DOI:
10.1039/C7RA01453H
(Paper)
RSC Adv., 2017,
7, 23127-23135
Fluorescent detection of multiple ions by two related chemosensors: structural elucidations and logic gate applications†
Received
4th February 2017
, Accepted 18th April 2017
First published on 26th April 2017
Abstract
Two related chemosensors L1 and L2 display selective detection of multiple ions (Cu2+, Al3+, Cd2+ and S2−) as a result of minor variation of functional groups. Both chemosensors offer identical pyrrole-2-carboxamide fragments; however, they differ by the presence of either an ester (L1) or an acid group (L2) at a remote arene ring. While L1 shows selectivity towards the Cu2+ ion L2 is highly selective for Al3+ and Cd2+ cations as well as S2− anion. A combination of studies (Stern–Volmer plots, detection limits and Benesi–Hildebrand fittings) displays the notable sensing abilities of both chemosensors. Both chemosensors were utilized to develop logic gate-based applications by considering multiple detection of assorted ions. Structural studies provide vital details about the mode of binding between chemosensors and cations. Simple synthesis, multi-stimuli response, fast response, solution visualization, and practical detection methods (filter paper strips and polystyrene films) suggest excellent sensing potentials of both chemosensors.
Introduction
Development of strategies for the detection of multiple ions has received considerable attention due to significance in biological, environment, and chemical assays.1 Detection of multiple ions by a single probe is not only cost-effective and time-saving but is also desirable for one-pot assays.2 Moreover, probes capable of detecting multiple ions have been effectively used for the development of memory devices and molecular logic gates.3 In this direction, molecular logic gates with proper input and output history are appropriate for such memory devices.4 However, progress is still in its infancy and hence multiple inputs/outputs based logic operations are required with better safety level and multiple inputs.5 Notably, inputs and outputs critically depend on the functional groups being offered by a probe and/or a chemosensor.6
It is well known that both cations and anions are required in various biological processes while both their excess as well as deficiency may have detrimental effects.7 For example, essential Cu2+ ion can cause serious complications due to its excess.8,9 Accumulation of aluminum induces several health hazards such as Alzheimer's and Parkinson's diseases while it can also damage the nervous system.10 Contamination of cadmium in environment causes serious environmental and health problems, including lung, prostatic, and renal cancers.11 Similarly, high level of several anions such as sulfide can cause irritation of mucous membrane, and may lead to unconsciousness and respiratory paralysis, Alzheimer's disease, Down's syndrome and liver cirrhosis.12,13 Therefore, detection of several such cations and anions is important due to their momentous effect both on human health and environment.14,15
Synthetic chemosensors have been developed for the individual detection of Cu2+,16,17 Al3+,18 Cd2+,19 and S2− ions.20,21 However, detection of multiple ions by a single chemosensor has been a major challenge.22–24 Martinez-Manez and co-workers have reported detection of multiple ions based on a single chemosensor in its different forms.25 On the other hand, Schmittel and co-workers have achieved technique-dependent selective detection of multiple ions.26 However, development of a single chemosensor, capable of detecting multiple ions, still remains a difficult task. Herein, we present two closely related chemosensors L1 and L2 with adept detection abilities for multiple ions (Cu2+, Al3+, Cd2+ and S2−). These chemosensors include biphenyl moiety as the fluorophore while pyrrole-2-carboxamide and carboxylic acid groups serve as the ion interacting sites (Scheme 1).
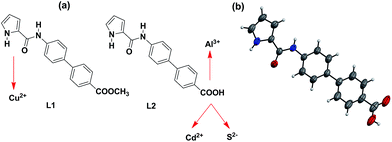 |
| Scheme 1 (a) Chemical drawings of chemosensors L1 and L2 and their mode of interaction with multiple ions; (b) molecular structure of chemosensor L2 wherein thermal ellipsoids are drawn at 50% probability level. | |
Results and discussion
Synthesis and characterization of L1 and L2
Chemosensor L1 was synthesized by the coupling of pyrrole-2-carboxylic acid with methyl-4-amino-[1,1-biphenyl]-4-carboxylate whereas L2 was obtained by the base-assisted hydrolysis of L1. FTIR spectrum shows two N–H stretches at 3405 and 3326 cm−1 for L1 and 3334 and 3284 cm−1 for L2 (Fig. S1 and S2, ESI†). Such stretches have been assigned to the presence of pyrrole-NH and amide-NH resonances.27 Similarly, proton NMR spectrum of L1 displays two signals for two different N–H groups at 9.82 and 11.69 ppm. On the other hand, L2 exhibits two such signals at 9.85 and 11.67 ppm whereas a broad feature at 12.90 ppm corresponds to –COOH group (Fig. S3–S6, ESI†). The ESI+ mass spectra of chemosensors L1 and L2 display [L + H]+ molecular ion peaks at 321.1237 and 307.1079, respectively (Fig. S7 and S8, ESI†). Chemosensor L2 crystallized in P21/c space group and its molecular structure is shown in Scheme 1b. The crystal structure displays that the biphenyl and pyrrole rings exist in the same plane while pyrrole-N and carboxamide-O groups are nicely positioned on the same side to potentially chelate a metal ion.
Detection of Cu2+, Al3+, Cd2+ and S2− ions
The UV-Vis spectra of chemosensors L1 and L2 in CH3OH display intense peaks at 312 nm (44
240 mol−1 cm−1) and 307 nm (41
050 mol−1 cm−1) (Fig. S9, ESI†). Such features are assigned to π–π* transitions within these chemosensors. Both L1 and L2 show intense emission at 418 nm upon excitation at 320 nm in CH3OH. We evaluated the effect of addition of alkali (Na+, K+), alkaline (Mg2+, Ca2+), transition (Cr3+, Co2+, Ni2+, Fe2+, Fe3+, Mn2+, Mn3+, Cu2+, Zn2+, Ag+, Hg2+, Pd2+, Cd2+) and post-transition (Al3+, Pb2+) metal ions on the emission spectra of L1 and L2. Notably, addition of five equivalents (100 μM) of assorted metal ions to L1 (20 μM) did not cause appreciable change. However, addition of Cu2+ ion resulted in considerable quenching in the emission intensity when compared to other metal ions (Fig. 1). Interestingly, in case of L2, Al3+ triggers enhancement with moderate red shift (Δλ = 3 nm) whereas Cd2+ caused notable quenching in the emission with considerable blue shift (Δλ = 25 nm) (Fig. 1).
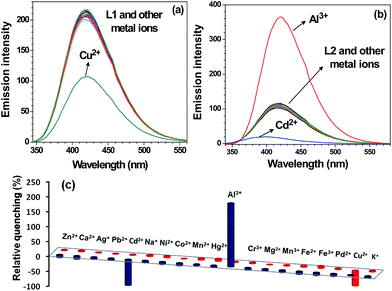 |
| Fig. 1 Emission spectra of chemosensor (a) L1 (20 μM) and (b) L2 (20 μM) in CH3OH and after their interaction with assorted metal ions (100 μM). Bar diagram exhibiting change in emission intensity at 418 nm for L1 (red pillars) and L2 (blue pillars) in presence of assorted metal ions (100 μM). | |
As probe L2 showed emission enhancement in presence of Al3+ ion, hence, we measured the fluorescence spectra of L2 in absence as well as in presence of Al3+ ion in different combinations of CH3OH and H2O (CH3OH/H2O; 100
:
0, 75
:
25, 50
:
50, 25
:
75, 10
:
90, 1
:
99). Fig. S10† shows that increasing water percentage quenches the emission of L2. Similarly, detection of Al3+ ion by chemosensor L2 was also higher in CH3OH when compared to a MeOH–H2O mixture (Fig. S11†). In light of these observations, sensing studies were only performed in CH3OH and not in 100% H2O or HEPES buffer.
The change in the fluorescence intensity of L1 and L2 was investigated by the successive addition of Cu2+, Al3+ and Cd2+ ions (Fig. 2 and 3a). Importantly, Cu2+ ion shows quenching without wavelength shift whereas Al3+ and Cd2+ ions respectively exhibited enhancement and quenching with wavelength shift. To measure the extent of binding, Stern–Volmer constants (KSV),28 detection limits29 and binding constants (Kb)30 were calculated using fluorescence spectral titrations. The Stern–Volmer constants were found to be 9.33 × 103 M−1 and 5.95 × 104 M−1 for Cu2+ and Cd2+ ions, respectively (Fig. S12† and Table 1). Chemosensor L1 showed the detection limit of 1.39 μM for Cu2+ ion while L2 showed detection limits of 0.55 μM and 1.02 μM for Al3+ and Cd2+ ions, respectively (Fig. S13† and Table 1). The binding constants Kb (M−1) were found to be 1.61 × 104, 3.72 × 103 and 1.24 × 105 for Cu2+, Al3+ and Cd2+ ions, respectively (Fig. 3b and Table 1).30
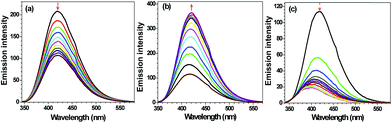 |
| Fig. 2 Change in emission intensity in CH3OH of (a) L1 (20 μM) on exposure to Cu2+ ion (0–100 μM); (b) and (c) L2 (20 μM) on exposure to Al3+ and Cd2+ ions (0–100 μM), respectively. | |
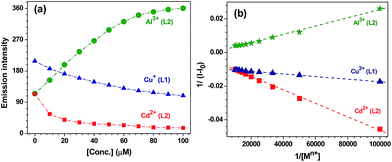 |
| Fig. 3 (a) Change in emission intensity of L1 (20 μM) and L2 (20 μM) at 418 nm with varying concentration of Cu2+, Al3+ and Cd2+ ions (100 μM). (b) Benesi–Hildbrand plots at 418 nm for Cu2+, Al3+ and Cd2+ ions with L1 and L2. | |
Table 1 Stern–Volmer constants (KSV), detection limits and binding constants (Kb) for chemosensors L1 and L2 with Cu2+, Al3+, Cd2+ and S2− ions
Species |
KSV (M−1) |
Detection limit (μM) |
Kb (M−1) |
Fluorescence |
UV-visible |
L1 + Cu2+ |
9.33 × 103 |
1.39 |
1.61 × 104 |
0.77 × 103 |
L2 + Al3+ |
— |
0.55 |
3.72 × 103 |
1.01 × 104 |
L2 + Cd2+ |
5.95 × 104 |
1.02 |
1.24 × 105 |
0.73 × 104 |
L2 + S2− |
4.21 × 106 |
0.01657 |
1.25 × 106 |
1.49 × 104 |
The detection of Cu2+, Al3+ and Cd2+ ions by the photo-responsive chemosensors L1 and L2 was also investigated by the UV-Vis spectral titrations. The intense peak at λmax = 312 nm (44
240 mol−1 cm−1) for L1 (20 μM, CH3OH) was red-shifted by 4 nm in presence of Cu2+ ion (0–100 μM). The observation of two isosbestic points at 288 and 330 nm suggests the formation of a new species (Fig. 4a). Chemosensor L2 (20 μM, CH3OH) displays an intense peak at λmax = 307 nm (41
050 mol−1 cm−1) which was red-shifted to 317 nm (Δλ = 12 nm) on addition of Al3+ ion (0–100 μM). The isosbestic points at 260 nm and 313 nm point towards the generation of a new species (Fig. 4b). The switch-on behavior of chemosensor L2 in presence of Al3+ ion was clearly noted under the UV light (Fig. 4b, inset). Similarly, chemosensor L2 (20 μM, CH3OH) showed a red shift of 5 nm on addition of Cd2+ ion (0–100 μM) with isosbestic points at 250 nm and 310 nm (Fig. S14†). Benesi–Hildebrand fitting of UV-visible spectral titrations provided the binding constants (Kb × 103 M−1) of 0.77, 10.1 and 7.3 for Cu2+, Al3+ and Cd2+ ions, respectively (Fig. S15† and Table 1).31
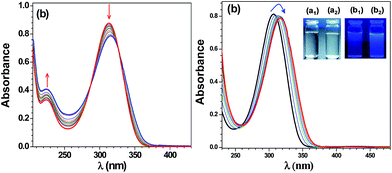 |
| Fig. 4 (a) Change in absorption spectra of chemosensor L1 (20 μM) after addition of different concentrations of Cu2+ ion (0–100 μM). (b) Change in absorption spectra of chemosensor L2 (20 μM) with Al3+ ion (0–100 μM); inset: color change of a CH3OH solution of L2 in absence (a1, b1) and in presence of Al3+ ion (a2, b2) under visible and UV light, respectively. | |
The presence of N–H groups both from pyrrole and amide moieties suggested that chemosensors L1 and L2 may also offer anion sensing abilities. Therefore, we investigated the effect of following anions on the emission of L1 and L2: SCN−, SO42−, SO32−, S2−, S2O82−, S2O72−, C2O42−, CH3COO−, CO3−, HCO3−, Cl−, Br−, I−, NO3−, NO2− and PO43−. In case of L1 (20 μM), addition of 1.2 equivalents (24 μM) of any anion did not cause appreciable change. Importantly, in case of L2 (20 μM), addition of S2− ion resulted in significant quenching in the emission spectrum with a blue shift (Δλ = 7 nm) (Fig. S16† and 5a). The change in fluorescence intensity of L2 was then investigated by the successive addition of S2− ion (Fig. 5b and S17a†). The Stern–Volmer constant (KSV)28 was found to be 4.21 × 106 M−1 suggesting a strong quenching by S2− ion (Fig. S17b† and Table 1).32 A high detection limit of 16.57 nM and a high binding constant (Kb, M−1) of 1.25 × 106 were observed for S2− ion.29,30 (Fig. S18† and Table 1). Detection of S2− ion by chemosensor L2 was also investigated by the UV-visible titration in CH3OH. The intense peak at 307 nm (20 μM) was found to blue shift (Δλ = 4 nm) after the addition of S2− ion (0–30 μM) (Fig. 5c). Benesi–Hildebrand fitting of the UV-visible spectral titration data provided Kb of 1.49 × 104 M−1 (Fig. S19† and Table 1).
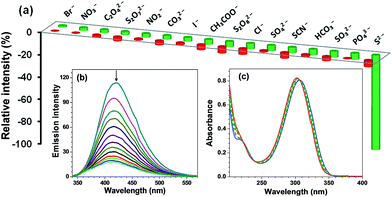 |
| Fig. 5 (a) Bar diagram exhibiting change in emission intensity in CH3OH at 418 nm of chemosensors L1 (20 μM, red pillars) and L2 (20 μM, green pillars) in presence of 1.2 equivalents of different anions (24 μM). (b) Change in emission spectra of L2 (20 μM) on exposure to S2− ion (0–24 μM) in CH3OH. (c) Change in absorption spectra of chemosensor L2 (20 μM) with different concentrations of S2− ion (0–30 μM). | |
Selectivity studies
A good chemosensor should not only offer high sensitivity and fast response time but also high degree of selectivity for an analyte from a mixture. Therefore, selectivity was investigated by performing competitive binding studies of L1 towards Cu2+ and L2 towards Al3+ as well as Cd2+ ion in presence of other ions. As shown in Fig. 6a, no significant change in the emission intensity of chemosensor L1 (20 μM) towards Cu2+ ion was observed in presence of following metal ions (100 μM): Na+, K+, Mg2+, Ca2+, Al3+, Cr3+, Co2+, Fe2+, Fe3+, Mn2+, Ni2+, Mn3+, Zn2+, Ag+, Pb2+, Hg2+, Pd2+, Cd2+. Similarly, no remarkable change in the emission intensity of L2 (20 μM) in presence of Al3+ ion was observed upon addition of (100 μM) of other metal ions. As expected, however, Cd2+ ion showed considerable quenching (Fig. 6b). The selectivity was also investigated for S2− ion in presence of other potential anions by performing competitive binding studies as shown in Fig. 7. In this case also, addition of other interfering anions (24 μM) only caused negligible change in the emission intensity of chemosensor L2 (20 μM).
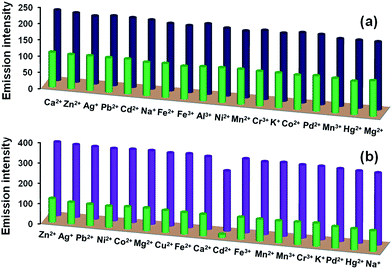 |
| Fig. 6 (a) Selectivity of chemosensor L1 towards Cu2+ ion in presence of other metal ions: L1 + metal ions (blue pillars); and L1 + metal ions + Cu2+ ion (green pillars). (b) Selectivity of chemosensor L2 towards Al3+ ion in presence of other metal ions: L2 + metal ions (green pillars); and L2 + metal ions + Al3+ ion (purple pillars). | |
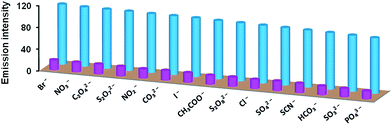 |
| Fig. 7 Selectivity of chemosensor L2 towards S2− ion in presence of other anions: L2 + anions (blue pillars); and L2 + anions + S2− ion (pink pillars). | |
Interestingly, in presence of both Al3+ and Cd2+ ions, emission intensity of chemosensor L2 was found to enhance, hence Al3+ ion is more dominating as compared to Cd2+ ion (Fig. 8, green bars). Similarly, for a combination of Al3+ and S2− ions, Al3+ ion is more dominating as compared to S2− ion and enhances the emission intensity of L2 (Fig. 8, blue bars). Therefore, high degree of selectivity of probe L2 towards Al3+ ion as compared to Cd2+ and S2− ions, was used for the construction of two-input combinational logic circuit.3,4,33,34 The logic circuit and the corresponding truth table was planned in such a way that Al3+ acts as IN 1 while Cd2+/S2− function as the IN 2. The change in emission intensity of L2 at 418 nm was selected as the output with the threshold value of 200 (Fig. 8). The emission above the threshold values is assigned as “ON” whereas emission below the threshold values was allocated as “OFF” for “on” and “off” readout signals, respectively. Fig. 8 displays the simplest symbolic representation of a TRANSFER (Al3+) logic gate in which Al3+ line goes into a YES gate35 whereas Cd2+/S2− line is left unconnected. Herein, YES gate output is the fluorescence intensity at 418 nm.
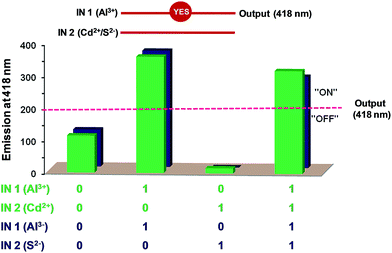 |
| Fig. 8 Emission outputs of L2 at 418 nm in presence of chemical inputs viz. IN 1 = Al3+ and IN 2 = Cd2+ (green bars); IN 1 = Al3+ and IN 2 = S2− (blue bars) and the corresponding two-input combinational logic circuit; dotted line provides guide to eyes. | |
Reversibility studies
Reversibility is an important factor for the optimum functioning of a chemosensor.36 In case of Cu2+ ion, Na2S was used to remove Cu2+ ion to achieve the reversibility.37 Fig. 9a shows that Cu2+ ion quenches the fluorescence of L1; however, addition of sulfide ion restores it (ca. 96%). Reversibility cycles were generated for L1 (20 μM) at 418 nm upon successive addition of Cu2+ (100 μM) and S2− (200 μM) ions (Fig. 9b). Similarly, in order to develop a reversible chemosensor for the detection of Al3+ and Cd2+ ions, K2HPO4 was used to remove Al3+ (red bars) as well as Cd2+ ions (green bars) from L2–Al and L2–Cd systems (Fig. 10a). Reversibility cycles were generated for L2 (20 μM) by the successive addition of Al3+/Cd2+ ions (100 μM) and K2HPO4 (100 μM) (Fig. 10b and b′).
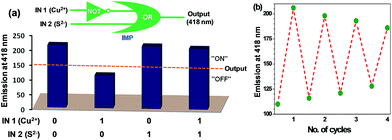 |
| Fig. 9 (a) Emission outputs of L1 at 418 nm in presence of chemical inputs viz. IN 1 = Cu2+ and IN 2 = S2− (blue bars) and the corresponding two-input combinational logic circuit. (b) Reversibility cycles for L1 (20 μM) at 418 nm upon addition of Cu2+ (100 μM) and subsequent regeneration by the addition of Na2S (200 μM); dotted lines provide guide to eyes. | |
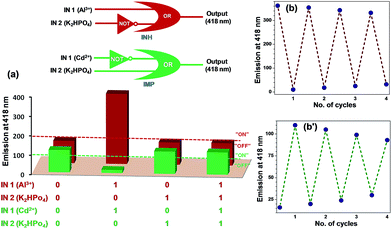 |
| Fig. 10 (a) Emission outputs of L2 at 418 nm in presence of chemical inputs viz. IN 1 = Al3+ and IN 2 = K2HPO4 (red bars); IN 1 = Cd2+ and IN 2 = K2HPO4 (green bars) and the corresponding two-input combinational logic circuit. (b) and (b′) are reversible cycles for L2 (20 μM) at 418 nm upon addition of Al3+ (100 μM) and Cd2+ (100 μM), respectively and subsequent regeneration by the addition of K2HPO4 (200 μM); dotted lines provide guide to eyes. | |
As Na2S and K2HPO4 can be used for achieving reversibility by respectively removing Cu2+ and Al3+/Cd2+ ions; logic circuits and truth tables for chemosensors have been constructed (IN 1 = Cu2+ and IN 2 = S2− for L1, IN 1 = Al3+ or Cd2+ and IN 2 = K2HPO4 for L2). The threshold emission values were fixed at 150 (for L1), 200 and 100 (for L2) at 418 nm (OUT) for the detection of Cu2+, Al3+ and Cd2+ ions, respectively (Fig. 9a and 10a). The emission above and below these threshold values were allocated as “ON” and “OFF” for “on” and “off” output signals, respectively.
Fig. 9a shows the simplest symbolic representation of IMPLICATION (Cu2+) logic gate with two input lines (Cu2+ and S2−) of which Cu2+ line goes into a single-input NOT gate while outputs from NOT gate and S2− line go into an OR gate. Herein, fluorescence intensity at 418 nm is OR gate output. A similar IMPLICATION (Cd2+) logic gate38 was observed for Cd2+–K2HPO4 system (Fig. 10a, green colored truth table and logic gate). Notably, Al3+–K2HPO4 system provided an INHIBIT logic gate39 wherein Al3+ input line goes into a single-input OR gate and K2HPO4 input line goes into NOT gate while output from the NOT gate goes into the OR gate (Fig. 10a, red colored truth table and logic gate). In this case, OR gate output is the fluorescence intensity at 418 nm.
Binding modes
Job's method28,40 of mole fraction suggested 1
:
1 stoichiometry for L1–Cu, L2–Al, L2–Cd and L2–S species (Fig. S20–S22, ESI†). The 1
:
1 stoichiometry was further supported by the Benesi–Hildebrand fitting both from fluorescence as well as UV-Visible spectral titrations (Fig. 3b, 5b, S15 and S19†). Moreover, formation of L1–Cu, L2–Al, L2–Cd and L2–S was also investigated by the UV-Vis titrations (vide supra) in which wavelength shift including isosbestic points confirmed the generation of a new species (Fig. 4, 5c and S14, ESI†). We, however, intended to isolate the product(s) from the reaction between different chemosensors to that of metal salts in order to obtain conclusive structural details.
Subsequently, chemosensor L1 was reacted with CuCl2 whereas L2 was treated with Cd(OAc)2 and Al(NO3)3 salts to obtain the respective product.41 The FTIR spectrum of the blue-colored L1–Cu complex displayed the disappearance of pyrrole N–H group which was noted at 3326 cm−1 in free L1 (Fig. S23†). In addition, νC
O stretches for the ester group exhibited negligible shift thus ruling out its involvement in coordination. On the other hand, νC
O band of amide group displayed a significant blue shift (from 1602 cm−1 in free L1 to 1654 cm−1 in L1–Cu) confirming its coordination through the O-amide atom. The high-resolution ESI-MS spectrum of L1–Cu displayed molecular ion peak at m/z 475.9728 attributed to [{L1–H} + Cu2+ + 2Cl− + Na+ + H+]+ (Fig. S24†). The solution molar conductance of L1–Cu compound displayed non-conducting nature.42 Collectively, these studies suggest a 1
:
1 stoichiometry between L1 and Cu2+ ion forming a L1–Cu compound as displayed in Scheme 2.
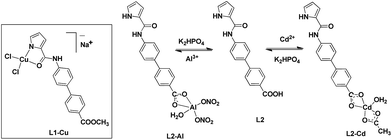 |
| Scheme 2 Chemical drawings of proposed structures of L1–Cu, L2–Al and L2–Cd compounds formed between chemosensors L1/L2 and Cu2+/Al3+/Cd2+ ions and the chemical reversibility for the L2–Al and L2–Cd systems. | |
Finally, blue colored plate-shaped crystals were obtained by the slow evaporation of a CH3OH/CH3CN solution of [L1–Cu] compound. The partial molecular structure however shows a bis-chelated composition, [Cu(L1)2] (Fig. 11).43 The crystal structure shows a distorted square-planar geometry around the Cu(II) ion wherein two deprotonated pyrrole-N and two neutral O-amide atoms constitute the basal plane around the metal ion. We believe that during the crystallization, a thermodynamically stable product, [Cu(L1)2], was crystallized which is different from the solution-based species [L1–Cu] responsible of sensing of Cu2+ ion in solution. Nevertheless, crystal structure of [Cu(L1)2] clearly illustrates the coordination mode of chemosensor L1 and its strong ligating ability.
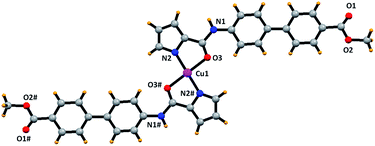 |
| Fig. 11 Ball and stick representation of the partial crystal structure of [Cu(L1)2]. Selected bond distances (Å) and angles (°): Cu1–N2, 1.902; Cu1–O3, 2.014. Selected bond angles (°): O3–Cu1–O3#, 180.0; N2–Cu1–O3, 84.4; N2–Cu1–O3#, 95.6; N2–Cu1–N2, 180.0. | |
On the other hand, FTIR spectra of L2–Al and L2–Cd showed two different N–H signals due to the presence of both pyrrole-NH and amide-NH groups thus suggesting protonated form of L2 (Fig. S25 and S26†). In addition, νC
O stretches for the carboxylate group exhibited moderate red shift thus suggesting its coordination to the metal ion. The 1H NMR spectrum of L2 in presence of Al3+ and Cd2+ ions showed disappearance of COO–H signal which was noted at 12.89 ppm in free L2 (Fig. S27†). Therefore, both FTIR and NMR spectral studies confirm the coordination of L2 through the deprotonated carboxylate group in L2–Al and L2–Cd as shown in Scheme 2. The nature of interaction of L2 with Al3+ and Cd2+ ions was also examined by the high-resolution ESI+ mass spectra. Herein, molecular ion peaks were observed at m/z 475.3264 and 531.2752 that were assigned to [{L2–H + Al3+ + 2NO3− + H2O} + H+]+ (Fig. S28†) and [{L2–H + Cd2+ + CH3COO− + H2O} + Na+]+, respectively (Fig. S29†). The presence of coordinated water in L2–Al and L2–Cd was established by the thermal weight loss (3.4–3.8% for 1H2O) in the TGA studies (Fig. S30, ESI†).
Interestingly, when L2–Cd was crystallized from DMSO–CH3OH, a new compound L2–Cd# was obtained that was structurally characterized. The asymmetric unit of L2–Cd# displays two L2, one Cd(II) ion, and two Cd-coordinated DMSO molecules. The crystal structure of L2–Cd#, shown in Fig. 12, exhibits that a Cd(II) ion is coordinated to two O-carboxylate and two O-amide atoms from two different chemosensor L2 in addition to two DMSO molecules. As a result, two chemosensors L2 hold two Cd(II) ions to generate a large metallacycle. Such metallacycles are further extended via Cd–Oamide bonds to generate a double chain structure. It is suggested that the solution-based species, L2–Cd, slowly reorganizes to form a thermodynamically stable product, L2–Cd#. Importantly, crystal structure of L2–Cd# proves our assumption that chemosensor L2 coordinates preferentially through its O-carboxylate end and not through pyrrole-N part. Interestingly, crystal structures of [Cu(L1)2] and L2–Cd# illustrate that minor changes in a chemosensor (ester versus acid) leads to drastic changes in its sensing abilities.
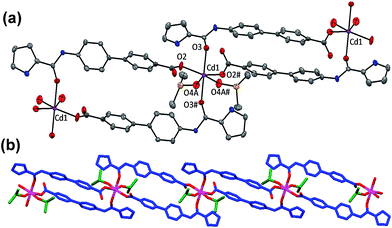 |
| Fig. 12 (a) Crystal structure of L2–Cd# where thermal ellipsoids are drawn at 30% probability level while hydrogen atoms are omitted for clarity. Selected bond distances (Å): Cd1–O2, 2.206(6); Cd1–O3, 2.278(6); Cd1–O4A, 2.299(14). Selected bond angles (°): O2–Cd1–O2#, 122.6(3); O3–Cd1–O3#, 178.3(4); O4A–Cd1–O4A#, 55.3(8); O2–Cd1–O3, 97.8(2); O2–Cd1–O3#, 83.0(2); O2–Cd1–O4A, 91.7(5); O2–Cd1–O4A#, 145.2(5); O4A–Cd1–O3, 91.6(4); O4A–Cd1–O3#, 87.0(4). (b) An extended view of double chain structure of L2–Cd#. | |
The proton NMR titration of chemosensor L2 with 0–2 equivalents (0, 0.5, 1.0, 1.5 and 2.0) of Na2S showed changes in the biphenyl ring protons (Fig. S31†). In addition, a new signal was observed at −3.34 ppm that has been assigned to the HS− species (Fig. S32†).44 Such an experiment suggests that the interaction of S2− ion with the carboxylic acid group of L2 potentially generates HS− species and anionic carboxylate group. Importantly, no change in the emission of L1 (having ester group) in presence of S2− ion strongly support the role of free carboxylic acid in its sensing. In the high-resolution ESI-MS spectrum of [L2–S], a peak was observed in the negative mode with m/z 341.0679 that corresponded to [{L2 + HS− + H+} + H−]− (Fig. S33†). Collectively, these experiments suggest that the recognition of S2− ion is due to its ability to abstract a proton from COO–H group of the chemosensor L2. Literature has suggested similar mode of interaction and recognition for the sulfide ion.45
Monitoring of Al3+ ion in different systems
In literature, most of the Al-sensors only operate in a solvent, thus, limiting the practical applications.46 However, straight-forward and cost-effective detection of Al3+ ion is important for the practical applications. Therefore, “turn-on” sensing of Al3+ ion and its reversibility using K2HPO4 provided us an attractive and cost-effective detection opportunity. Towards such a goal, we fabricated filter-paper test strips as well as polystyrene films for the detection of Al3+ ion. Fig. 13x exhibits that the presence of Al3+ ion in CH3OH enhances the emission of L2 while addition of K2HPO4 quenches the emission. For the monitoring of Al3+ ion, filter-paper test strips were prepared by immersing filter-paper strips in a CH3OH solution of L2 followed by air drying.47 Such test strips successfully detected Al3+ ion by dipping them directly into either CH3OH or even water solution of Al(III) salt (Fig. 13y). Importantly, if such strips were exposed to an aqueous solution of K2HPO4; the original emission of L2 can be retrieved thus providing reversibility. We also attempted to detect Al3+ ion using thin polystyrene films. Such thin films were prepared by doping L2 during the synthesis of polystyrene films28a,48 and demonstrated attractive detection of Al3+ ion as well as its reversibility using a solution of K2HPO4 (Fig. 13z). Both such detection methods suggest potential monitoring of Al3+ ion under environmental as well as industrial conditions.
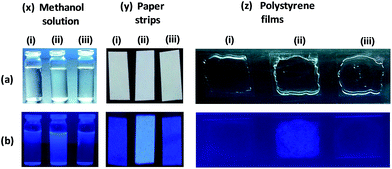 |
| Fig. 13 Detection of Al3+ ion in (x) CH3OH solution (y) paper strips and (z) polystyrene films; (a) and (b) are the images in visible and UV region, respectively. In all cases: (i) displays only L2, (ii) displays L2 in presence of Al3+ ion, (iii) displays L2 in presence of Al3+ ion and K2HPO4. ‘y’ and ‘z’ studies have been performed both in H2O and CH3OH. | |
Conclusions
This report illustrated a simple strategy of developing effective chemosensors for the detection of multiple ions by simply changing the remote functional group. In two related chemosensors, carboxylic acid group was found responsible for the detection of Al3+ Cd2+, and S2− ions whereas a pyrrolecarboxamide fragment was needed for the recognition of Cu2+ ion. Utilizing multiple detection abilities of two chemosensors, different logic gates were developed. The “turn on” sensing of Al3+ ion helped us in developing filter-paper strips as well as polystyrene films based detection strategies. Facile synthesis and multi-stimuli response offered by the present chemosensors may offer potential detection applications.
Experimental section
The discussion about the Stern–volmer constants (KSV), detection limits, binding constants (Kb) and X-ray crystallography as well as figures for the characterization of L1, L2, L1–Cu, L2–Al, L2–Cd and L2–S can be found in the ESI.†
Materials and physical measurements
The analytical grade commercially available reagents were used without further purifications. Stock solutions (10 mM) of cations and anions were prepared in methanol. HPLC grade solvents were used for the UV-Vis and fluorescence spectral measurements and spectra were recorded with a 1.0 cm path length cuvette at ambient temperature. Elemental analysis data were obtained by the Elementar Analysen Systeme GmbH Vario EL-III instrument. The 1H and 13C NMR spectra recorded with a JEOL 400 MHz instrument. The FTIR spectra (Zn–Se ATR) were recorded with a Perkin-Elmer Spectrum-Two spectrometer. The absorption spectra were recorded with a Perkin-Elmer Lambda–25 spectrophotometer. Fluorescence spectral studies were performed with a Cary Eclipse fluorescence spectrometer. ESI-MS mass spectra were measured with an Agilent Q-TOF LC-MS mass spectrometer.
Synthesis of L1 (methyl 4′-(1H-pyrrole-2-carboxamido)-[1,1′-biphenyl]-4-carboxylate)
Pyrrole-2-carboxylic acid (1.0 g, 0.0090 mol) and methyl-4-amino-[1,1-biphenyl]-4-carboxylate (2 g, 0.0090 mol) in 25 mL of pyridine were refluxed for 30 min at 120 °C. P(OPh)3 (3.348 g, 0.0108 mol) was added drop-wise to the aforementioned reaction mixture. The reaction mixture was finally stirred at 80 °C for 12 h. After cooling to room temperature, the reaction mixture was poured into ice-cold water that caused precipitation of the product, which was filtered, washed with water followed by ethanol and dried in vacuum. Yield: 2.2 g (73%). Anal. calc. for C19H16N2O3: C, 71.24; H, 5.03; N, 8.74. Found: C, 71.31; H, 5.12; N, 8.53. FTIR spectrum (cm−1): 3405 cm−1 and 3326 cm−1 (N–H). UV/Vis (MeOH): λmax (ε, mol−1 cm−1) = 312 (44
240). ESI+ mass spectrum (MeOH, m/z): 321.1237 for [L1 + H]+. 1H NMR spectrum (400 MHz, DMSO-d6): δ = 11.69 (s, 1H), 9.87 (s, 1H), 7.98 (d, J = 8.0 Hz 2H), 7.86 (d, J = 8.3 Hz, 2H), 7.80 (d, J = 8.1 Hz, 2H), 7.71 (d, J = 9.32 Hz, 2H), 7.07 (s, 1H), 6.95 (s, 1H) 6.15 (s, 1H), 3.83 (s, 3H). 13C NMR (DMSO-d6, 400 MHz): 166.63, 159.74, 144.81, 142.21, 140.38, 133.49, 130.35, 128.37, 127.75, 126.86, 126.48, 123.35, 120.63, 112.10, 109.52, 52.66.
Syntheses of L2 (4′-(1H-pyrrole-2-carboxamido)-[1,1′-biphenyl]-4-carboxylic acid)
1 g of L1 was dissolved in 10 mL mixture of THF/water (3
:
1) followed by the addition of a saturated aqueous NaOH solution (5 mL). The said mixture was stirred at room temperature for 8 h. The progress of the reaction was monitored by the thin layer chromatography. After the complete hydrolysis, the reaction mixture was neutralized by the drop-wise addition of 1 N HCl. Subsequently, THF was evaporated under the reduced pressure that caused precipitation of a product. The product was filtered, washed with water and dried under vacuum. Single crystals of L2 were grown by the slow evaporation of a DMSO/H2O solution. Yield: 0.827 g (93%). Anal. calc. for C18H14N2O3: C, 70.58; H, 4.61; N, 9.15. Found: C, 70.12; H, 4.52; N, 9.11. FTIR spectrum (cm−1): 3334 cm−1 and 3284 cm−1 (N–H). UV/Vis (MeOH) λmax (ε, mol−1 cm−1) = 307 (41
050). ESI+ mass spectrum (MeOH, m/z): 307.1079 for [L2 + H]+. 1H NMR (DMSO-d6, 400 MHz): δ = 12.89 (s, OH), 11.67 (s, 1H), 9.85 (s, 1H), 7.97 (d, J = 8.3 Hz, 2H), 7.85 (d, J = 8.6 Hz, 2H), 7.70 (d, J = 8.7 Hz, 2H), 7.07 (s, 1H), 6.95 (s, 1H), 6.16 (s, 1H) 13C NMR (DMSO-d6, 400 MHz): 167.72, 159.73, 144.44, 140.25, 133.75, 130.50, 129.56, 127.71, 126.72, 126.50, 123.32, 120.65, 112.09, 109.51.
Fabrication of filter paper strips and polystyrene films
Strips of Whatman filter paper were dipped in a methanol solution of chemosensor L2 and were air-dried.47 Test strips coated with L2 were dipped for a few seconds directly into either aqueous or methanolic solution of aluminum nitrate. In another experiment, these test strips were further dipped in an aqueous or methanolic solution of K2HPO4. Finally, such test strips were investigated under the visible and ultraviolet light.
A mixture of styrene (1 mL), α,α′-azoisobutyronitrile (AIBN; 1 mg) and L2 in methanol (1 mL) was heated on water bath for 30 min at 80 °C.28a,48 A few drops of the above solution were placed over glass slide and the slides were air dried to produce the polystyrene film. Such polystyrene films were used for the detection of Al(III) ion by directly dipping them into either aqueous or methanolic solution of aluminum nitrate. To achieve reversibility, such films were dipped into an aqueous or methanolic solution of K2HPO4. Such polystyrene films were photographed under the visible and ultraviolet light.
Acknowledgements
RG acknowledges the Council of Scientific and Industrial Research (CSIR), New Delhi and the University of Delhi for the financial support. PK thanks UGC, New Delhi for the award of Dr D. S. Kothari Postdoctoral fellowship. VK thanks UGC, New Delhi for award of junior research fellowship. Authors thank CIF-USIC at this university for the instrumental facilities including X-ray data collection. Authors gratefully acknowledge the crystallographic assistance received by an anonymous referee.
Notes and references
-
(a) S. K. Kim and J. L. Sessler, Acc. Chem. Res., 2014, 47, 2525 CrossRef CAS PubMed
;
(b) Y. Huang, F. Li, C. Ye, M. Qin, W. Ran and Y. Song, Sci. Rep., 2015, 5, 9724 CrossRef CAS PubMed
;
(c) M. Ciardi, A. Galan and P. Ballester, J. Am. Chem. Soc., 2015, 137, 2047 CrossRef CAS PubMed
;
(d) V. Lakshmi and M. Ravikanth, J. Mater. Chem. C, 2014, 2, 5576 RSC
. -
(a) B. Daly, J. Ling and A. P. de Silva, Chem. Soc. Rev., 2015, 44, 4203 RSC
;
(b) J. Ling, G. Naren, J. Kelly, D. B. Fox and A. P. de Silva, Chem. Sci., 2015, 6, 4472 RSC
;
(c) J. Ling, B. Daly, V. A. D. Silverson and A. P. de Silva, Chem. Commun., 2015, 51, 8403 RSC
. -
(a) G. de Ruiter and M. E. van der Boom, Acc. Chem. Res., 2011, 44, 563 CrossRef CAS PubMed
;
(b) M. Kumar, N. Kumar and V. Bhalla, Chem. Commun., 2013, 49, 877 RSC
. -
(a) M. Irie, T. Fukaminato, K. Matsuda and S. Kobatake, Chem. Rev., 2014, 114, 12174 CrossRef CAS PubMed
;
(b) X.-J. Jiang and D. K. P. Ng, Angew. Chem., Int. Ed., 2014, 53, 10481 CrossRef CAS PubMed
. -
(a) H. Tian, Angew. Chem., Int. Ed., 2010, 49, 4710 CrossRef CAS PubMed
;
(b) A. P. de Silva and S. Uchiyama, Nat. Nanotechnol., 2007, 2, 399 CrossRef CAS PubMed
;
(c) Y. Wu, Y. Xie, Q. Zhang, H. Tian, W. Zhu and A. D. Q. Li, Angew. Chem., Int. Ed., 2014, 53, 2090 CrossRef CAS PubMed
. - S. Samanta, U. Manna, T. Ray and G. Das, Dalton Trans., 2015, 44, 18902 RSC
. -
(a) L. I. Bruijn, T. M. Miller and D. W. Cleveland, Annu. Rev. Neurosci., 2004, 27, 723 CrossRef CAS PubMed
;
(b) B. Wang, W. Xing, Y. Zhao and X. Deng, Environ. Toxicol. Pharmacol., 2010, 29, 308 CrossRef CAS PubMed
. - C. Vulpe, B. Levinson, S. Whitney, S. Packman and J. Gitschier, Nat. Genet., 1993, 3, 7 CrossRef CAS PubMed
. - P. C. Bull, G. R. Thomas, J. M. Rommens, J. R. Forbes and D. W. Cox, Nat. Genet., 1993, 5, 327 CrossRef CAS PubMed
. -
(a) G. D. Fasman, Coord. Chem. Rev., 1996, 149, 125 CrossRef CAS
;
(b) T. P. Flaten, Brain Res. Bull., 2001, 55, 187 CrossRef CAS PubMed
;
(c) J. R. Walton, Neurotoxicology, 2006, 27, 385 CrossRef CAS PubMed
. -
(a) S. Satarug, J. R. Baker, S. Urbenjapol, M. Haswell-Elkins, P. E. B. Reilly, D. J. Williams and M. R. Moore, Toxicol. Lett., 2003, 137, 65 CrossRef CAS PubMed
;
(b) R. A. Goyer, J. Liu and M. P. Waalkes, BioMetals, 2004, 17, 555 CrossRef CAS PubMed
. -
(a) X. Cao, W. Lin and L. He, Org. Lett., 2011, 13, 4716 CrossRef CAS PubMed
;
(b) R. E. Gosselin, R. P. Smith and H. C. Hodge, Hydrogen sulfide, in Clinical Toxicology of Commercial Products, Williams and Wilkins, Baltimore, MD, 5th edn, 1984, pp. III-198−III-202 Search PubMed. - K. Eto, T. Asada, K. Arima, T. Makifuchi and H. Kimura, Biochem. Biophys. Res. Commun., 2002, 293, 1485 CrossRef CAS PubMed
. -
(a) K. P. Carter, A. M. Young and A. E. Palmer, Chem. Rev., 2014, 114, 4564 CrossRef CAS PubMed
;
(b) S. K. Ko, Y. K. Yang, J. Tae and I. Shin, J. Am. Chem. Soc., 2006, 128, 14150 CrossRef CAS PubMed
. -
(a) J. Chan, S. C. Dodani and C. J. Chang, Nature, 2012, 4, 973 CAS
;
(b) X. L. Zhang, Y. Xiao and X. H. Qian, Angew. Chem., Int. Ed., 2008, 47, 8025 CrossRef CAS PubMed
. -
(a) L. Fabrizzi, M. Licchelli, P. Pallavicini, A. Perotti and D. Sacchi, Angew. Chem., Int. Ed. Engl., 1994, 33, 1975 CrossRef
;
(b) A. Torrado, G. K. Walkup and B. Imperiali, J. Am. Chem. Soc., 1998, 120, 609 CrossRef CAS
;
(c) Y. Li and C. M. Yang, Chem. Commun., 2003, 2884 RSC
;
(d) M. Royzen, Z. Dai and J. W. Canary, J. Am. Chem. Soc., 2005, 127, 1612 CrossRef CAS PubMed
;
(e) Y. Q. Wen, F. Yue, Y. R. Zhong and B. H. Ye, Inorg. Chem., 2007, 46, 7749 CrossRef PubMed
;
(f) S. H. Kim, J. S. Kim, S. M. Park and S.-K. Chang, Org. Lett., 2006, 8, 371 CrossRef CAS PubMed
;
(g) A. Ghorai, J. Mondal, S. Chowdhury and G. K. Patra, Dalton Trans., 2016, 45, 11540 RSC
;
(h) S. Khatua, S. H. Choi, J. Lee, J. O. Huh, Y. Do and D. G. Churchill, Inorg. Chem., 2009, 48, 1799 CrossRef CAS PubMed
;
(i) A. Paul, S. Anbu, G. Sharma, M. L. Kuznetsov, M. F. C. G. da Silva, B. Koch and A. J. L. Pombeiro, Dalton Trans., 2015, 44, 16953 RSC
. -
(a) Q. Wu and E. V. Anslyn, J. Am. Chem. Soc., 2004, 126, 14682 CrossRef CAS PubMed
;
(b) Z. C. Wen, R. H. Yang, H. He and Y. B. Jiang, Chem. Commun., 2006, 106 RSC
;
(c) L. Zheng, E. W. Miller, A. Pralle, E. Y. Isacoff and C. J. Chang, J. Am. Chem. Soc., 2006, 128, 10 CrossRef PubMed
;
(d) Z. C. Xu, Y. Xiao, X. H. Qian, J. N. Cui and D. W. Cui, Org. Lett., 2005, 7, 889 CrossRef CAS PubMed
;
(e) J. J. Lee, Y. W. Choi, G. R. You, S. Y. Lee and C. Kim, Dalton Trans., 2015, 44, 13305 RSC
;
(f) G. K. Li, Z. Xu, X. C. F. Chen and Z. T. Huang, Chem. Commun., 2008, 1774 RSC
;
(g) S. Khatua, S. H. Choi, J. Lee, J. O. Huh, Y. Do and D. G. Churchill, Inorg. Chem., 2009, 48, 1799 CrossRef CAS PubMed
;
(h) G. R. You, G. J. Park, J. J. Lee and C. Kim, Dalton Trans., 2015, 44, 9120 RSC
;
(i) S. H. Choi, K. Pang, K. Kim and D. G. Churchill, Inorg. Chem., 2007, 46, 10564 CrossRef CAS PubMed
;
(j) M. Li, X.-J. Jiang, H.-H. Wu, H.-L. Lu, H.-Y. Li, H. Xu, S.-Q. Zang and T. C. W. Mak, Dalton Trans., 2015, 44, 17326 RSC
. -
(a) S. Goswami, K. Aich, S. Das, A. K. Das, D. Sarkar, S. Panja, T. K. Mondal and S. Mukhopadhyay, Chem. Commun., 2013, 49, 10739 RSC
;
(b) A. Sahana, A. Banerjee, S. Lohar, B. Sarkar, S. K. Mukhopadhyay and D. Das, Inorg. Chem., 2013, 52, 3627 CrossRef CAS PubMed
;
(c) C. Liang, W. Bu, C. Li, G. Men, M. Deng, Y. Jiangyao, H. Sun and S. Jiang, Dalton Trans., 2015, 44, 11352 RSC
;
(d) S. Das, M. Dutta and D. Das, Anal. Methods, 2013, 5, 6262 RSC
;
(e) D. Maity and T. Govindaraju, Chem. Commun., 2010, 46, 4499 RSC
;
(f) K. K. Upadhyay and A. Kumar, Org. Biomol. Chem., 2010, 8, 4892 RSC
;
(g) C.-H. Chen, D.-J. Liao, C.-F. Wan and A.-T. Wu, Analyst, 2013, 138, 2527 RSC
;
(h) J. F. Wang and Y. Pang, RSC Adv., 2014, 4, 5845 RSC
;
(i) Y.-W. Liu, C.-H. Chen and A.-T. Wu, Analyst, 2012, 137, 5201 RSC
;
(j) S. Tao, X. Li, C. Wang and C. Meng, ChemistrySelect, 2016, 1, 3208 CrossRef CAS
. -
(a) X. Peng, J. Du, J. Fan, J. Wang, Y. Wu, J. Zhao, S. Sun and T. Xu, J. Am. Chem. Soc., 2007, 129, 1500 CrossRef CAS PubMed
;
(b) R. T. Bronson, D. J. Michaelis, R. D. Lamb, G. A. Husseini, P. B. Farnsworth, M. R. Linford, R. M. Izatt, J. S. Bradshaw and P. B. Savage, Org. Lett., 2005, 7, 1105 CrossRef CAS PubMed
;
(c) T. Gunnlaugsson, T. C. Lee and R. Parke, Org. Lett., 2003, 5, 4065 CrossRef CAS PubMed
;
(d) W. Liu, L. Xu, R. Sheng, P. Wang, H. Li and S. Wu, Org. Lett., 2007, 9, 3829 CrossRef CAS PubMed
;
(e) T. Cheng, Y. Xu, S. Zhang, W. Zhu, X. Qian and L. Duan, J. Am. Chem. Soc., 2008, 130, 16160 CrossRef CAS PubMed
;
(f) X.-L. Tang, X.-H. Peng, W. Dou, J. Mao, J.-R. Zheng, W.-W. Qin, W.-S. Liu, J. Chang and X.-J. Yao, Org. Lett., 2008, 10, 3653 CrossRef CAS PubMed
;
(g) Z. Xu, G. Li, Y.-Y. Ren, H. Huang, X. Wen, Q. Xu, X. Fan, Z. Huang, J. Huang and L. Xu, Dalton Trans., 2016, 45, 12087 RSC
;
(h) C. Lu, Z. Xu, J. Cui, R. Zhang and X. Qian, J. Org. Chem., 2007, 72, 3554 CrossRef CAS PubMed
;
(i) A. Visscher, S. Bachmann, C. Schnegelsberg, T. Teuteberg, R. A. Matac and D. Stalke, Dalton Trans., 2016, 45, 5689 RSC
;
(j) H.-L. Lu, W.-K. Wang, X.-X. Tan, X.-F. Luo, M.-L. Zhang, M. Zhang and S.-Q. Zang, Dalton Trans., 2016, 45, 8174 RSC
. -
(a) X. Cao, W. Lin and L. He, Org. Lett., 2011, 13, 4716 CrossRef CAS PubMed
;
(b) M. G. Choi, S. Cha, H. Lee, H. L. Jeon and S.-K. Chang, Chem. Commun., 2009, 7390 RSC
;
(c) L. Zhang, X. Lou, Y. Yu, J. Qin and Z. Li, Macromolecules, 2011, 44, 5186 CrossRef CAS
;
(d) K. M. Vengaian, C. D. Britto, K. Sekar, G. Sivaraman and S. Singaravadivel, Sens. Actuators, B, 2016, 235, 232 CrossRef CAS
. -
(a) D. P. Bhopate, P. G. Mahajan, K. M. Garadkar, G. B. Kolekar and S. R. Patil, New J. Chem., 2015, 39, 7086 RSC
;
(b) C. R. Liu, J. Pan, S. Li, Y. Zhao, L. Y. Wu, C. E. Berkman, A. R. Whorton and M. Xian, Angew. Chem., Int. Ed., 2011, 50, 10327 CrossRef CAS PubMed
;
(c) K. Sasakura, K. Hanaoka, N. Shibuya, Y. Mikami, Y. Kimura, T. Koma, T. Ueno, T. Ter, H. Kimura and T. Nagano, J. Am. Chem. Soc., 2011, 133, 18003 CrossRef CAS PubMed
. - H. Komatsu, T. Miki, D. Citterio, T. Kubota, Y. Shindo, Y. Kitamura, K. Oka and K. Suzuki, J. Am. Chem. Soc., 2005, 127, 10798 CrossRef CAS PubMed
. -
(a) Z. Zhang, Y. Zou and C. Deng, RSC Adv., 2017, 7, 14742 RSC
;
(b) S. Qu, C. Zheng, G. Liao, C. Fan, G. Liu and S. Pu, RSC Adv., 2017, 7, 9833 RSC
. -
(a) A. Barba-Bon, A. M. Costero, S. Gil, M. Parra, J. Soto, R. Martınez-Manez and F. Sancenon, Chem. Commun., 2012, 48, 3000 RSC
;
(b) C. Marin-Hernandez, L. E. Santos-Figueroa, M. E. Moragues, M. M. M. Raposo, R. M. F. Batista, S. P. G. Costa, T. Pardo, R. Martinez-Manez and F. Sancenon, J. Org. Chem., 2014, 79, 10752 CrossRef CAS PubMed
;
(c) M. L. Presti, S. E. Sayed, R. Martınez-Manez, A. M. Costero, S. Gil, M. Parra and F. Sancenon, New J. Chem., 2016, 40, 9042 RSC
;
(d) K. Chen, J. W. Bats and M. Schmittel, Inorg. Chem., 2013, 52, 12863 CrossRef CAS PubMed
. - C. Caltagirone, V. Lippolis, F. Isaia, A. Garau, M. A. Scorciapino, A. Casula, R. Martinez-Manez, F. Sancenon, C. Santi, M. Kubicki, A. Owczarzak and A. Llopis-Lorente, Chem. Commun., 2017, 53, 3729 RSC
. -
(a) K. Chen and M. Schmittel, Anal. Bioanal. Chem., 2016, 408, 7077 CrossRef CAS PubMed
;
(b) K. Chen and M. Schmittel, Chem. Commun., 2014, 50, 5756 RSC
;
(c) K. Chen, Q. Shu and M. Schmittel, Chem. Soc. Rev., 2015, 44, 136 RSC
;
(d) K. Chen and M. Schmittel, Analyst, 2013, 138, 6742 RSC
;
(e) Q. Shu, L. Birlenbach and M. Schmittel, Inorg. Chem., 2012, 51, 13123 CrossRef CAS PubMed
. - G. W. Bates, P. A. Gale and M. E. Light, Chem. Commun., 2007, 2121 RSC
. -
(a) D. Bansal and R. Gupta, Dalton Trans., 2016, 45, 502 RSC
;
(b) D. Bansal, G. Kumar, G. Hundal and R. Gupta, Dalton Trans., 2014, 43, 14865 RSC
;
(c) P. Kumar, V. Kumar and R. Gupta, RSC Adv., 2015, 5, 97874 RSC
. - S. Paul, S. Goswami and C. D. Mukhopadhyay, New J. Chem., 2015, 39, 8940 RSC
. - H. A. Benesi and J. H. Hildebrand, J. Am. Chem. Soc., 1949, 71, 2703 CrossRef CAS
. - S. Goswami, K. Aich, A. K. das, A. Manna and S. Halder, Analyst, 2013, 138, 1909 RSC
. - C. Fan, S. Wang, J. W. Hong, G. C. Bazan, K. W. Plaxco and A. J. Heeger, Proc. Natl. Acad. Sci. U. S. A., 2003, 100, 6297 CrossRef CAS PubMed
. -
(a) D. Margulies, C. E. Felder, G. Melman and A. Shanzer, J. Am. Chem. Soc., 2007, 129, 347 CrossRef CAS PubMed
;
(b) L. Feng, Z. Lyu, A. Offenhausser and D. Mayer, Angew. Chem., Int. Ed., 2015, 54, 7693 CrossRef CAS PubMed
;
(c) J. Andreasson and U. Pischel, Chem. Soc. Rev., 2015, 44, 1053 RSC
. -
(a) J. Andreasson and U. Pischel, Chem. Soc. Rev., 2015, 44, 1053 RSC
;
(b) J. Andreasson and U. Pischel, Chem. Soc. Rev., 2010, 39, 174 RSC
;
(c) A. P. de Silva, Molecular Logic-based Computation, The Royal Society of Chemistry, Cambridge, 2013 Search PubMed
. -
(a) D. C. Magri and A. P. de Silva, New J. Chem., 2010, 34, 476 RSC
;
(b) A. P. De Silva and S. Uchiyama, Nat. Nanotechnol., 2007, 2, 399 CrossRef CAS PubMed
;
(c) X. J. Zhao and C. Z. Huang, Analyst, 2010, 135, 2853 RSC
. - A. Visscher, S. Bachmann, C. Schnegelsberg, T. Teuteberg, R. A. Matac and D. Stalke, Dalton Trans., 2016, 45, 5689 RSC
. -
(a) M. Sun, H. Yu, H. Li, H. Xu, D. Huang and S. Wang, Inorg. Chem., 2015, 54, 3766 CrossRef CAS PubMed
;
(b) Y. F. Zhu, D. H. Fan and W. Z. Shen, J. Phys. Chem. C, 2008, 112, 10402 CrossRef CAS
. - R.-R. Gao, S. Shi, Y. Zhu, H.-L. Huang and T.-M. Yao, Chem. Sci., 2016, 7, 1853 RSC
. - S. Goswami, A. Manna, S. Paul, K. Aich, A. K. Das and S. Chakraborty, Dalton Trans., 2013, 42, 8078 RSC
. - A. Senthilvelan, I. Ho, K. Chang, G. Lee, Y. Liu and W. Chung, Chem.–Eur. J., 2009, 15, 6152 CrossRef CAS PubMed
. - In each case, chemosensor L1 or L2 was reacted with the respective metal salt in a 1
:
1 stoichiometry in CH3OH. In all cases, reaction led to a clear solution from which the product was isolated after the removal of solvent under the reduced pressure. Such products were further purified by the forced precipitation from a CH3OH solution using excess diethyl ether. - G. W. Geary, Coord. Chem. Rev., 1971, 7, 81 CrossRef
. - Complex [Cu(L1)2] always produced poorly-diffracting thin plate-like crystals and one such effort resulted in data collection and structure solution.
However, poor structure convergence only allowed partial structure. Crystal data: cell = monoclinic; space group = P21/n; a = 5.0716(19), b = 18.084(6), c = 17.170(5); α = γ = 90, β = 90.06(3); V = 1574.7(9); Z = 2.
- M. D. Hartle, D. J. Meininger, L. N. Zakharow, Z. J. Tonzetich and M. D. Pluth, Dalton Trans., 2015, 44, 19782 RSC
. -
(a) Y. Qian, J. Karpus, O. Kabil, S.-Y. Zhang, H.-L. Zhu, R. Banerjee, J. Zhao and C. He, Nat. Commun., 2011, 2, 495 CrossRef PubMed
;
(b) T. D. Ashton, K. A. Jolliffeb and F. M. Pfeffer, Chem. Soc. Rev., 2015, 44, 4547 RSC
. - S. Paul, A. Manna and S. Goswami, Dalton Trans., 2015, 44, 11805 RSC
. - M. Wang, X. Liu, H. Lu, H. Wang and Z. Qin, ACS Appl. Mater. Interfaces, 2015, 7, 1284 CAS
. - P. Kumar, V. Kumar and R. Gupta, RSC Adv., 2017, 7, 7734 RSC
.
Footnotes |
† Electronic supplementary information (ESI) available: Experimental details for the determination of Stern–Volmer constants, detection limits and binding constants; and X-ray crystallography; figures for FTIR, NMR, mass, absorption, and emission spectra, binding constants, detection limit, optical images, and a table for X-ray data collection. CCDC 1530941 and 1530943. For ESI and crystallographic data in CIF or other electronic format see DOI: 10.1039/c7ra01453h |
‡ These authors contributed equally to this work. |
|
This journal is © The Royal Society of Chemistry 2017 |