na2S concentration, corresponding to the fluorescent color of BN-H2S solution changed from weak green to strong green after the addition of Na2S under the irradiation of 365 nm ultraviolet light. An excellent linearity between the fluorescence intensity at 544 nm and Na2S concentration in the range of 0–80 μM was observed, and the detection limit was calculated to 71 nM (S/N = 3) according to IUPAC recommendations. Therefore, BN-H2S has highly sensitivity and can potentially be applied for detecting H2S in living system.
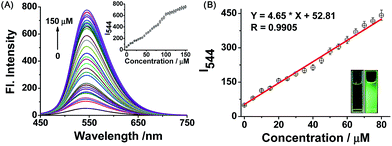 |
| Fig. 2 (A) Fluorescence spectra of 5 μM BN-H2S upon the addition of 0–150 μM Na2S in PBS (20 mM, pH = 7.4, 5% MeOH) with the excitation at 440 nm, inset: the fluorescence intensity at 544 nm as a function of Na2S concentration. (B) Linearity between the fluorescence intensity at 544 nm and Na2S concentration in the range of 0–80 μM, inset: images of 5 μM BN-H2S in absence (left) and presence (right) of 100 μM Na2S under a UV lamp at 365 nm. | |
The response mechanism of BN-H2S to H2S was further explored on the basis of the HRMS data and the reaction experiment of BN-H2S and Na2S. The azide group can be liable to be reduced to amino by H2S chemically, and the response mechanism of BN-H2S to H2S was proposed to be based on the reduction of nitrine (Scheme S1†). As shown in the HRMS assay (Fig. S1†), the two peaks at 482.1861 and 504.1671 corresponding to BN-NH2 (calcd [M + H]+, 482.1857 and [M + Na]+, 504.1681) were clearly observed, indicating that BN-H2S can be reduced to BN-NH2 in PBS. Meanwhile, BN-H2S can react with Na2S in DMF for 6 h, to afford the product BN-NH2 with a yield of 75% (Scheme 1). BN-NH2 displayed the main absorption at 432 nm and fluorescence at 544 nm (Φ = 0.29) under excitation at 440 nm in PBS, consisting well with the absorption and fluorescence spectra of BN-H2S after responding to Na2S (Fig. 3). Therefore, the response mechanism of BN-H2S to H2S was proposed to base on the reduction of azide group.
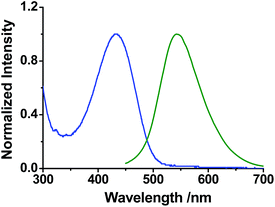 |
| Fig. 3 Normalized absorption (blue) and fluorescence spectra (green, λex = 440 nm) of 5 μM BN-NH2 in PBS (pH 7.4, 20 mM, 5% MeOH). | |
The selectivity of BN-H2S to H2S was evaluated by determining the fluorescence spectra of BN-H2S with various biologically relevant species in PBS (pH 7.4, 20 mM, 5% MeOH). We selected a serious of small biomolecules and ions that commonly exist in living systems as potential competitive analytes, such as Cys, GSH, SO32−, H2O2. After the addition of Na2S, the absorption at 432 nm appeared obviously, while the absorption spectra of BN-H2S had no marked change upon the addition of other relevant species (Fig. S2†). As shown in Fig. 4, only Na2S can trigger a significantly enhanced fluorescence at 544 nm, while the other relevant species showed no marked influence on the fluorescence spectra of BN-H2S. It indicates that BN-H2S has excellent selectivity for H2S and can be potentially used for detecting H2S in living system.
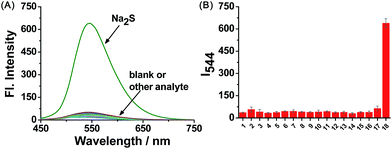 |
| Fig. 4 Fluorescence spectra (A) and fluorescence intensity at 544 nm (B) of 5 μM BN-H2S to various species in PBS (pH 7.4, 20 mM, 5% MeOH) under excitation at 440 nm. 1, blank; 2, GSH; 3, Cys; 4, Hcy; 5, FeCl2; 6, VC; 7, NaF; 8, NaBr; 9, NaI; 10, NaNO2; 11, H2O2; 12, NaClO; 13, ˙OH; 14, MgCl2; 15, ZnCl2; 16, NO; 17, Na2SO3; 18, Na2S. Concentration: Cys and GSH, 1 mM; the other species, 100 μM. | |
Time-dependent fluorescence spectra of BN-H2S in the presence of Na2S were studied. Upon the addition of 100 μM Na2S to BN-H2S solution, the fluorescence intensity at 544 nm increased gradually with time, and reached the maximum within 25 min (Fig. 5). It indicates that the probe BN-H2S could show response to H2S in a short time. In addition, BN-H2S displayed very weak fluorescence in the pH range of 4.0–10.0 (Fig. S3†). Upon the addition of Na2S, the enhanced fluorescence at 544 nm was observed clearly. However, the fluorescence response of BN-H2S to Na2S tended to occur at weak basic condition, probably because the H2S (pKa1 = 6.88) could be ionized to HS− at weak basic condition, which has stronger nucleophilicity relative to H2S and benefits for the reduction of azide group. Considering the physiological pH of 7.4 and the desirable response of BN-H2S to H2S at this pH, BN-H2S can be potentially used for detecting H2S in living system.
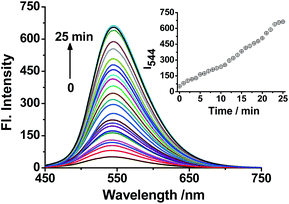 |
| Fig. 5 Time-dependent fluorescence spectra of 5 μM BN-H2S upon addition of 100 μM Na2S in PBS (20 mM, pH = 7.4, 5% MeOH) with the excitation at 440 nm, inset: fluorescence intensity at 544 nm as a function of time. | |
Furthermore, the two-photon properties of BN-H2S in absence and presence of Na2S were investigated by determining its two-photon action spectra (δΦ). As shown in Fig. 6, BN-H2S displayed nearly no two-photon property, while it displayed the maximum δΦ values of about 82 GM after the treatment of Na2S. It indicates that BN-H2S could be potentially served as a two-photon probe for determining H2S in living systems.
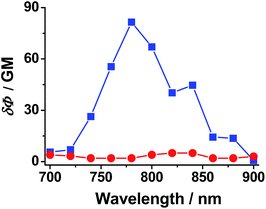 |
| Fig. 6 Two-photon action (δΦ) spectra of BN-H2S in absence (red) and presence (blue) of Na2S in PBS (20 mM, pH = 7.4, 5% MeOH). | |
3.3 Fluorescence imaging of BN-H2S in living cells
To confirm the cancer cell-specific capacity of BN-H2S and the two-photon fluorescence response of BN-H2S to H2S, the fluorescence imaging experiments were performed in cancer and normal cells, respectively. In consideration of the different expression quantities of biotin receptor on the cell surface, HeLa and NIH 3T3 cells were selected as cancer and normal cell models, respectively. MTT assays indicate that BN-H2S had no marked cytotoxicity for HeLa and NIH 3T3 cells at the concentration of 10 μM (Fig. S4†), and could be suitable for the cell imaging. HeLa and NIH 3T3 cells were incubated with 10 μM BN-H2S for 20 min, and then the cells were incubated with Na2S for another 15 min. As shown in Fig. 7, the HeLa cells treated with only BN-H2S showed nearly no fluorescence, and displayed strong green fluorescence after the further incubation with Na2S under the one-photon or two-photon excitation. However, when pretreated with 2 mM biotin and further incubated with BN-H2S and Na2S, HeLa cells showed relatively weak fluorescence under the one-photon or two-photon excitation, indicating the biotin can bond to the receptor and then adverse to the cell uptake of BN-H2S. Meanwhile, compared with HeLa cells, NIH 3T3 cells had weak green fluorescence when treated with BN-H2S and Na2S (Fig. 8). It indicates that the BN-H2S has higher affinity for the HeLa cells, likely because of the higher expression quantity of biotin receptor on the HeLa cell surface relative to NIH 3T3 cell surface. As the control experiments, the NA-H2S which has no biotin group, can be applied for the imaging of H2S in both HeLa and NIH 3T3 cells (Fig. S5†). Moreover, under two-photon excitation at 760 nm, the fluorescence peaks at about 540 nm were observed when BN-H2S responded H2S in PBS, HeLa cells and 3T3 cells, further confirming that the detectable fluorescence in cells can be ascribed to the response of BN-H2S to H2S (Fig. 9). To the best of our knowledge, the cancer cell-specific two-photon probe BN-H2S for monitoring H2S in living cells was reported for the first time. Taken together, the BN-H2S can be used as a cancer cell-specific two-photon probe for detecting H2S in living cells.
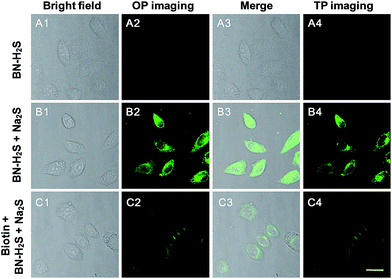 |
| Fig. 7 (A) Fluorescence images of HeLa cells treated with 10 μM BN-H2S; (B) fluorescence images of HeLa cells treated with 10 μM BN-H2S and 100 μM Na2S; (C) fluorescence images of HeLa cells pretreated 2 mM biotin and further treated with 10 μM BN-H2S and 100 μM Na2S. One-photon (OP) imaging: emission at 500–550 nm with excitation at 488 nm; two-photon (TP) imaging: emission at 500–550 nm with excitation at 760 nm. Scale bar = 20 μm. | |
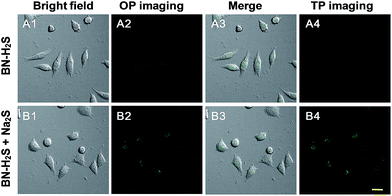 |
| Fig. 8 (A) Fluorescence images of NIH 3T3 cells treated with 10 μM BN-H2S. (B) Fluorescence images of NIH 3T3 cells treated with 10 μM BN-H2S and 100 μM Na2S. One-photon (OP) imaging: emission at 500–550 nm with excitation at 488 nm; two-photon (TP) imaging: emission at 500–550 nm with excitation at 760 nm. Scale bar = 20 μm. | |
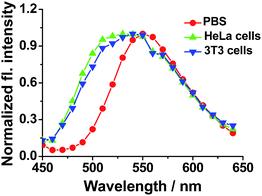 |
| Fig. 9 Normalized two-photon fluorescence spectra (λex = 760 nm) of 10 μM BN-H2S in presence of 100 μM Na2S in PBS (20 mM, pH = 7.4, 5% MeOH), HeLa cells and 3T3 cells, respectively. | |
4. Conclusions
In conclusion, we have designed a novel cancer cell-specific two-photon fluorescent probe BN-H2S for detecting H2S in cancer cells. In BN-H2S, biotin was selected as the cancer cell-specific group and the azide group was employed as the response site. When BN-H2S responded to H2S, the azide group was fast reduced to amide, and the turn-on green fluorescence was observed obviously. The probe exhibited excellent sensitivity with the detection limit of 71 nM, and high selectivity for H2S over the other relative species. Under the guidance of biotin group, BN-H2S can be successfully applied for the two-photon imaging of H2S in living cancer cells. We expect that this design concept could be further developed for the detection of other biomolecules in the living cancer cells.
Acknowledgements
This work was financially supported by NSFC (21472067, 21672083, 51602127), Taishan Scholar Foundation (TS 201511041), and the startup fund of the University of Jinan (309-10004).
References
- R. Wang, Antioxid. Redox Signaling, 2010, 12, 1061–1064 CrossRef CAS PubMed.
- K. Shimamoto and K. Hanaoka, Nitric Oxide, 2015, 46, 72–79 CrossRef CAS PubMed.
- G. D. Yang, L. Y. Wu, B. Jiang, W. Yang, J. S. Qi, K. Cao, Q. Meng, A. K. Mustafa, W. Mu, S. Zhang, S. H. Snyder and R. Wang, Science, 2008, 322, 587–590 CrossRef CAS PubMed.
- B. D. Paul and S. H. Snyder, Nat. Rev. Mol. Cell Biol., 2012, 13, 499–507 CrossRef CAS PubMed.
- J. W. Elrod, J. W. Calvert, J. Morrison, J. E. Doeller, D. W. Kraus, L. Tao, X. Jiao, R. Scalia, L. Kiss, C. Szabo, H. Kimura, C. W. Chow and D. J. Lefer, Proc. Natl. Acad. Sci. U. S. A., 2007, 104, 15560–15565 CrossRef CAS PubMed.
- Y. Kaneko, Y. Kimura, H. Kimura and I. Niki, Diabetes, 2006, 55, 1391–1397 CrossRef CAS PubMed.
- K. Eto, T. Asada, K. Arima, T. Makifuchi and H. Kimura, Biochem. Biophys. Res. Commun., 2002, 293, 1485–1488 CrossRef CAS PubMed.
- K. Abe and H. Kimura, J. Neurosci., 1996, 16, 1066–1071 CAS.
- H. Kimura, Neurochem. Int., 2013, 63, 492–497 CrossRef CAS PubMed.
- K. Fukami, F. Sekiguchi, M. Yasukawa, E. Asano, R. Kasamatsu and M. Ueda, Biochem. Pharmacol., 2015, 97, 300–309 CrossRef CAS PubMed.
- L. Li, M. Bhatia, Y. Z. Zhu, Y. C. Zhu, R. D. Ramnath, Z. J. Wang, F. B. Mohammed Anuar, M. Whiteman, M. Salto-Tellez and P. K. Moore, FASEB J., 2005, 19, 1196–1198 CAS.
- M. L. Lo Faro, B. Fox, J. L. Whatmore, P. G. Winyard and M. Whiteman, Nitric Oxide, 2014, 41, 38–47 CrossRef CAS PubMed.
- Q. Cao, L. Zhang, G. Yang, C. Xu and R. Wang, Antioxid. Redox Signaling, 2010, 12, 1101–1109 CrossRef CAS PubMed.
- W. J. Cai, M. J. Wang, L. H. Ju, C. Wang and Y. C. Zhu, Cell Biol. Int., 2010, 34, 565–572 CrossRef CAS PubMed.
- V. Kuban, P. K. Dasgupta and J. N. Marx, Anal. Chem., 1992, 64, 36–43 CrossRef CAS PubMed.
- D. M. Tsai, A. S. Kumar and J. M. Zen, Anal. Chim. Acta, 2006, 556, 145–150 CrossRef CAS PubMed.
- C. M. Klingerman, N. Trushin, B. Prokopczyk and P. Haouzi, Am. J. Physiol., 2013, 305, 630–638 Search PubMed.
- M. Nishida, T. Sawa, N. Kitajima, K. Ono, H. Inoue, H. Ihara, H. Motohashi, M. Yamamoto, M. Suematsu, H. Kurose, A. Vliet, B. Freeman, T. Shibata, K. Uchida, Y. Kumagai and T. Akaike, Nat. Chem. Biol., 2012, 8, 714–724 CrossRef CAS PubMed.
- J. R. Lakowicz, Principles of Fluorescence Spectroscopy, Springer, New York, 3rd edn, 2006 Search PubMed.
- Y. Kushida, T. Nagano and K. Hanaoka, Analyst, 2015, 140, 685–695 RSC.
- B. Dong, X. Song, X. Kong, C. Wang, Y. Tang, Y. Liu and W. Lin, Adv. Mater., 2016, 28, 8755–8759 CrossRef CAS PubMed.
- V. S. Lin, W. Chen, M. Xian and C. J. Chang, Chem. Soc. Rev., 2015, 44, 4596–4618 RSC.
- X. Li, X. Gao, W. Shi and H. Ma, Chem. Rev., 2014, 114, 590–659 CrossRef CAS PubMed.
- L. Qian, L. Liand and S. Q. Yao, Acc. Chem. Res., 2016, 49, 626–634 CrossRef CAS PubMed.
- K. Zheng, W. Lin, L. Tan, H. Chen and H. Cui, Chem. Sci., 2014, 5, 3439–3448 RSC.
- X. Zhou, F. Su, H. Lu, P. Senechal-Willis, Y. Tian, R. H. Johnson and D. R. Meldrum, Biomaterials, 2012, 33, 171–180 CrossRef CAS PubMed.
- N. S. Makarov, M. Drobizhev and A. Rebane, Opt. Express, 2008, 6, 4029–4047 CrossRef.
- S. Chen, X. Zhao, J. Chen, J. Chen, L. Kuznetsova, S. S. Wong and I. Ojima, Bioconjugate Chem., 2010, 12, 979–987 CrossRef PubMed.
- Y. H. Lee, Y. Tang, P. Verwilst, W. Lin and J. S. Kim, Chem. Commun., 2016, 52, 11247–11250 RSC.
- L. Zhang, S. Li, M. Hong, Y. Xu, S. Wang, Y. Liu, Y. Qian and J. Zhao, Org. Biomol. Chem., 2014, 12, 5115–5125 CAS.
Footnote |
† Electronic supplementary information (ESI) available: Supplementary spectra, NMR and HRMS data. See DOI: 10.1039/c7ra01479a |
|
This journal is © The Royal Society of Chemistry 2017 |
Click here to see how this site uses Cookies. View our privacy policy here.