DOI:
10.1039/C7RA01472D
(Paper)
RSC Adv., 2017,
7, 31875-31885
Characteristics of soy protein isolate/gum arabic-stabilized oil-in-water emulsions: influence of different preparation routes and pH
Received
5th February 2017
, Accepted 13th June 2017
First published on 21st June 2017
Abstract
The properties of oil-in-water emulsions containing soy protein isolate (SPI) with or without gum arabic (GA) have been studied under different pH and different preparation routes. Three routes were used for the preparation of emulsions (10% (w/v) oil and 0.5% (w/v) SPI with or without 0.5% (w/v) GA). In emulsions I, SPI solution with/without GA were first pH adjusted followed by homogenization with oil; in emulsions II, the oil was directly emulsified with SPI–GA coacervates at pH 4.0 to form the ‘mixed emulsions’, then pH was adjusted; in emulsions III, GA was added to a SPI-stabilized emulsion followed by pH adjustment. The results showed that pH adjustment before or after homogenization with oil greatly influenced the droplet size and emulsifying stability whether for the SPI emulsions or SPI/GA emulsions. The emulsions III showed much slower flocculation rate and higher emulsifying stability at pH 4.0–7.0. The microstructures of SPI–GA emulsion III were more uniform, with the comparably smallest d43 values in the presence of SDS. It could be indicated that the preparation routes of emulsions greatly influenced the stability of SPI–control emulsion or SPI–GA emulsion.
1. Introduction
Emulsions are widely used in cosmetics, pharmaceuticals, and food industries because lipophilic bioactive compounds can be encapsulated in oil droplets to increase their solubility and stability in the aqueous phase.1 Milk protein and soy protein are preferably used as emulsifiers in the food industry.2 Soy protein is an abundant byproduct of the soybean oil industry and has good functionalities for food processing.3 The protein adsorption layers in the oil–water interface prevent the drop–drop coalescence and then stabilize the emulsions.4 However, protein-stabilized emulsions are highly sensitive to environmental stresses such as pH, ionic strength and temperature.5–7 When the pH approaches the isoelectric point of the protein and/or salt concentration is higher in the emulsion, the electrostatic repulsion of the protein adsorption layers decreases and therefore coalescence and flocculation happen.8
The emulsion stability depends on the factors such as emulsifier surface coverage and surface charge density, as well as emulsifier layer thickness and bulk physicochemical conditions. It is reported that the stability of protein emulsion can be improved by protein–polysaccharide complex formed by electrostatic attraction.9–11 The polysaccharide can change the charges as well as increase the thickness of the interfacial layers, enhancing the hydrophilicity and steric repulsion of the droplets. For example, the addition of negatively charged polysaccharides interacting with positively charged proteins may form a thick layer at the interface which prevents coalescence. The behavior of charged polysaccharides added to protein-stabilized oil-in-water emulsions depends on the pH. For example, at a pH below the isoelectric point of the protein the negatively charged pectin interacts via electrostatic interactions with β-lactoglobulin absorbed at the interface. McClements and Li (2010) have found that the emulsion stability can be improved by adding polysaccharide, forming an interfacial complex with the adsorbed protein layer after homogenization, i.e., forming “bilayer” or “layer-by-layer” coated droplets.1 The emulsions containing multilayer-coated droplets are more stable than those containing bilayer-coated droplets.5 Soy soluble polysaccharides are shown to prevent destabilization of SPI-based oil-in-water (O/W) emulsions under acidic conditions.12
A number of different preparative routes have been explored to form stabilized oil-in-water emulsions and ‘order of addition’ effects have been shown to be important.13 Emulsions stabilized by electrostatic polysaccharide–protein complexes can be formed in different ways. Traditionally, emulsions have been prepared using premixed coacervates. Weinbreck, Minor and Kruif (2004), for example, used whey protein–gum arabic complex coacervates to encapsulate flavor oils.14 They reported that the best capsules are formed at the pH for maximum coacervation and maximum viscosity. Another approach is to form an emulsion using a protein as a primary emulsifier and then to add a polysaccharide to adsorb onto the protein layer forming a bilayer. The increase in adsorbed polymer layer thickness and likely increase in zeta potential will tend to stabilize the emulsion by inhibiting droplet aggregation through enhanced electrosteric repulsions. Emulsions produced using this procedure have been shown to have enhanced stability in respect of ionic strength and pH.15
In the present study, two biopolymers (soy protein isolates, SPI and gum arabic, GA) have been chosen to stabilize oil-in-water emulsions. SPI is mainly composed of β-conglycinin (7S) and glycinin (11S). The former is a glycoprotein composed of three subunits, α, α′, and β, whereas the latter is a hexamer of acidic and basic subunit pairs connected by one disulfide bond.16 Both glycinin and β-conglycinin have emulsifying ability and are capable of stabilizing emulsions by lowering the interfacial tension between water and oil.17 GA is widely utilized in food production as a soluble dietary fiber. GA is an arabinogalactan-type polysaccharide, which is composed of six carbohydrate moieties and a protein fraction and can be considered as a weak polyelectrolyte.18 The major fraction of GA consists of β-(1-3) galactopyranose polysaccharide backbone that is highly branched with β-(1-6) galactopyranose residues terminating in arabinose and glucuronic acid and/or 4-O-methyl glucuronic acid units.19 GA has a pKa value of about 3 due to its ionized carboxylic groups along the backbone. GA is negatively charged in mildly acidic solution. Previously, we characterized the complexing behavior between SPI and GA by isothermal titration calorimetry (ITC), turbidity, sedimentation and ternary phase boundaries.20 However, knowledge of emulsifying properties of soy proteins/gum arabic is far away from its application at acidic environment.
The understanding of protein–polysaccharide interactions is fundamental in predicting the stability and rheology of emulsions. The objective of this research was to investigate the influence of different preparation routes, pH and addition of GA on the stability of SPI-stabilized emulsions. And the mean droplet diameter, microstructure, droplet surface coverage, ζ-potential and rheological properties were evaluated.
2. Materials and methods
2.1 Materials
Defatted low-denatured soybean meal (protein content, 52.4% (w/w), dry basis) was provided by Shandong Wonderful Co., Ltd. (Dongying, China). Gum arabic (crude protein: 2.19%, moisture: 10.72%, ash: 3.32%, w/w) was purchased from Sinopharm Chemical Reagent Co., Ltd. (Shanghai, China). Nile Red and Fluorescein isothiocyanate (FITC) were purchased from Sigma-Aldrich (St. Louis, Mo). Soybean oil was obtained from a local supermarket. Other reagents and chemicals used in this study were of analytical grade. Deionized water was used for the preparation and dilution of the solutions.
2.2 Preparation of SPI
To prepare soy protein with high solubility, the method of Li et al. (2007) was used.21 Briefly, defatted soybean flake, which had been prewashed with aqueous alcohol, was suspended in deionized water and adjusted to pH 7.0 with 2.0 M NaOH. After stirring for 1 h, the suspension was centrifuged at 8000g for 30 min at 4 °C. Then, the pH of the supernatant was adjusted to 4.5 with 2.0 M HCl. The protein precipitate collected by centrifugation at 8000g for 30 min at 4 °C was redissolved with deionized water and adjusted to pH 7.0 with 2.0 M NaOH. Protein solution was then dialyzed and freeze dried. Proximate analysis showed that the dried powder had protein contents of 92.47% (N × 6.25) and ash contents of 3.01% on a dry basis.
2.3 Preparation of SPI and GA stock solutions
SPI and GA stock solutions were prepared by dispersing a certain amount of biopolymer powder in distilled water under gentle stirring at room temperature (25 ± 1 °C) for 2 h and left overnight at 4 °C to allow complete hydration of macromolecules. SPI (7.0%, w/w) dispersion was centrifuged at 10
000g for 30 min at 4 °C and the protein content of the supernatants was determined by Lowry's method.22 NaN3 (0.02%, w/v) was added to inhibit bacteria growth.
2.4 Protein solubility
SPI solutions diluted to 0.5% (w/w) were adjusted to pH 3.0–7.0 with 1 M HCl and stirred for 30 min. The suspension was centrifuged at 10
000g for 30 min at 20 °C. Protein content in the supernatant determined using Lowry method. Protein solubility was the percentage of the original protein that was solubilized. All experiments were carried out at least three times.
2.5 Turbidity assay
SPI and GA solutions were diluted and well mixed with a total biopolymer concentration of 0.1% (w/w). Control SPI solution was also assayed at a concentration of 0.05% (w/w). The turbidity was changed by adding 0.01–2.0 M HCl (pH range of 7.0–2.0). Turbidity titration curves were measured with a UV-2450 spectrophotometer (Shimadzu, Kyoto, Japan) at 600 nm using plastic cuvettes (1 cm path length) according to Dong et al. (2013).20
2.6 Zeta potential measurement
SPI solution was diluted to 1.0% (w/w) and mixed with GA solution (1.0%, w/w) in equal volume. The zeta potential samples (SPI, GA and mixtures) were prepared by adjusting to desired pH and then diluted to 0.1% (w/w) with the same pH value. Zeta potential measurement was performed on Zetasizer Nano-ZS instrument (Malvern Instruments, British) at 25 °C. The equilibration time for each sample was 90 s.
2.7 Emulsion preparation
Aqueous biopolymer solutions and 10% (w/v) soybean oil were initially emulsified using a high-shear homogenizer (FA25 model, Fluko Equipment Co., Ltd., Shanghai, China) at 10
000 rpm for 1 min, and then homogenized at 40 MPa with three passes using a high-pressure homogenizer (AH 2010, ATS Engineering, Inc., Shanghai, China). As shown in Fig. 1, emulsions were prepared in three different ways. (I) SPI and GA solutions were well mixed. The mixed solutions were adjusted to pH 3.0–7.0 with 1.0 M HCl. Then the mixtures were stirred for 1 h before emulsification. (II) SPI and GA solutions were well mixed. Then pH was adjusted to 4.0 with 1.0 M HCl to form complex. SPI–GA emulsion was prepared by homogenizing the complex with soy oil. The emulsion was divided into five small aliquots and then adjusted pH to 3.0–7.0 with 1.0 M HCl, respectively. (III) GA solutions was slowly added to SPI-stabilized emulsion (1.0% SPI, 20% soybean oil, w/v). The resultant emulsion was divided into five small aliquots and then adjusted pH to 3.0–7.0 with 1.0 M HCl, respectively. SPI-stabilized emulsions as controls were also separately prepared in the above different ways. All the final emulsions contained 10% (w/v) oil, 0.5% (w/v) SPI with or without 0.5% (w/v) GA.
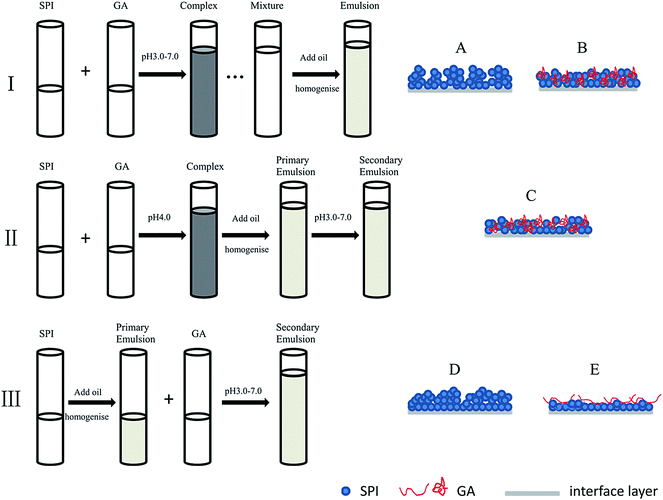 |
| Fig. 1 Schematic representation of different preparation routes (I, II, III) for emulsions. (A–E) were the situation of interface absorption at acidic pHs. (A) Soy proteins aggregates absorbed on the interface for SPI–control emulsion I; (B) soy proteins and GA complexes absorbed on the interface for SPI–GA emulsion I; (C) soy proteins and GA complexes on the interface for SPI–GA emulsion II (similar to B); (D) protein monolayer absorbed on the surface at pH 7.0, then with the decrease of pH, protein dissolved in water deposited gradually on the monolayer protein for SPI–control emulsion III; (E) protein monolayer absorbed on the surface at pH 7.0, then with the decrease of pH, soy proteins–GA mixtures deposited gradually for SPI–GA emulsion III. | |
2.8 Particle size measurement
The particle size measurement of the emulsion sample was carried out on a Mastersizer 2000 Laser Particle Size Analyzer (Malvern Instruments, Malvern, UK). The parameters of refractive index were set to 1.470 for soybean oil and 1.330 for deionized water. The emulsions were diluted ten times using deionized water or 1.0% (w/v) sodium dodecyl sulfate (SDS) solution before determinations. The particle size was reported as the volume mean diameter (d43).
2.9 Flocculation index (FI) measurement
The calculation of flocculation was used for quantitative evaluation of flocculation degree of different samples. Flocculation index was calculated as follows: |
FI (%) = [(d43 in water/d43 in SDS) − 1.0] × 100
| (1) |
where d43 in water and d43 in SDS were the volume mean diameter determined in water and in 1% SDS, respectively.
2.10 Determination of percentage of adsorbed proteins and polysaccharides
Percentage of adsorbed proteins and polysaccharides were determined according to the method described by Tangsuphoom and Coupland (2009) with slight modification.23 Emulsions were centrifuged at 18
000g for 60 min at room temperature inducing the separation of a cream layer at the top and an aqueous phase at the bottom of the tube. The aqueous phase was carefully withdrawn using a syringe and passed through a 0.22 μm filter and protein and polysaccharide concentrations were determined by Lowry method and phenol/sulfuric acid method, respectively.22,24
The percentage of adsorbed protein and polysaccharide was calculated as follows:
|
AP (%) = (Ci − Cs)/Ci × 100
| (3) |
where AP was adsorbed protein (or polysaccharide),
Ci was the initial protein (or polysaccharide) concentration of samples used for the emulsion preparation,
Cs was the protein (or polysaccharide) concentration of the aqueous phase after centrifugation.
2.11 Rheological measurement
Rheological measurement according to Peng, Hua, Chen, Kong, and Zhang (2016) was performed on the controlled-stress rheometer AR1000 (TA Instrument, New Castle, UK) with a parallel plate geometry (50 mm diameter and 1 mm gap) at 25 °C.25 The viscosity was determined as the function of shear rates (ranging from 0 to 100 s−1) and fitted to a power law model as follows:where η was the viscosity (Pa s), γ was the shear rate (s−1), k was the consistency index (Pa sn), n was the index of flow behavior: n < 1 for shear-thinning fluid, n = 1 for Newtonian fluid and n > 1 for shear-thickening fluid.
2.12 Confocal laser scanning microscopy (CLSM) measurement
Fresh emulsion was mixed with FITC-Nile Red mixture solution (40 μl of 0.1% (w/w) FITC and 0.1% (w/w) Nile Red per ml emulsion), and the stained mixture was stirred for 30 min in the dark. The samples were examined using confocal laser scanning microscope (LSM 710) with a ×20 objective. The images were collected using 488 and 543 nm excitation wavelength for FITC and Nile Red, respectively.
2.13 Creaming index (CI) measurement
3.0 ml of fresh emulsion was added into sample bottle and stored at room temperature. The total height of emulsion (Ht) was 3.5 cm. The height of aqueous layer at the bottom (Ha) was recorded until the fourteenth day. Creaming index was calculated as follows: |
CI (%) = (Ha/Ht) × 100
| (5) |
2.14 Data analysis
All experiments were performed in triplicate. The data were analyzed using SPSS for Windows (version 13.0, SPSS Inc. Chicago, IL) for one-way ANOVA. Data were expressed as mean values ± standard deviations. Significant differences were determined by comparing the means and to identify P < 0.05.
3. Results and discussion
3.1 Turbidity analysis
Changes to turbidity as a function of pH for SPI or SPI–GA mixed solutions were given in Fig. 2. On addition of HCl with different concentrations to the SPI solution (pH 7.0), it was observed that the curve showed a rapid rise in turbidity near pH approximately 5.5 and kept the maximum turbidity of about 0.4 at pH 4.0–5.0. Turbidity is a function of particle shape, refractive index, size distribution and specific gravity. Here the observed rapid rise in turbidity was thought to be associated with formation of protein aggregates as a result of reduced electrostatic repulsive forces between individual proteins, which could also be seen from the low nitrogen solubility (8.07% for pH 4.0 and 4.36% for pH 5.0).
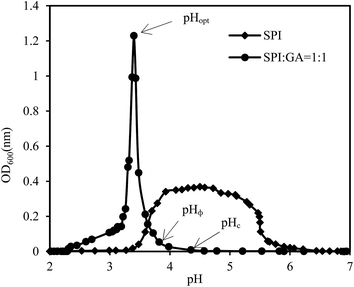 |
| Fig. 2 Turbidity curve as a function of pH for SPI–GA. | |
pH affected the charge densities and thus the complexing behavior of SPI and GA, as shown in Fig. 2. Acidification of SPI–GA mixture from neutral pH first led to the formation of soluble complexes at pH 4.35 (pHc). Above pHc, electrostatic repulsive forces inhibited the formation of complexes, and the turbidity remained at the baseline. With further acidification to pH 3.82 (pHΦ), an abrupt increase in OD600 was observed, indicating the formation of insoluble complexes. The turbidity reached the maximum at pH 3.43 (pHopt) and decreased rapidly as pH continued to drop. It could be seen that the addition of GA could keep SPI–GA mixture remain at very low turbidity when pH is higher than 4.0, which might be helpful to the resultant emulsions. Liu, Elmer, Low, and Nickerson (2010) studied β-lactoglobulin and GA complex coacervation and reported that a maximum interaction between the two biopolymers occurred at pH 4.2 for the 1
:
1 mixture.26
3.2 Surface charge analysis
SPI dispersions showed gradually less negative values with decreasing pH as shown in Fig. 3, eventually becoming positive below the pI of SPI. With the addition of GA, at pH 6.0–7.0, there was little interaction between SPI and GA due to the both negative charges as evidenced by −20 to −22 mV ζ potential values. At pH 4.0 and 5.0, the dispersion yielded negative ζ potential due to electrostatic complexation between SPI and GA.12 This was particularly evident at pH 4.0 and 5.0, where SPI would be expected to precipitate sans added GA. When pH was decreased to 3.0, SPI–GA dispersion showed positive ζ potential (about 10 mV) due to the increased charges of SPI.
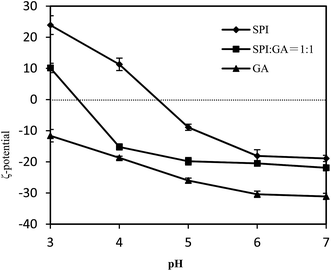 |
| Fig. 3 ζ-Potential values as a function of pH for SPI–GA. | |
3.3 Fresh emulsion characteristics: influence of pH and order of addition to oil–water interface
Three routes were investigated for the preparation of emulsions (10% (w/v) oil, 0.5% (w/v) SPI with/without 0.5% (w/v) GA). In emulsions I, SPI solution with/without GA were first adjusted pH followed by homogenization with oil; in emulsions II, the oil was directly emulsified with mixed SPI–GA solution at pH 4.0 to form the ‘mixed emulsions’, then pH was adjusted to prepare the different emulsions; in emulsions III, GA was added to a SPI-stabilized emulsion followed by pH adjustment. The schematic representation of the three preparation methods was shown in Fig. 1.
As summarized in Table 1, a key finding was that pH adjustment before or after homogenization with oil greatly influenced the droplet size and stability of the emulsions whether for the SPI emulsion or SPI–GA emulsion. It indicated that the order of pH adjustment resulted in different conformations of soy proteins and the adsorption morphology on the interfacial layers.27 The addition of GA could partly improve the emulsion (emulsion III), with the smallest d43 values in the presence of SDS.
Table 1 Mean particle size values (d43, in water or 1% SDS), flocculation index (FI) and percentage of absorbed protein (AP1) and polysaccharides (AP2) in fresh emulsions prepared in different waysa
Emulsion type |
pH |
d43 (μm) |
FI (%) |
AP1 (%) |
AP2 (%) |
Water |
1% SDS |
Means within a column with different letters are significantly different (P < 0.05). |
I |
SPI |
3 |
33.53 ± 0.45h |
1.21 ± 0.05c |
26.71 ± 0.64ij |
68.61 ± 0.99j |
— |
4 |
124.40 ± 1.51a |
2.54 ± 0.86a |
47.97 ± 0.28f |
94.98 ± 1.00de |
5 |
119.90 ± 1.43b |
1.23 ± 0.01b |
96.47 ± 0.93b |
95.80 ± 1.00cd |
6 |
17.59 ± 0.23n |
1.13 ± 0.02d |
14.56 ± 0.25l |
61.42 ± 0.99kl |
7 |
1.08 ± 0.23r |
0.92 ± 0.01h |
0.17 ± 0.02s |
60.82 ± 0.99l |
SPI/GA |
3 |
45.89 ± 0.17f |
1.05 ± 0.01e |
42.57 ± 0.10g |
96.32 ± 0.02c |
95.93 ± 0.04b |
4 |
95.34 ± 9.03d |
1.22 ± 0.03c |
77.14 ± 5.58de |
98.17 ± 0.36b |
69.47 ± 2.19c |
5 |
131.90 ± 6.12a |
1.28 ± 0.07bc |
102.05 ± 8.99ab |
96.06 ± 0.11d |
66.96 ± 0.13d |
6 |
39.16 ± 0.32g |
1.01 ± 0.01f |
37.78 ± 0.32h |
90.76 ± 0.04f |
5.40 ± 0.09j |
7 |
1.34 ± 0.01q |
0.97 ± 0.01g |
0.38 ± 0.01q |
69.57 ± 0.18j |
3.89 ± 0.09k |
II |
SPI/GA |
3 |
89.17 ± 1.45d |
1.06 ± 0.01e |
82.12 ± 0.89d |
97.63 ± 0.18b |
97.27 ± 0.31a |
4 |
92.80 ± 2.27d |
1.07 ± 0.01e |
85.73 ± 1.79c |
98.17 ± 0.36b |
69.47 ± 2.19c |
5 |
112.7 ± 0.75c |
1.07 ± 0.03e |
104.32 ± 0.42a |
99.08 ± 0.18a |
67.50 ± 2.01cd |
6 |
91.88 ± 2.06d |
1.07 ± 0.03e |
84.87 ± 0.89c |
95.99 ± 0.36c |
32.49 ± 0.14f |
7 |
83.00 ± 1.75e |
1.06 ± 0.07defg |
77.30 ± 0.92e |
95.26 ± 0.36d |
32.05 ± 0.12g |
III |
SPI |
3 |
18.36 ± 0.01m |
1.46 ± 0.12b |
11.55 ± 1.07m |
75.99 ± 0.38i |
— |
4 |
18.93 ± 0.25l |
2.45 ± 0.09a |
6.74 ± 0.39n |
98.69 ± 0.19b |
5 |
29.65 ± 0.96i |
2.54 ± 0.23a |
10.66 ± 0.69m |
88.56 ± 0.56g |
6 |
6.39 ± 0.01o |
0.99 ± 0.01g |
5.44 ± 0.01o |
60.98 ± 1.88kl |
7 |
1.07 ± 0.01r |
0.85 ± 0.01i |
0.25 ± 0.01r |
62.85 ± 0.75k |
SPI/GA |
3 |
20.88 ± 0.18k |
0.77 ± 0.01j |
26.19 ± 0.27j |
95.48 ± 0.03e |
56.58 ± 0.11e |
4 |
19.24 ± 0.51l |
0.84 ± 0.01i |
21.96 ± 0.81k |
96.05 ± 0.08d |
14.20 ± 0.30h |
5 |
23.80 ± 0.66j |
0.83 ± 0.01i |
27.54 ± 0.90i |
96.10 ± 0.22cd |
14.85 ± 0.04i |
6 |
2.92 ± 0.16p |
0.64 ± 0.01k |
3.60 ± 0.27p |
95.91 ± 0.12d |
0.06 ± 0.01l |
7 |
0.67 ± 0.01s |
0.63 ± 0.01k |
0.05 ± 0.01t |
79.12 ± 0.53h |
0.05 ± 0.01l |
3.3.1 Droplet size (in the absence or presence of SDS). Significant differences in the droplet size of SPI or SPI–GA emulsion were evident with the change of pH from 7.0 to 3.0 no matter which preparation route was applied. Table 1 shows the d43 values of droplets in different emulsions prepared at different conditions, at varying pH (3.0–7.0), determined using water or 1% SDS as the dispersing solvent. The d43 of deflocculated droplets in the emulsions, e.g., in the presence of SDS, can reflect ability of the proteins to help dispersion of oil phase into an aqueous medium. As shown in Table 1 and Fig. 4, the particle size distributions were dominated by a single peak at about 1 μm (d43 in 1% SDS) except SPI–GA emulsion I at pH 4.
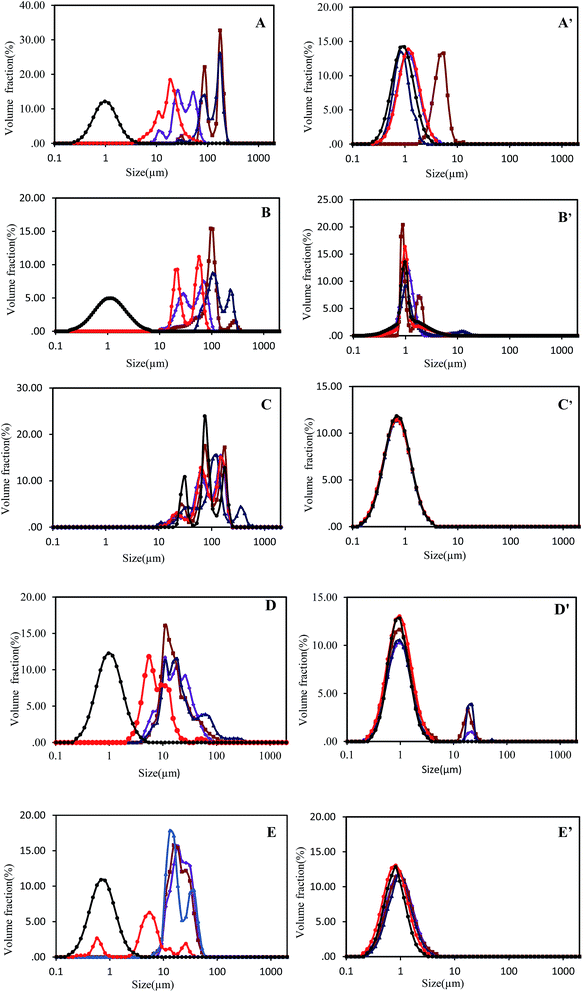 |
| Fig. 4 pH-dependent (pH 3 , pH 4 , pH 5 , pH 6 , pH 7 ) particle size distributions of SPI and SPI/GA emulsions prepared by method I (A, A′ for SPI emulsions and B, B′ for SPI/GA emulsions), method II (C, C′ for SPI/GA emulsions) and method III (D, D′ for SPI emulsions and E, E′ for SPI/GA emulsions), as determined with water (A–E) or 1% SDS (A′–E′). | |
For SPI–control emulsions, the particle size values differ significantly with the emulsifying routes and pH. At neutral pH, the particle size (d43) and FI for the emulsions prepared by different routes were similar, while at acidic pH they are quite different for the emulsions prepared by different routes. Due to the low solubility of soy proteins at pH 4.0–5.0, the SPI–control emulsion I owned bigger particle size about 120 μm. Combined with d43 values in the presence of SDS, it could be seen that the droplets were flocculated severely especially at pH 5.0 with FI of 96.47%. The flocculation of the droplets might be due to the hydrophobic interactions among soy proteins.28 For emulsion I or III at pH 3.0 and pH 6.0–7.0, the charged SPI prevented flocculation via electrostatic repulsion. Droplet sizes were smallest (ca. 1.07–1.08 μm) at pH 7.0 further away from the pI of SPI. At pH 6.0 and 3.0, average droplet sizes were ca. 6.39–33.53 μm due to reduction of like-charge repulsion between droplets. It was interestingly found that SPI–control emulsion III had smaller droplet size and FI, which were significantly different from SPI–control emulsion I. This indicated that the preparation route greatly influenced the obtained emulsions.
Both SPI–control emulsions III and SPI–GA emulsions III owned smaller average droplet size ranged from 0.67 to 29.65 μm at pH 3.0–7.0. This suggested that SPI emulsions obtained by homogenizing at neutral pH were more resistant to pH variations compared with those (emulsions I) adjusting pH followed by homogenization, which could be reflected from the obviously different particle size, especially at pH 4.0 and 5.0. The result was similar with the work from Azarikia and Abbasi (2016), who investigated the effects of different preparation methods on whey protein-tragacanth emulsions and found that “layer by layer” emulsions had lower particle size compared with “mixed emulsions”.29
The SPI–GA emulsion I and III at pH 7.0 had d43 values of 1.34 μm and 0.67 μm, respectively, while SPI–GA emulsion I with pH below 6.0 also had larger d43 values and FI. The presence of 0.5% GA for the mixed-layer emulsion II resulted in the higher d43 values and FI at pH 3.0–7.0. Compared with SPI–control emulsion III, d43 values of SPI–GA emulsion III showed a slight decrease at pH 5.0 and 6.0, combined with the increase of interfacial protein adsorption.
3.3.2 Protein and polysaccharide adsorption at the oil/water interface. The addition of polyelectrolytes to existing protein-stabilized interfaces seems to have been less frequently investigated than the direct adsorption of protein–polyelectrolyte complexes. Nevertheless, the process of sequential addition can be expected to have a strong influence on the overall adsorption behavior, as well as on the thickness and mechanical strength of the composite interfacial film.As shown in Table 1, when no GA was present at pH 7.0 in the emulsion I or III, the adsorption percentage of SPI onto the droplets was around 60.82–62.85%. With the presence of GA in emulsions I or III, at pH 7.0, 6.0 or 3.0, there were more SPI adsorbed (69.57–96.32%), while there was little adsorption of GA onto the droplet surface at pH 7.0 or 6.0. Khouryieh, Puli, Williams, and Aramouni (2015) reported that xanthan gum does not bind on the whey protein-coated droplet surface at neutral pH, hence will not alter the size of the droplet.30 Jourdain, Leser, Schmitt, Michel, and Dickinson (2008) also found no change of droplet charge and size in mixed emulsion containing sodium caseinate and different concentrations of dextran sulfate at neutral pH, suggesting that there was no electrostatic attraction between sodium caseinate and dextran sulfate.27 Compared at pH 4.0 and 5.0, GA adsorption percentages were significantly higher in SPI–GA emulsion I (66.96–69.47%) than those in SPI–GA emulsion III (14.20–14.85%), which resulted in the higher d43 values. It was noticeable that at pH 3.0, there were more proteins and polysaccharides adsorbed on the interfaces, which might result from the electronic interaction. At pH 6.0, the protein adsorption ratio increased from 60.98% to 95.91% due to the presence of GA with the polysaccharides adsorption ratio of only 0.06%. It seemed that the presence of GA promoted the adsorption of SPI on the interfaces, which might due to the repulsion interaction among them, depletion flocculation.31
3.3.3 Microstructure of the emulsions by CLSM. In contrast to conventional light microscopy, confocal laser scanning microscopy restricts the illumination to a single point, the image, thus, showed a two-dimensional section of the specimen. In Fig. 5, the different types of emulsions were visualized. The oil phase was colored in red and the soy protein phase appeared green. The light green circles around the droplets clearly indicated the protein layer surrounding the red oil phase. The light green hue or the orange hue reflected that both lipid and protein phase were mixed. As expected, the droplet microstructure of these emulsions considerably varied with the types of the emulsion (the order of homogenization and pH adjustment, with or without the presence of GA). For the emulsion I and III, it can be observed that most of the droplets were presented in the separated and unflocculated form at neutral pH, especially at pH 7.0, whereas at pH 6.0, slight flocculation was observed. It could be seen clearly that the droplets in the emulsions at acidic pH flocculated greatly, especially at pH 4.0 and 5.0. For emulsions I, soy protein solutions were adjusted to the corresponding pH, followed by homogenization. At pH 4.0–5.0, near SPI's pI, uncontrolled aggregation of the proteins occurred due to charge neutralization, which dominated by attractive van der Waals and hydrophobic forces.
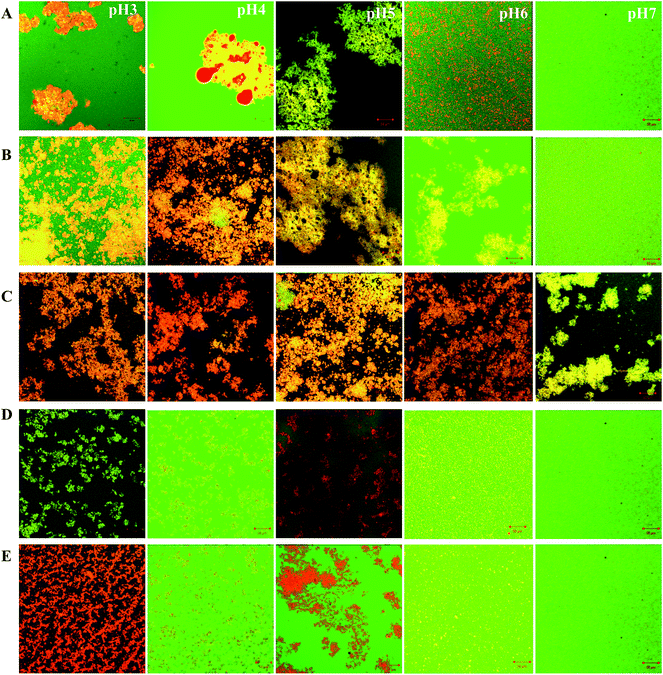 |
| Fig. 5 CLSM images of SPI (A, D prepared by method I, III) and SPI/GA emulsions (B, C and E prepared by method I, II and III) produced from FITC-labeled soy protein and Nile Red-labeled soy oil at different pH. The changes of pH values in B, C, D and E were the same as A. | |
In the case of a protein and polysaccharide mixture, CLSM images showed large orange hue intensive areas at pH 3.0–6.0 (Fig. 5B and C), which also means that the oil droplet flocculation in SPI–GA emulsion I and II were greater than SPI–GA emulsion III. This result was consistent with the findings related to flocculation index (Table 1). At neutral pH, except SPI–GA emulsion II, the emulsions showed a more uniform structure. The nonuniform structure of SPI–GA emulsion II may explained by bridging flocculation because of the introduction of salt ions in the process of emulsion preparation with pH adjustment from 4.0 to 7.0. Salt ions could promote flocculation between droplets via electrostatic screening.12 Additionally, the emulsions with or without GA presented similar microstructures: aggregations and large flocs were observed in emulsions at acidic pHs, no obvious aggregations at neutral pH.
3.3.4 Rheological properties of the emulsions. The rheological properties of the emulsions stabilized by SPI with/without GA were investigated (Fig. 6). All test emulsions were showed shear-thinning behavior, which can also deduced from the flow behavior index n (n < 1). However, the k values were significantly affected by different pH and process. For SPI control emulsions, the k values were higher at pH 4.0 and 5.0 than other pHs, which were similar between SPI–control emulsion I and III. This indicated that acidic pH closing to the isoelectric point increases the viscosity of the emulsions, which may due to the generation of large protein aggregates. Compared to SPI–control emulsions, the SPI–GA emulsions III owned lower k values at pH 4.0 and 5.0 but the k values showed increasing intendency at pH 3.0. The change of rheological properties in the presence of GA may due to the increase of protein solubility at pH 4.0 and 5.0 leading to the decreasing viscosity. However, the protein solubility was reduced because of the formation of insoluble SPI–GA complex at pH 3.0, which increased the emulsion viscosity. According to Fig. 6, the SPI–GA emulsion III possessed the smallest k value at pH 4.0, 5.0 and 6.0. This may be due to the lower degree of flocculation of droplet (Table 1) and the better protein solubility (Fig. 1).
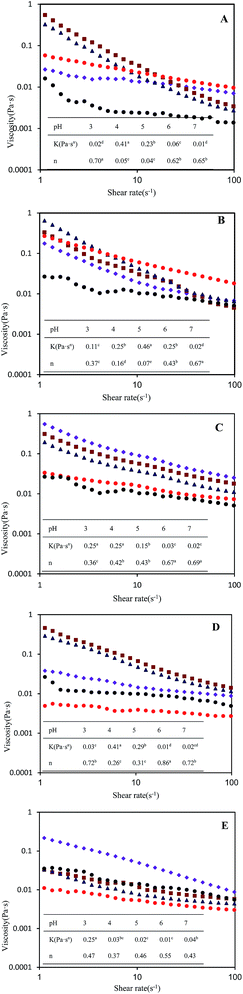 |
| Fig. 6 pH-dependent (pH 3 , pH 4 , pH 5 , pH 6 , pH 7 ) rheological properties of SPI and SPI/GA emulsions prepared by method I (A for SPI emulsion and B for SPI/GA emulsion), method II (C for SPI/GA emulsion) and method III (D for SPI emulsion and E for SPI/GA emulsion). K is the consistency index (Pa sn), and n is the flow behavior index. Means within a row with different letters are significantly different (p < 0.05). | |
3.4 Emulsion stability/creaming stability
Fig. 7 shows the changes in percentage of creaming index (CI%) for all the test emulsions, upon storage up to 2 weeks. For SPI–control emulsions I, distinctly visual creaming occurred after storage of 1 day at pH 4.0 and 5.0, while the presence of GA improved greatly the creaming stability at pH 4.0. This might be due to the formation of soluble SPI–GA complex.32,33 Surprisingly, the creaming stability for SPI–GA emulsion I at pH 5.0 was not improved with CI% of about 50% after 1 day storage. At pH 3.0, SPI–control emulsion I had better creaming stability compared with SPI–GA emulsion I. This could be explained that at pH 3.0, soy proteins carried more positive charges and there were more protein and polysaccharides absorbed on the droplets. At pH 6.0, with the presence of GA (emulsion I), protein adsorption percentage increased to 90.76%. Thus, the bottom of the emulsions became less turbid which might be due to the depletion flocculation as seen from the increase of FI (from 14.56% to 37.78%).
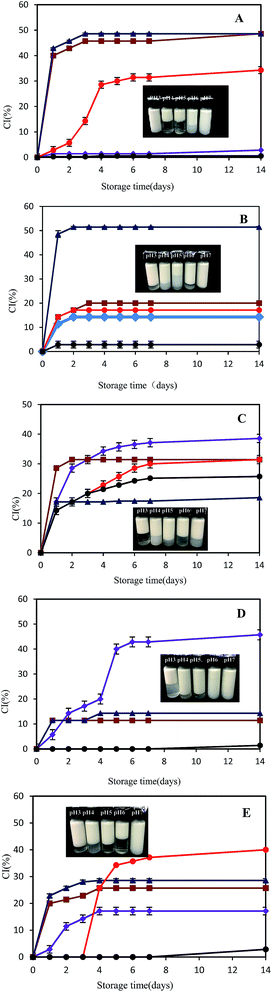 |
| Fig. 7 pH-dependent (pH 3 , pH 4 , pH 5 , pH 6 , pH 7 ) creaming index of SPI and SPI/GA emulsions prepared by method I (A for SPI emulsion and B for SPI/GA emulsion), method II (C for SPI/GA emulsion) and method III (D for SPI emulsion and E for SPI/GA emulsion). The photographs were visual images of emulsions after 14 days storage. | |
For SPI–GA emulsion II, the creaming stability decreased at pH 3.0, 6.0 and 7.0, while it increased at pH 4.0 and 5.0 compared with SPI–control emulsion I. This indicated that mixed-layers emulsions prepared by soluble SPI–GA complex at pH 4.0 were less resistant to pH variations, especially pH below 3.0 or above 6.0. As seen from Table 1, SPI–GA emulsions II at pH 3.0–7.0 all had a high protein adsorption ratio in a range of 95.26–99.08%. When pH decreased from pH 4.0 to 3.0, GA adsorption ratio increased from 69.47% to 97.27%. With the increase of pH from 4.0 to 6.0–7.0, GA adsorption ratio decreased to about 32%. This was due to the stronger or weaker electrostatic interactions between SPI and GA since soy proteins became more positive at pH 3.0 and more negative above pH 6.0 (Fig. 3). Compared with SPI–GA emulsions I, there were more polysaccharides adsorption for SPI–GA emulsions II at pH 6.0–7.0, which indicated the occurrence of the mixed layers on the droplet.
For SPI–control emulsion III, the emulsions were first prepared by homogenization at pH 7.0, then pH was adjusted. Comparably, SPI emulsions III at pH 4.0–7.0 all had a high creaming stability, with no distinct creaming at pH 6.0–7.0 and CI of 10% at pH 4.0–5.0 after 14 days storage. Only emulsions at pH 3.0 were not very stable. After 14 days, the SPI emulsion III at pH 3.0 destabilized as evidenced by the population of flocs ≥100 μm in diameter (data not shown). Similar to SPI–GA emulsions I and SPI–control emulsions III, SPI–GA emulsion III at pH 7.0 was quite stable and exhibited no distinct creaming upon storage up to 8 days (Fig. 7B and E). For SPI–GA emulsions III, about 56.58% GA adsorbed to the droplet surfaces at pH 3, about 14% GA at pH 4–5, but not at pH 6 and 7, which was attributed to electrostatic attraction between anionic groups on the GA and cationic groups on the SPI. With the presence of GA, CI at pH 3.0 was remarkably decreased as compared to the SPI–control emulsion III.
4. Conclusions
The objective of this study was to examine the influence of different preparation routes and pH on the stability of SPI-coated or SPI–GA coated emulsion droplets. The results have shown that the sequence of adjusting pH and homogenizing soy oil with soy proteins greatly influenced the droplet size and the stability of the above referred emulsions. SPI-coated emulsions III are more stable to droplet flocculation which indicated that the adsorbed soy proteins on the O/W interfaces are more resistant to aggregation compared with the aqueous soy proteins in the preparation route I.
GA may either increase or decrease the stability of SPI–GA stabilized emulsions depending on the preparation routes and pH. In the absence of GA, soy proteins emulsions I were unstable to droplet flocculation over the range pH 4–5 which was attributed to their relatively low droplet charge. At a pH value slightly below the pI of SPI, the soluble complexes between SPI and GA formed, which increases the stability of SPI–GA emulsions I at pH 4. The emulsion III showed much lower flocculation rate, and the viscosity of SPI–GA stabilized emulsion decreased significantly, even near protein isoelectric point. This study can be an available reference for the choice of emulsion preparation routes, which would be helpful for the development of emulsion products containing SPI under acidic pH.
Acknowledgements
The work was financially supported by National Natural Science Foundation of China (No. 31201380) and 863 Program (Hi-tech research and development program of China, No. 2013AA102204-3).
References
- D. J. McClements and Y. Li, Adv. Colloid Interface Sci., 2010, 159, 213–228 CrossRef CAS PubMed.
- D. J. McClements, Curr. Opin. Colloid Interface Sci., 2004, 9, 305–313 CrossRef CAS.
- D. I. Comas, J. R. Wagner and M. C. Tomas, Food Hydrocolloids, 2006, 20, 990–996 CrossRef CAS.
- B. Yin, W. Deng, K. Xu, L. Huang and P. Yao, J. Colloid Interface Sci., 2012, 380, 51–59 CrossRef CAS PubMed.
- D. Guzey and D. J. McClements, Adv. Colloid Interface Sci., 2006, 128, 227–248 CrossRef PubMed.
- E. Dickinson, Colloids Surf., B, 2010, 81, 130–140 CrossRef CAS PubMed.
- R. Pongsawatmanit, T. Harnsilawat and D. J. McClements, Colloids Surf., A, 2006, 287, 59–67 CrossRef CAS.
- E. Dickinson, Soft Matter, 2008, 4, 932–942 RSC.
- E. Bouyer, G. Mekhloufi, I. L. Potier and T. D. F. D. Kerdaniel, J. Colloid Interface Sci., 2011, 354, 467–477 CrossRef CAS PubMed.
- M. Corredig, N. Sharafbafi and E. Kristo, Food Hydrocolloids, 2011, 25, 1833–1841 CrossRef CAS.
- E. Dickinson, Food Hydrocolloids, 2011, 25, 1966–1983 CrossRef CAS.
- T. Tran and D. Rousseau, Food Hydrocolloids, 2013, 30, 382–392 CrossRef CAS.
- L. Jourdain, C. Schmitt, M. E. Leser, B. S. Murray and E. Dickinson, Langmuir, 2009, 25, 10026–10037 CrossRef CAS PubMed.
- F. Weinbreck, M. Minor and C. G. de Kruif, J. Microencapsulation, 2004, 6, 667–697 CrossRef PubMed.
- E. Dickinson, Food Hydrocolloids, 2003, 17, 25–39 CrossRef CAS.
- S. Jung, P. C. Roussel and J. L. Briggs, J. Am. Oil Chem. Soc., 2004, 81, 953–960 CrossRef CAS.
- M. Keerati-u-rai and M. Corredig, J. Agric. Food Chem., 2010, 58, 9171–9180 CrossRef CAS PubMed.
- T. Mahendran, P. A. Williams, G. O. Phillips, S. Al-Assaf and T. C. Baldwin, J. Agric. Food Chem., 2008, 56, 9269–9276 CrossRef CAS PubMed.
- Y. Dror, Y. Cohen and R. Yerushalmi-Rozen, J. Polym. Sci., Part B: Polym. Phys., 2006, 44, 3265–3271 CrossRef CAS.
- D. Dong, Y. Hua, Y. Chen, X. Kong, C. Zhang and Q. Wang, J. Agric. Food Chem., 2013, 61, 3934–3940 CrossRef CAS.
- X. Li, Y. Li, Y. Hua, A. Qiu, C. Yang and S. Cui, Food Chem., 2007, 104, 1410–1417 CrossRef CAS.
- O. H. Lowry, N. J. Rosebrough, A. L. Farr and R. J. Randall, J. Biol. Chem., 1951, 193, 265–275 CAS.
- N. Tangsuphoom and J. N. Coupland, Food Hydrocolloids, 2009, 23, 1801–1809 CrossRef CAS.
- M. Dubois, K. A. Gilles, J. K. Hamilton, P. A. Rebers and F. Smith, Anal. Chem., 1956, 28, 350–356 CrossRef CAS.
- W. Peng, X. Kong, Y. Chen, C. Zhang, Y. Yang and Y. Hua, Food Hydrocolloids, 2016, 52, 301–310 CrossRef CAS.
- S. Liu, C. Elmer, N. H. Low and M. T. Nickerson, Food Res. Int., 2010, 43, 489–495 CrossRef CAS.
- L. Jourdain, M. E. Leser, C. Schmitt, M. Michel and E. Dickinson, Food Hydrocolloids, 2008, 22, 647–659 CrossRef CAS.
- Y. Chang and D. J. McClements, Food Hydrocolloids, 2015, 51, 252–260 CrossRef CAS.
- F. Azarikia and S. Abbasi, Food Hydrocolloids, 2016, 59, 26–34 CrossRef CAS.
- H. Khouryieh, G. Puli, K. Williams and F. Aramouni, Food Chem., 2015, 167, 340–348 CrossRef CAS PubMed.
- T. Harnsilawat, R. Pongsawatmanit and D. J. McClements, J. Agric. Food Chem., 2006, 54, 5540–5547 CrossRef CAS PubMed.
- A. Ye, J. Gilliland and H. Singh, Food Hydrocolloids, 2011, 25, 1677–1686 CrossRef CAS.
- T. Vinayahan, P. A. Williams and G. O. Phillips, Biomacromolecules, 2010, 11, 3367–3374 CrossRef CAS PubMed.
|
This journal is © The Royal Society of Chemistry 2017 |