DOI:
10.1039/C7RA00952F
(Paper)
RSC Adv., 2017,
7, 21164-21174
Ultrasensitive hydrazine sensor fabrication based on Co-doped ZSM-5 zeolites for environmental safety†
Received
22nd January 2017
, Accepted 3rd April 2017
First published on 13th April 2017
Abstract
Various Co-loaded ZSM-5 zeolites (Co-ZSM-5) were prepared and the details of their structural, morphological and elemental properties characterized by different conventional methods. A flat glassy carbon electrode (GCE) was modified with the Co-ZSM-5 (150%-loading) materials to obtain a sensor for hydrazine (Hyd) which displays improved sensitivity, a large dynamic range and good long-term stability. The calibration plot (best acquired at a voltage of +0.4 V) is linear (r2 = 0.9968) in the 0.01 nM to 0.01 mM Hyd concentration range. The detection limit is as low as 9.1 pM, and the sensitivity is ∼31.6455 μA μM−1 cm−2. To the best of our knowledge, this is the first report on the determination of Hyd using a Co-ZSM-5 (150%-loading)/Nafion/GCE combination with the I–V method for environmental approaches and it has been applied in real industrial effluents and water from the Red Sea with satisfactory results.
1. Introduction
Cobalt-containing ZSM-5 zeolites have drawn a great deal of attention in recent years. They have been used as catalysts for a wide range of important chemical reactions, such as oxidation of styrene and α-pinene,1,2 direct conversion of syngas to gasoline range hydrocarbons,3 Fischer–Tropsch synthesis,4 combustion of isopropanol,5 oxidation of methane to methanol and formaldehyde6,7 and ammoxidation of ethane8 and ethylene.9 High activity was reported for nitrogen oxides abatement over Co-ZSM-5 catalysts. In this context, high N2O direct decomposition activity was reported over Co-ZSM-5.10–14 Earlier work by Armor and Farris10 revealed that the N2O decomposition activity of Co-ZSM-5 is largely unchanged as a result of its hydrothermal treatment using 2% water vapor at 750 °C compared to the dramatic loss of activity of Cu-ZSM-5 under the same treatment. Isothermal oscillations were reported during N2O direct decomposition over Co-ZSM-5.13 Co-ZSM-5 catalysts showed promising activity for the selective catalytic reduction of NOx (SCR) with CH4,14–16 C2H4,17 iso-C4H10,18 and NH3.19 Under NO-SCR conditions, it was suggested that low Co loadings (Co/Al < 0.3), where isolated Co2+ ions are located in charge compensation positions, are the active sites for NO reduction, whereas the Co–oxide species are responsible for the combustion of hydrocarbons.14,18 Various methods have been utilized for the preparation of cobalt-containing ZSM-5 zeolites. These include hydrothermal synthesis, decomposition of metal organic complexes, incipient wetness or through ion exchange. Focusing our attention on the exchange route, this can be performed by sublimation, in a liquid solution or by using the solid state ion exchange (SSIE) method. Depending on the cobalt loading (Co/Al ratio) and the exchange method employed, Co2+, micro-aggregates of cobalt and oxygen, and Co–oxides (CoO and Co3O4) in different coordination geometries were obtained.8,14–18 The SSIE method offers the following advantages; (i) it allows the preparation of over-exchanged M/zeolites (M/Al > 0.5), (ii) it is reproducible, (iii) it avoids the necessity of using large volumes of salt solutions with their accompanying waste, and (iv) it allows the exchange of multivalent cations.8,11,13,20 Active Co-ZSM-5 catalysts for the ammoxidation of ethane and ethylene8 and N2O direct decomposition11,13 were prepared by using the SSIE method. Based on the modification of the zeolites' dielectric constant as a result of the adsorption of a certain molecule, the zeolite-based materials are often applied as sensors with the required selectivity towards certain molecules and not to others.21 In this way, Hagena et al.22 reported the preparation of highly sensitive and selective hydrocarbon Cr2O3/Na-ZSM-5 gas sensors. Franke et al.23 developed a selective ammonia exhaust gas sensor using H-ZSM-5. Sazama et al.24 described the application of AgH-ZSM-5 as a high-temperature sensor of water vapor. Na-ZSM-5 was used as an alkane (C1–C4) sensitive material under partial pressure changes (1–100 kPa) in the presence of O2/CO2/N2 gases.25 Using a ZSM-5-based capacitor sensor, Moos et al.26 were able to detect NH3 without cross-sensitivity to CO, hydrocarbons and O2.
Hyd is a poisonous chemical and frequently considered a cancer-causing, toxic, hazardous, cyanogenetic and nephrotoxic substance.27 Various uses of Hyd include: pesticides, plant-growth regulators, dyes and the photographic industry, pharmaceuticals and the polymer industry, industries related to agriculture, rocket fuel, space craft fuel, and explosives.28 Symptoms of severe exposure to Hyd include: burning in eyes and nose, short-term loss of sight, fainting, vomiting, respiratory edema and unconsciousness. Liver and kidney functions may also be seriously affected by long-term exposure to Hyd.29 The central nervous system can also be affected by Hyd, sometimes leading to unconsciousness. When Hyd is absorbed through the skin, it produces caustic-like burning and also interrupts the production of blood.30 Hence, a superior analytical technique is essential for the detection and quantification of Hyd. Recently, Gang Wei et al.31 have proposed a hydrazine sensor based on a GCE coated with sulfur-doped γ-MnOOH micro-rods and Vellaichamy Ganesan et al.32 have studied a guar gum-based composite coated with palladium nanoparticles for electrochemical Hyd detection, but in both approaches, linear dynamic ranges are very low and they have limitations in detecting nano-level concentrations of Hyd. Electrochemical chemi-sensors always offer a fast, powerful and cost effective method of Hyd detection and quantification. However, electrochemical oxidation of Hyd at a bare-electrode is kinetically slow and associated with high over-potential. Consequently, searching for new materials for the modification of electrodes to enhance the rate of electron transfer and reduce the over-potential of the Hyd oxidation is necessary.33–36 Several redox mediators such as metal nanoparticles,37,38 metal oxides,39 hexacyanoferrate salts,40 and organic mediators41 have so far has been developed for this purpose. Although several Hyd detection methods, including a spectrophotometric method,42,43 a chromatographic method,44 titrimetric method,45 coulometry,46 electrochemistry,47 and fluorescence,48,49 have also been reported elsewhere, all these methods are either expensive or time consuming or need sophisticated instruments, and even sometimes fail to detect nano-level concentrations of Hyd. During the proficient detection of ultra-trace amounts of environmental toxicants, nanomaterials exhibit better properties than their bulk substance: for example, mechanical strength, heat tolerance, electro-catalytic property, electrical conductance, electro-magnetic property, and photo-catalytic property.50 Moreover, due to their low cost, rapid response and higher sensitivity, electrochemical sensors are often more useful than any other methods for Hyd detection.
While a literature survey showed the catalytic application of Co-ZSM-5 in many industrially important reactions, it revealed a lack of information about its sensing applications. Therefore, the purpose of this investigation is to illustrate that Co-ZSM-5 zeolites represent promising materials for selective Hyd sensing applications for environmental approaches. Here, Hyd is extremely carcinogenic and usually seriously damaging to health and environment, therefore detection by using a reliable method with Co-ZSM-5 materials using a GCE electrode is immediately required. In an investigation of Hyd, Co-ZSM-5 materials deposited as thin films on GCE are fabricated and studied in detail for chemical sensor development. An easy-coating method for the construction of thin-layer Co-ZSM-5 materials within conducting binding-agents is executed for the preparation of films on GCE. In this approach, Co-ZSM-5 material fabricated films with conducting binders are utilized to target hazardous analytes using a reliable I–V method under room temperature and pressure. It is confirmed that the fabricated Hyd sensor is a unique and noble research work for ultra-sensitive recognition with active Co-ZSM-5 materials on GCE in a short response-time. In this work, the synthesis, electrochemical characterization, and screening for determination of Hyd using fabricated Co-ZSM-5 materials are reported.
2. Experimental
2.1. Materials and methods
NH4-ZSM-5 zeolite, with aSi/Al ratio of 11.4 (SM 27), was obtained from ALSI Penta Zeolite GmbH (Germany). The preparation of cobalt exchanged ZSM-5 by using the SSIE method has been reported in a number of previous papers.8,11,13 A series of cobalt-exchanged ZSM-5 zeolites were prepared by heating a mechanical mixture of cobalt(II)acetate tetrahydrate (Alfa Aesar) with NH4-ZSM-5 with a target exchange level in the range 10–150% at 500 °C for 3 h in static air. During the heat treatment, solid-state ion exchange occurs, resulting in the formation of Co-ZSM-5 and volatile gases. Then the obtained ion-exchange products were cooled to room temperature, and placed in a bottle. The various obtained solids were referred to by abbreviations Co-ZSM-5_x, where x indicates the exchange level. The laboratory grade chemical reagents, sodium hydroxide, Nafion (5% ethanolic solution), acetone, 2-nitrophenol, 3-methoxyphenol, 4-aminophenol, 4-methoxyphenol, ethanol, hydrazine, pyridine, chloroform, dichloromethane, tetrahydrofuran, monosodium phosphate, and disodium phosphate were purchased from Sigma-Aldrich, and received no further treatment before use. Powder X-ray diffraction (XRD) patterns were recorded by using a Thermo-Scientific ARL X'TRA Powder Diffractometer using Cu Kα radiation (λ = 1.54056 Å) in the 2θ range between 7° and 60° at room temperature. The morphology of the Co-ZSM-5 zeolite was checked on a FESEM instrument (FESEM; JSM-7600F, Japan). Elemental analysis was performed for Co-ZSM-5 by XEDS from JEOL, Japan. The FT-IR spectra were recorded by using a Nicolet iS50 FT-IR spectrometer without KBr employing the Attenuated Total Reflectance (ATR) sampling accessory. Pyridine adsorption was performed in order to estimate the cobalt exchange level of the various Co-ZSM-5 samples. Detailed information about the working procedure followed is reported elsewhere.11,51 The current-vs.-potential (I–V) technique was accomplished to detect the hydrazine in the desired range of electrical potential by a Keithley electrometer (6517A, USA) and the fabricated Co-ZSM-5 (coating with 5% Nafion) assembly functioned as a working electrode in the desired responsive buffer system. The choice of material for various Co-doping ZSM-5 has been described in the ESI† section (Ψ).
2.2. Fabrication of Co-ZSM-5/Nafion/GCE electrode
0.1 M phosphate buffer (PBS-solution) at pH 7.0 was prepared by mixing of an equi-molar concentration of 0.2 M Na2HPO4 and 0.2 M NaH2PO4 solution in 100.0 mL of de-ionized water under room temperature and pressure. To fabricate the GCE, a slurry of Co-ZSM-5 was prepared with ethanol and then coated onto GCE and dried under room temperature and pressure. For the improvement of the adhesive property between GCE and Co-ZSM-5, a drop of 5% ethanolic Nafion solution was added onto the fabricated working electrode. Then the electrode was placed inside an oven at a temperature of 35 °C for an hour and kept there until the conducting film was completely dried. An electrochemical cell was combined with Co-ZSM-5/Nafion/GCE and Pt-wire (dia., 1.5 mm) where Co-ZSM-5/Nafion/GCE acted as the working electrode and Pt-wire was the counter electrode. The different hydrazine solutions (full concentration range: 0.01 nM to 1.0 M) were prepared and incorporated in the assembled electrochemical cell as the target analyte. In the hydrazine detection process, the sensitivity (S) of the prepared working electrode with Co-ZSM-5/GCE was measured from the slope of the calibration curve (the ratio of current versus concentration). In the same way, the detection limit (DL) and linear dynamic range (LDR) were also calculated from the ratio of 3N/S (ratio of noise × 3 vs. sensitivity). The utilized electrometer which provides a constant voltage for I–V measurement is a simple two electrode system. The amount of 0.1 M PBS-solution was kept constant in the electrochemical cell at 5.0 mL throughout the chemical investigation. The I–V response was measured with Co-ZSM-5.
3. Results and discussion
3.1. Structural and morphological characterization of Co-ZSM-5
Fig. 1 displays the XRD patterns of the Co-ZSM-5 samples with various Co-loadings being calcined at 500 °C. In the investigated 2θ range the obtained diffracto-grams reveal the presence of the following reflections 7.92°, 9.06°, 13.97°, 20.38°, 23.28°, 23.98°, 24.54°, 30.02°, and 45.16°. These reflections can be assigned to the (101), (200), (301), (103), (501), (30 3), (313), (503), and (804) crystal planes of ZSM-5 zeolite (JCPDS file no. 79-2401). This indicates the presence of an MFI topology in the cobalt-containing zeolite powders which remains intact during the SSIE with cobalt ions and the calcination processes. The magnified section of Fig. 1 indicates the development of one diffraction peak at 2θ = 36.97° for the samples having an exchange level >50%. This reflection could plausibly be assigned to the presence of traces of Co3O4 spinel oxide (JCPDS file no. 80-1545).52,53 The detection of Co3O4 oxide is in agreement with other data in the literature for cobalt-containing ZSM-5 zeolites prepared by different routes.4,5,54 It was shown that the low angle diffraction peaks of ZSM-5 zeolite are sensitive to the presence of impurities or species inside the zeolite channels; and, therefore, they are used to calculate the variation in crystallinity accompanying the zeolites after synthesis treatments.55 From an inspection of the low angle diffraction peaks in Fig. 1 it appears that the samples with high cobalt exchange levels show a noticeable decrease in intensity of these peaks. Therefore, the diffraction peak at 2θ = 7.92° was used to calculate the crystallinity of the calcined Co-ZSM-5 samples. In comparison with the crystallinity of the H-ZSM-5, the calculated crystallinities for the Co-ZSM-5 samples were 98, 95, 93, 72, 51, and 22% for the cobalt exchange levels of 10, 25, 50, 75, 100, and 150%, respectively. This obtained crystallinity loss accompanying the increase in cobalt content could be ascribed to the possible destruction of some frameworks during the sample preparation. In agreement, El-Bahy et al.54 reported a gradual loss in crystallinity of their Co-ZSM-5, prepared by conventional ion exchange from solution, upon increasing the cobalt wt% from 2 up to 10%. Higher cobalt content has led to disintegration of the ZSM-5 structure.56
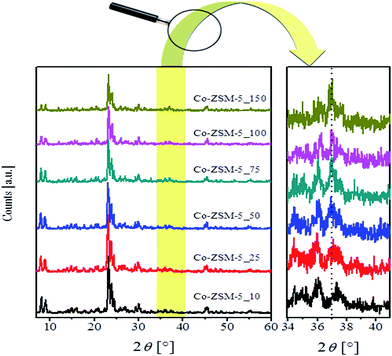 |
| Fig. 1 XRD patterns of the various Co-ZSM-5 zeolites prepared by the SSIE method. | |
The FT-IR spectra of the calcined Co-ZSM-5 samples are shown in Fig. 2. Close inspection of the spectrum of Co-ZSM-5-10 reveals the presence of absorption peaks at 1216 cm−1, 1078 cm−1, 791 cm−1, 544 cm−1 and 445 cm−1. The three absorptions located at 1216 cm−1, 791 cm−1 and 445 cm−1 could be attributed to the T–O bending, symmetric and asymmetric vibrations of the internal tetrahedron of ZSM-5, respectively.57–60 The two peaks at 1216 cm−1 and 544 cm−1 could be ascribed to the external asymmetric and the double five-ring (D5R) vibrations of SiO4 and AlO4 tetrahedra in the ZSM-5.57–60 The same absorptions can be observed for the other Co-ZSM-5 samples. Moreover, the obtained spectra revealed the absence of the peak at around 1465 cm−1 attributable to the ν4 mode of vibration of the NH4+ ion in the zeolite61 or any absorption related to the presence of cobalt acetate.62 It is known that Co3O4 shows two absorption peaks at 560–567 cm−1 and 656–670 cm−1 assignable to the ν1 and ν2 stretching vibrations of the Co–O bond, respectively.53,54 The position of the first Co3O4 peak lies in the absorption range of the D5R tetrahedral vibrations of ZSM-5. Therefore, the existence of Co3O4 cannot be judged from this peak. The spectra of the various Co-ZSM-5 samples indicate the emergence of a new absorption at 673 cm−1 with the increase in cobalt content. The peak could plausibly be assigned to the ν2 stretching vibrations of Co3O4. Thus, the FT-IR results suggest the coexistence of cobalt substituted ZSM-5 and Co3O4 as constituents of the samples with high cobalt content, i.e. the samples with a target exchange level ≥50%. This finding agrees well with the XRD results.
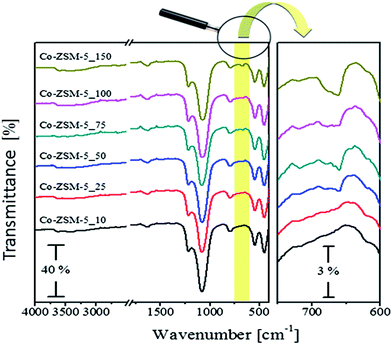 |
| Fig. 2 FTIR spectra of the various Co-ZSM-5 samples prepared by the SSIE method. | |
High resolution FE-SEM images of the calcined Co-ZSM-5 zeolites are exhibited in Fig. 3a–f. The FE-SEM images indicate the morphology of the prepared Co-ZSM-5 zeolites, where the exchange level of NH4+ by Co2+ into NH4-ZSM-5 is (a) 10%, (b) 25%, (c) 50%, (d) 75%, (e) 100%, and (f) 150%. The average diameter of Co-ZSM-5 is calculated to be in the range of 0.6 μm to 1.5 μm, which is close to 1.0 μm. It is noticeable from the FE-SEM images that the simple blending methodology of the prepared products results in Co-ZSM-5 zeolites, as revealed by the aggregated shape and high density of the obtained zeolites. It is also proposed that nearly all of the structure is composed of aggregated Co-ZSM-5 zeolites.63,64
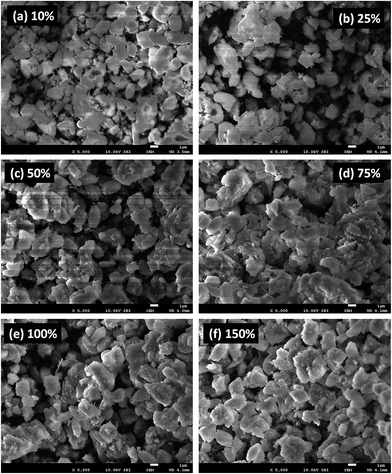 |
| Fig. 3 Low to high magnified FE-SEM images of Co-ZSM-5 zeolites. The exchange level used for NH4– by Co in the zeolite is (a) 10%, (b) 25%, (c) 50%, (d) 75%, (e) 100%, and (f) 150%. | |
X-ray electron dispersive spectroscopy (EDS) was used to investigate the elemental composition of the prepared Co-ZSM-5 materials. It is clearly shown that the prepared Co-ZSM-5 zeolites consist of oxygen, aluminum, silicon, and cobalt elements, as presented in Fig. 4a–f. The composition of O, Al, Si, and Co is presented in the selected area analysis by XEDS in the corresponding table with all exchange levels, and a comparative elemental analysis data is also included in Fig. 4a–f. From inspection of these data it appears that by increasing the loading of Co (from 10% to 150%) into the zeolites, the weight% and atomic% are increased gradually from 10% to a 150% loading. The obtained cobalt content values are close to the theoretical cobalt contents in the prepared Co-ZSM-5 materials, which are 0.33, 0.99, 1.86, 2.76, 3.79, and 6.05 wt% for the exchange levels of 10, 25, 50, 75, 100, and 150%, respectively. No other peak related to any impurity has been detected in the XEDS (Fig. 4), which confirms that the Co-ZSM-5 zeolite products are composed of only O, Al, Si, and Co elements.65
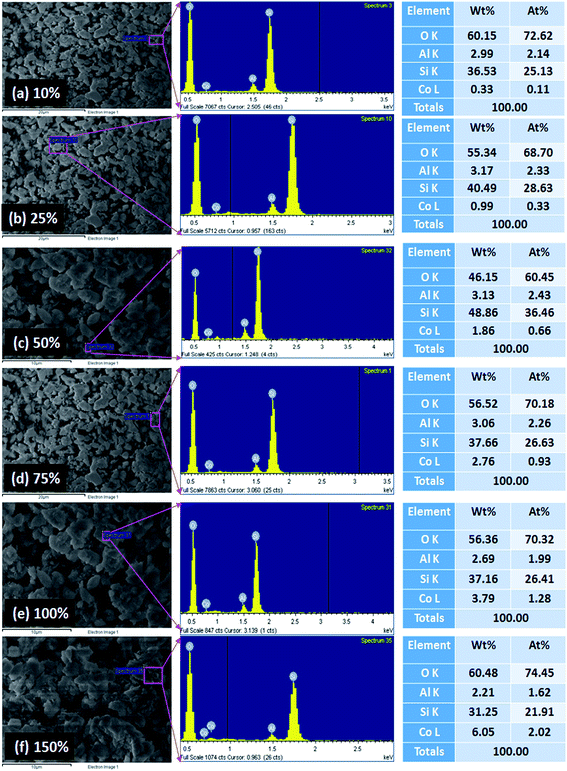 |
| Fig. 4 Selected area of FE-SEM image of Co-ZSM-5 zeolites, corresponding Co-loading, elemental analysis of Co-ZSM-5 zeolites. The exchange level used for NH4– by Co into the zeolites is (a) 10%, (b) 25%, (c) 50%, (d) 75%, (e) 100%, and (f) 150%. | |
3.2. Study of cobalt-exchange level in ZSM-5 zeolites (pyridine adsorption)
The nature of the acid sites and the cobalt exchange level of the various Co-ZSM-5 samples were studied using pyridine adsorption. Fig. 3 shows the FT-IR spectra of adsorbed pyridine on the H-ZSM-5 and the various cobalt-containing ZSM-5 samples. The scanned wavenumber region is 1420–1470 cm−1, where the bands characteristic of both Brønsted (Bpy) and Lewis (Lpy) acids appeared. Pyridine adsorbed on H-ZSM-5 is characterized by the presence of three bands at 1448, 1491 and 1547 cm−1. These absorptions could be assigned to the chemisorbed Lpy, Lpy + Bpy and Bpy, respectively.11,12,66,67 Meanwhile, the spectrum of H-ZSM-5 does not show any band at 1433 cm−1, which is characteristic of physisorbed pyridine molecules on the Na-ZSM-5.11 This, in turn, indicates that the parent NH4-ZSM-5 was a sodium free zeolite. The spectra obtained for the cobalt-containing zeolites reveal the presence of the three absorptions characterizing the Lpy, Lpy + Bpy and Bpy at slightly shifted positions, as shown in Table 1. Fig. 3 clearly indicates that the relative amount of Lewis acid sites increased and that of Brønsted acidity decreased upon increasing the cobalt loading. The area of Bpy with respect to that of the H-form was used to estimate the cobalt exchange level in the various samples. The obtained values are listed in the right-hand column in Table 1. The following points could be raised from an inspection of the obtained values: (i) the obtained exchange level is lower than that of the target level for all the samples, (ii) there is a maximum exchange level that can be obtained using the SSIE method (less than 80%), which is in agreement with a previous report using the same method to prepare various metal exchanged ZSM-5 catalysts,11 and (iii) the lower than expected obtained exchange level is in good agreement with the observed XRD and FT-IR detected of Co3O4 upon increasing the exchange level. Various mixtures were prepared for investigation of pyridine adsorption with different exchange levels and the detailed information is given in the ESI† section (Φ).
Table 1 FT-IR absorption bands for adsorbed pyridine and the pyridine-estimated exchange level of cobalt
Sample |
Lpy [cm−1] |
Lpy + Bpy [cm−1] |
Bpy [cm−1] |
Target exchange level [%] |
Pyridine calculated exchange level [%] |
Co-ZSM-5_10 |
1449 |
1491 |
1545 |
10 |
8 |
Co-ZSM-5_25 |
1450 |
1491 |
1545 |
25 |
19 |
Co-ZSM-5_50 |
1451 |
1491 |
1544 |
50 |
46 |
Co-ZSM-5_75 |
1452 |
1492 |
1543 |
75 |
69 |
Co-ZSM-5_100 |
1452 |
1493 |
1543 |
100 |
74 |
Co-ZSM-5_150 |
1453 |
1493 |
1542 |
150 |
77 |
3.3. Detection of hydrazine with Co-ZSM-5(150%-loading)/Nafion/GCE materials
The potential application of Co-ZSM-5(150%-loading) materials assembled onto GCE as a chemical sensor (especially Hyd analyte in buffer system) has been investigated for measuring and detecting a target selected chemical. Enhancement of the Co-ZSM-5(150%-loading)/GCE as a chemical sensor is in its initial stage and no other reports are available. The Co-ZSM-5(150%-loading)/GCE sensors have advantages such as stability in air, non-toxicity, chemical inertness, electro-chemical activity, simplicity of assembly, ease in fabrication, and chemo-safe characteristics. As in the case of Hyd sensors, the current response in the I–V method of Co-ZSM-5(150%-loading)/GCE changes considerably when aqueous Hyd analyte is adsorbed. The Co-ZSM-5(150%-loading)/GCE is applied for the fabrication of a chemi-sensor, where hydrazine is measured as a target analyte. The fabricated-surface of a Co-ZSM-5(150%-loading)/GCE sensor was prepared with conducting binders (5% ethanolic Nafion solution) on the GCE surface. I–V signals of the Hyd chemical sensor are anticipated for thin film Co-ZSM-5(150%-loading)/GCE as a function of current versus potential. The resultant electrical responses of target Hyd are investigated by a simple and reliable I–V technique using Co-ZSM-5(150%-loading)/GCE. The holding time of the electrometer was set at 1.0 s. A noticeable significant magnification in the current response against applied potential is confirmed. The simple and possible reaction mechanism is generalized in Scheme 1 in the presence of Co-ZSM-5(150%-loading)/GCE surfaces by the I–V method. Hazardous Hyd in aqueous solution was detected and measured using Co-ZSM-5(150%-loading)/GCE as a chemical sensor. Its nontoxic nature, chemical stability and electrochemical activity make Co-ZSM-5(150%-loading)/GCE one of the best sensing materials for Hyd. Upon contact with Co-ZSM-5(150%-loading)/GCE, Hyd gives a significant response in the simple I–V method. Scheme 1(a) shows the fabricated-surface of the Co-ZSM-5(150%-loading)/GCE sensor prepared in 5% ethanolic Nafion solution. The possible oxidation scheme on the Co-ZSM-5(150%-loading)/GCE is generalized in Scheme 1(b); where Hyd is oxidized to N3−, releasing free electrons on the sensor surface during I–V measurements. In the presence of Hyd, electrons are also released from the adsorbed reduced oxygen species on the Co-ZSM-5(150%-loading)/GCE surface, which further increases the current intensity with increasing voltage at room temperature.68,69 A practical I–V response with Hyd and without Hyd on the Co-ZSM-5(150%-loading)/GCE working electrode is given in Scheme 1(c) with a delay time of 1.0 second in the electrometer; where a higher current response to the increasing voltage is clearly demonstrated.
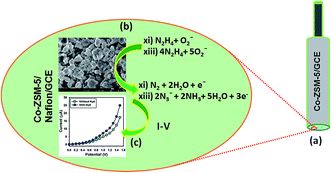 |
| Scheme 1 Scheme representing (a) rod-shaped round-GCE electrode coated with Co-ZSM materials with conducting Nafion (5% ethanol) coating binders, (b) proposed detection mechanism of Hyd, where Hyd is oxidized to N3− by releasing electrons onto Co-ZSM-5(150%-loading)/GCE, and (c) observed I–V response by the Co-ZSM-5(150%-loading)/GCE. Surface area of GCE: 0.0316 cm2; method: I–V. Delay time: 1.0 s. | |
Fig. 5a shows that the differences in the current responses between bare and coated GCE due to the current signals were slightly affected for the coated electrode compared with the bare GCE. The target molecule, Hyd (0.01 nM to 1.0 mM), was added dropwise to the Co-ZSM-5(150%-loading) modified electrode and the changes in current responses were recorded without (light-blue-dotted) and with (blue-dotted) analyte (Fig. 5b). Due to the presence of Co-ZSM-5(150%-loading), a significant enhancement in current is achieved with Hyd which gives a higher surface area with better coverage in absorption and adsorption capability onto the porous zeolite surfaces for the target molecule (Hyd). The responses (current vs. voltage) of the Co-ZSM-5(150%-loading)/GCE were recorded for the different concentrations (0.01 nM to 1.0 mM) of Hyd which indicates that the current of the fabricated electrode changes as a function of Hyd concentration under room temperature and pressure (Fig. 5c) and it was also reported that the current responses increased regularly from lower to higher concentration of the target analyte. For the determination of the probable analytical limit, a broad range of analyte concentrations (0.01 nM to 1.0 mM) were measured from a lower to a higher potential (0 to +1.5 V). From the various concentrations of Hyd, the linear calibration and magnified calibration curve at +0.4 V were plotted (Fig. 5d). The linear dynamic range (0.01 nM to 0.01 mM), regression coefficient (r2: 0.9968), detection limit (9.1 pM) and sensitivity (31.6455 μA μM−1 cm−2; at signal to noise ratio of 3) were calculated from the calibration curve (Fig. 5d).
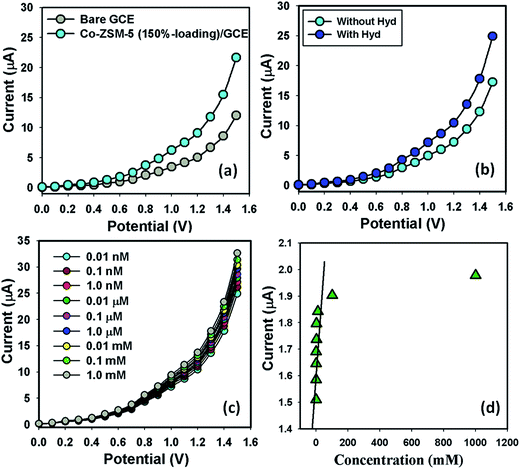 |
| Fig. 5 (a) Uncoated and coated electrode with Co-ZSM-5 (150%-loading) nanostructure materials, (b) absence and presence of Hyd with Co-ZSM-5 (150%-loading) GCE electrode, (c) concentration variation of the Hyd with Co-ZSM-5 (150%-loading) GCE electrode, and (d) calibration curve of the Co-ZSM-5 (150%-loading) materials fabricated on GCE surface. | |
The resistance value of the Co-ZSM-5(150%-loading) modified GCE chemical sensors could be decreased by enhancing the active surface area, which is an important criterion of the Co-ZSM-5(150%-loading).70,71 These reactions are conducted in a bulk-system/air-liquid interface/neighboring atmosphere owing to the small carrier concentration, which increased the magnitude of the resistance. The hydrazine sensitivity towards Co-ZSM-5(150%-loading)/GCE is attributed to the higher-oxygen lacking conducts to enhance the oxygen adsorption. The larger the amount of oxygen adsorbed onto the Co-ZSM-5(150%-loading)/GCE-sensor surface, the higher would be the oxidizing potentiality and the faster would be the oxidation of Hyd. The activity of Hyd would have been extremely high in contrast to other carcinogenic chemicals with the surface under room temperature and pressure.72,73
The selectivity analysis was performed with different chemicals such as pyridine, chloroform, dichloromethane, ethanol, 2-nitrophenol, hydrazine, acetone, 4-methoxyphenol, 3-methoxyphenol, tetrahydrofuran, 4-aminophenol (Fig. 6a). Clear I–V responses are observed in the magnified view of the selected potential area. Hyd showed maximum current responses to a Co-ZSM-5(150%-loading)/GCE fabricated sensor and therefore it was clearly noted that the sensor was most selective towards Hyd compared with other chemicals. The I–V response of the Co-ZSM-5(150%-loading)/GCE coated electrode sensor was studied for up to 2 weeks for the determination of the reusability or reproducibility and long-term stability. It was marked that the current response was not significantly changed after washing in each experiment of the fabricated Co-ZSM-5(150%-loading)/GCE electrode substrate (Fig. 6b). The sensitivity remained similar to the initial value for up to two weeks and after that the responses of the fabricated Co-ZSM-5(150%-loading)/GCE electrode decreased gradually. Under different conditions, a series of seven successive measurements of Hyd solution (0.1 μM) yielded good reproducible responses with the Co-ZSM-5(150%-loading)/GCE (relative standard deviation, RSD: 1.8%; N = 7; Run-1 to Run-7). This small % RSD may be due to the mass variation of the coating materials, Co-ZSM-5, on the GCE working electrodes. When the same working electrode was used in different solutions of the same concentration, even under identical conditions, the current response decreased slightly. This is because after each run, the total number of active sites of the Co-ZSM-5(150%-loading)/GCE decreases slightly. Additionally, a control experiment was also conducted in 0.1 μM Hyd concentration with different Co-loadings into ZSM-5 fabricated electrodes (10% loading, 25% loading, 50% loading, 75% loading, 100% loading, and 150% loading) and a slight increase in current response was marked for the Co-ZSM-5(150%-loading)/GCE compared with other compositions (Fig. 6c). Here, it is observed that the current response exhibited a significantly higher value for higher-Co-loading (150%) compared to other compositions (Fig. 6c) due to the enhancement of Co-dopants into the zeolites to increase the large surface area. Based on the current responses on various compositions, the detailed Hyd sensor development is calculated for 150% Co-loading in a ZSM-5 composition in terms of all analytical parameters such as sensitivity, detection limit, linearity, reproducibility, and selectivity. The higher current response of the fabricated Co-ZSM-5(150%-loading)/GCE could be attributed to the excellent absorption (porous surfaces in Co-ZSM-5(150%-loading)/GCE) and adsorption ability, and high catalytic-decomposition activity of the conduction zeolites.
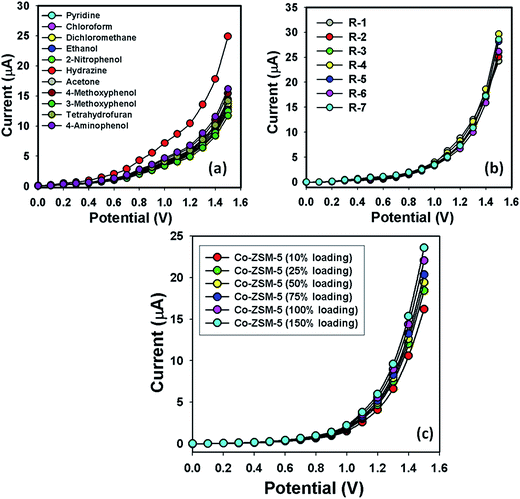 |
| Fig. 6 Co-ZSM-5(150%-loading)/GCE coated electrode for Hyd sensing I–V responses of (a) selectivity study (0.1 μM Hyd) and (b) reproducibility study (0.1 μM Hyd). (c) A control experiment was performed with different percentage loadings of Co-loading into ZSM-5 (10%, 25%, 50%, 75%, 100%, and 150%) in 0.1 μM Hyd. | |
The interior resistance for the fabricated Co-ZSM-5(150%-loading)/GCE sensor decreases with the increasing electron communication characteristics that are important features of zeolites under room temperature and pressure.74–76 The adsorption of oxygen regulates a significant function in the exceptional electrochemical properties of the Co-ZSM-5(150%-loading)/GCE. The adsorption of O2− and/or O− ions decreases the number of electrons in the conduction band and hence increases the resistance of the Co-ZSM-5(150%-loading)/GCE. Dissolved O2 from the aqueous solution or surrounding atmospheric air is adsorbed on the Co-ZSM-5(150%-loading)/GCE surface and ionized according to eqn (i–iii).
|
O2(dissolved) → O2(adsorbed)
| (i) |
|
O2(adsorbed) + e− (Co-ZSM-5/GCE) → O2(adsorbed)−
| (ii) |
|
O2(adsorbed)− + e− (Co-ZSM-5/GCE) → 2O(adsorbed)−
| (iii) |
Oxidation of Hyd to N2 or N3− at the Co-ZSM-5(150%-loading)/GCE surface is the main phenomenon involved in this proposed Hyd sensor. At room temperature, reactive oxygen species (O2− and O−) are chemisorbed onto the pores of Co-ZSM-5(150%-loading)/GCE, where the quantity of such adsorption is mainly dependent on the surface area of the Co-ZSM-5(150%-loading)/GCE. The porous structure gives a very high surface area in the Co-ZSM-5/GCE, which is ultimately responsible for such high adsorption. Extremely high sensitivity of the Co-ZSM-5(150%-loading)/GCE towards Hyd could be attributed to the oxidation of Hyd by adsorbed oxygen species (O2− and O−). The higher the adsorption of oxygen species on the Co-ZSM-5(150%-loading)/GCE, the higher will be the oxidizing power, which will oxidize Hyd quickly, resulting in the very low response time (10 s) of the sensor. The rate of Hyd oxidation in the Co-ZSM-5(150%-loading)/GCE was higher than for other chemicals even under similar conditions, as shown in Scheme 1. When Hyd is oxidized by adsorbed O2− or O− on the Co-ZSM-5(150%-loading)/GCE surface, it converted to N2 or N3−, leaving electrons in the conduction band,77 as given in the following eqn (iv–vii).
|
N2H4 + O2− → N2 + 2H2O + e−
| (iv) |
|
N2H4 + 2O− → N2 + 2H2O + 2e−
| (v) |
|
4N2H4 + 5O− → 2N3− + 2NH3 + 5H2O + 3e−
| (vi) |
|
8N2H4 + 5O2− → 4N3− + 4NH3 + 10H2O + e−
| (vii) |
Current response in the I–V method during Hyd detection largely depends on the dimensions, morphology, and nano-porosity of the Co-doped ZSM-5 zeolites (150%-loading). When the Co-ZSM-5(150%-loading)/GCE surface is exposed to the reducing Hyd, a surface-mediated oxidation reaction takes place. Removal of adsorbed oxygen species (O2− and O−) increases the number of electrons in the conduction-band and hence the surface conductance of the electrode increases. Oxidation of Hyd also provides electrons to the Co-ZSM-5(150%-loading)/GCE surface which further increases the conductance of the working electrode.78–80 Therefore, the current response intensified with the increasing potential. These supplies of electrons quickly increase the conductance of the Co-ZSM-5(150%-loading)/GCE coating. Substantial loading of the Co-ions into ZSM-5 with a porous/large-surface area of morphological zeolites has increased the adsorption ability of the Co-ZSM-5/GCE. The Co-ZSM-5(150%-loading)/GCE sensor requires approximately 10 s to achieve a constant current. This excellent sensitivity and high electrochemical performance of the Co-ZSM-5(150%-loading)/GCE are due to the porous surface that enhances the adsorption and absorption of oxygen species. The Co-ZSM-5(150%-loading)/GCE sensor is highly sensitive towards Hyd and has a lower detection limit than other sensors already reported in Hyd detection,81–92 as given in Table 2. Having a large surface area, the Co-ZSM-5(150%-loading)/GCE offered a positive environment during the detection and quantification of Hyd. The proposed Co-ZSM-5(150%-loading)/GCE sensor has also shown better reliability and stability.93 Despite these developments, there are still numerous important reservations that must be investigated more before the commercial production of this sensor.
Table 2 Comparison of analytical performances of Hyd detection using various materials with conventional electrodes
Electrode fabrication |
Technique/method |
Linear dynamic range (LDR) |
Limit of detection (LOD) |
Sensitivity |
pH |
Ref. |
CNT/GCE catechol derivatives |
CV |
0.50 μM to 1.0 mM |
50 nM |
1.834 nA M−1 m−2 |
7.0 |
81 |
Mn-HFerr/graphite–wax composites |
CV |
3.33 × 10−5 to 8.18 × 10−3 M |
6.65 μM |
0.4753 μA mM−1 m−2 |
7.0 |
82 |
Pd NPs-polyaniline |
CV |
10–300 μM |
0.06 μM |
0.5 μA μM−1 m−2 |
7.0 |
83 |
Ni-hexacyanoferrate/graphite |
Amperometry |
2.4 × 10−6 to 8.2 × 10−3 M |
1.0 μM |
0.0113 μA μM−1 m−2 |
7.0 |
84 |
Ru(II)tetrafluoroborate complex/f-MWCNT |
CV |
5 × 10−6 to 6.5 × 10−3 M |
0.37 μM |
0.010.31 μA μM−1 m−2 |
7.4 |
85 |
Ruthenium/GCE |
CV |
10−5 to 10−2 M |
8.5 μM |
— |
5.0 |
86 |
Mono-dispersed/PEG–ZnS NPs |
Spectroscopy |
1 μM−3 to 3 mM |
1.073 μM |
8.93 μA cm−2 M−1 m−2 |
7.0 |
87 |
AuNPs/graphite pencil |
Amperometry |
— |
3.07 μM |
— |
5.0 |
88 |
Au NPs/colorimetric probe |
Spectrometric |
6.0–40.0 × 10−6 M |
1.1 μM |
— |
7.0 |
89 |
CoS2/CNT NCs/Nafion/GCE |
I–V |
0.1 nM to 1.0 mM |
0.1 nM |
4.430 μA nM−1 m−2 |
7.0 |
90 |
SrO·CNT/GCE |
I–V |
0.2 nM to 0.2 M |
36.0 pM |
26.37 μA mM−1 cm−2 |
7.0 |
91 |
Silica-coated Fe2O3/GCE |
I–V |
0.2 nM to 2.0 mM |
76.0 pM |
12.658 μA mM−1 cm−2 |
7.0 |
92 |
Co-ZSM-5(150%-loading)/GCE |
I–V |
0.01 nM to 0.01 mM |
9.1 pM |
31.6455 μA μM−1 cm−2 |
7.0 |
This work |
3.4. Real sample analysis by Co-ZSM-5/GCE electrode
To confirm the validity of the developed sensor, the Co-ZSM-5/GCE was used as a working electrode to quantify Hyd in industrial effluent-water (collected from the industrial effluent treatment plant, Jeddah, Saudi Arabia) and water from the Red Sea (from the Red Sea coastline, Jeddah, Saudi Arabia). For this purpose, we used the standard addition method to check the precision of the Hyd detection in aqueous samples. Fixed amounts (∼25.0 μL) of aqueous samples of different concentrations along with the same amount of real samples were mixed and analyzed in PBS (5.0 mL) by the Co-ZSM-5/GCE working electrodes. Table 3 shows the results, which demonstrate that the Co-ZSM-5/GCE modified sensor showed a quantitative (∼100%) recovery of Hyd. Based on the results, therefore, it is concluded that the I–V method is suitable, consistent, and appropriate in real sample analysis with the Co-ZSM-5/GCE system.
Table 3 Real sample analysis of Hyd with Co-ZSM-5(150%-loading)/GCE by the I–V methoda
Real sample |
Hyd concentrationb added |
Hyd concentrationb determinedc by the Co-ZSM-5/GCE |
Recoveryc (%) |
RSDd (%) (n = 3) |
S1 and S2: real water samples collected from the industrial effluent treatment plant and Red Sea Jeddah coastline respectively, Jeddah, Saudi Arabia. Mean of the three repeated determinations (S/N = 3) with the Co-ZSM-5/GCE. Concentration of Hyd determined/concentration of Hyd taken. Relative standard deviation value indicates precision among three repeated determinations. |
S1 |
0.01 nM |
0.0102 nM |
102.7 |
2.4 |
S1 |
0.01 nM |
0.0101 nM |
101.4 |
1.8 |
S1 |
0.01 nM |
0.0098 nM |
98.5 |
1.5 |
S2 |
0.01 nM |
0.0103 nM |
103.1 |
2.5 |
S2 |
0.01 nM |
0.0101 nM |
101.4 |
1.8 |
S2 |
0.01 nM |
0.0099 nM |
99.4 |
1.6 |
4. Conclusions
For the first time in this contribution, a Hyd chemical sensor was successfully fabricated based on Co-ZSM-5 (150%-loading) zeolites immobilized on GCE with conducting Nafion binders. A simple fabrication technique was used here to build a Co-ZSM-5 (150%-loading) electrode onto flat GCE using a conductive coating of Nafion binder. The selective and sensitive Hyd sensor was fabricated successfully based on the cobalt-containing zeolite embedded GCE as Co-ZSM-5(150%-loading)/Nafion/GCE. The electrochemical features of the fabricated Hyd sensor were excellent in terms of detection limit, linear dynamic range, sensitivity, repeatability, robustness, stability, and response time. The Co-ZSM-5(150%-loading)/Nafion/GCE assembly exhibits higher sensitivity (31.6455 μA μM−1 cm−2) and a lower detection limit (9.1 pM) compared to previously reported Hyd sensors. The proposed technique can be used for the development of efficient and selective sensors in the health care and environmental fields.
Acknowledgements
The support of the Center of Excellence for Advanced Materials Research (CEAMR) at King Abdulaziz University, Jeddah, Saudi Arabia is gratefully acknowledged.
References
- L. B. Pierella, C. Saux, S. C. Caglieri, H. R. Bertorello and P. G. Bercoff, Appl. Catal., A, 2008, 347, 55–61 CrossRef CAS.
- B. Tang, X. H. Lu, D. Zhou, J. Lei, Z. H. Niu, J. Fan and Q. H. Xia, Catal. Commun., 2012, 21, 68–71 CrossRef CAS.
- S. Sartipi, J. E. van Dijk, J. Gascon and F. Kapteijn, Appl. Catal., A, 2013, 456, 11–22 CrossRef CAS.
- M. Yao, N. Yao, Y. Shao, Q. Han, C. Ma, C. Yuan, C. Li and X. Li, Chem. Eng. J., 2014, 239, 408–415 CrossRef CAS.
- Y. Yan, L. Wang and H. Zhang, Chem. Eng. J., 2014, 255, 195–204 CrossRef CAS.
- N. V. Beznis, A. N. C. van Laak, B. M. Weckhuysen and J. H. Bitter, Microporous Mesoporous Mater., 2011, 138, 176–183 CrossRef CAS.
- Y. K. Krisnandi, B. A. P. Putra, M. Bahtiar, Zahara, I. Abdullah and R. F. Howe, Procedia Chem., 2015, 14, 508–515 CrossRef CAS.
- M. Mhamdi, S. Khaddar-Zine and A. Ghorbel, Appl. Catal., A, 2008, 337, 39–47 CrossRef CAS.
- M. J. M. Mies, E. V. Rebrov, C. J. B. U. Schiepers, M. H. J. M. de Croon and J. C. Schouten, Chem. Eng. J., 2007, 62, 5097–5101 CAS.
- J. N. Armor and T. S. Farris, Appl. Catal., B, 1994, 4, L11–L17 CrossRef CAS.
- B. M. Abu-Zied, W. Schwieger and A. Unger, Appl. Catal., B, 2008, 84, 277–288 CrossRef CAS.
- R. S. da Cruz, A. J. S. Mascarenhas and H. M. C. Andrade, Appl. Catal., B, 1998, 18, 223–231 CrossRef CAS.
- B. M. Abu-Zied and W. Schwieger, Appl. Catal., B, 2009, 85, 120–130 CrossRef CAS.
- P. J. Smeets, Q. Meng, S. Corthals, H. Leeman and R. A. Schoonheydt, Appl. Catal., B, 2008, 84, 505–513 CrossRef CAS.
- C. Chupin, A. C. van Veen, M. Konduru, J. Després and C. Mirodatos, J. Catal., 2006, 241, 103–114 CrossRef CAS.
- F. Lónyi, H. E. Solt, Z. Pászti and J. Valyon, Appl. Catal., B, 2014, 150–151, 218–229 CrossRef.
- X.-M. Chen, X.-F. Yang, A.-M. Zhu, H.-Y. Fan, X.-K. Wang, Q. Xin, X.-R. Zhou and C. Shi, Catal. Commun., 2009, 10, 428–432 CrossRef CAS.
- X. Wang, H.-Y. Chen and W. M. H. Sachtler, Appl. Catal., B, 2000, 26, L227–L239 CrossRef CAS.
- P. N. Panahi, D. Salari, A. Niaei and S. M. Mousavi, Chin. J. Chem. Eng., 2015, 23, 1647–1654 CrossRef CAS.
- B. M. Abu-Zied, A. A. A. Farrag and A. M. Asiri, Powder Technol., 2013, 246, 643–649 CrossRef CAS.
- J. Coronas and J. Santamaria, Chem. Eng. Sci., 2004, 59, 4879–4885 CrossRef CAS.
- G. Hagena, A. Dubbea, F. Rettiga, A. Jergerb, Th. Birkhoferb, R. Müllerb, C. Plogb and R. Moos, Sens. Actuators, B, 2006, 119, 441–448 CrossRef.
- M. E. Franke, U. Simon, R. Moos, A. Knezevic, R. Müller and C. Plog, Phys. Chem. Chem. Phys., 2003, 5, 5195–5198 RSC.
- P. Sazama, H. Jirglová and J. Dědeček, Mater. Lett., 2008, 62, 4239–4241 CrossRef CAS.
- A. Dubbe and R. Moos, Sens. Actuators, B, 2008, 130, 546–550 CrossRef CAS.
- R. Moos, R. Müller, C. Plog, A. Knezevic, H. Leye, E. Irion, T. Braum, K. J. Marquardt and K. Binder, Sens. Actuators, B, 2002, 83, 181–189 CrossRef CAS.
- M. B. Gholivand and A. Azadbakht, Electrochim. Acta, 2011, 56, 10044 CrossRef CAS.
- I. G. Casella, M. R. Guascito, A. M. Salvi and E. Desimoni, Anal. Chim. Acta, 1997, 354, 333 CrossRef CAS.
- A. Salimi, L. Miranzadeh and R. Hallaj, Talanta, 2008, 75, 147 CAS.
- U. P. Azad and V. Ganesan, Electrochim. Acta, 2011, 56, 5766 CrossRef CAS.
- J. Ding, T. Liu, W. Xu, H. Liao, J. Li, G. Wei and Z. Su, RSC Adv., 2015, 5, 80719 RSC.
- P. K. Rastogi, V. Ganesan and S. Krishnamoorthi, Electrochim. Acta, 2014, 125, 593 CrossRef CAS.
- F. Li, B. Zhang, S. Dong and E. Wang, Electrochim. Acta, 1997, 42, 2563 CrossRef CAS.
- J. Li and X. Lin, Sens. Actuators, B, 2007, 126, 527 CrossRef CAS.
- S. Ivanov, U. Lange, V. Tsakova and V. M. Mirsky, Sens. Actuators, B, 2010, 150, 271 CrossRef CAS.
- H. Zhang, J. Huang, H. Hou and T. You, Electroanalysis, 2009, 21, 1869 CrossRef.
- C. B. McAuley, C. E. Banks, A. O. Simm, T. G. J. Jones and R. G. Compton, Analyst, 2006, 131, 106 RSC.
- B. Haghighi, H. Hamidi and S. Bozorgzadeh, Anal. Bioanal. Chem., 2010, 398, 1411 CrossRef CAS PubMed.
- B. Sljukic, C. E. Banks, A. Crossley and R. G. Compton, Electroanalysis, 2006, 18, 1757 CrossRef CAS.
- D. Jayasri and S. S. Narayanan, J. Hazard. Mater., 2007, 144, 348 CrossRef CAS PubMed.
- S. M. Golabi, H. R. Zare and M. Hamzehloo, Microchem. J., 2001, 69, 111 CrossRef CAS.
- A. Safavi and A. A. Ensafi, Anal. Chim. Acta, 1995, 300, 307 CrossRef CAS.
- S. Ganesh, F. Khan, M. K. Ahmed, P. Velavendan, N. K. Pandey and U. Kamachi Mudali, J. Anal. Sci., Methods Instrum., 2012, 2, 98 CAS.
- A. D. Smolenkov, Rev. J. Chem., 2012, 2(4), 329 CrossRef.
- K. R. Rao, R. Rambabu and K. R. Babu, International Journal of Innovative Research in Science, Engineering and Technology, 2013, 2(11), 6378 Search PubMed.
- E. C. Olson, Anal. Chem., 1960, 32, 1545 CrossRef CAS.
- L. Lauko, R. Hudec, K. Lenghartova, A. Manova, F. Cacho and E. Beinrohr, Pol. J. Environ. Stud., 2015, 24(4), 1659 CrossRef CAS.
- G. E. Collins and S. L. R. Pehrsson, Analyst, 1994, Vol. 119, 1907 RSC.
- M. Sun, J. Guo, Q. Yang, N. Xiao and Y. Li, J. Mater. Chem. B, 2014, 2, 1846 RSC.
- M. M. Rahman, H. B. Balkhoyora and A. M. Asiri, RSC Adv., 2016, 6, 29342 RSC.
- S. N. Basahel, M. Mokhtar, E. H. Alsharaeh, T. T. Ali, H. A. Mahmoud and K. Narasimharao, Catalysts, 2016, 6, 57 CrossRef.
- M. T. Makhlouf, B. M. Abu-Zied and T. H. Mansoure, Adv. Powder Technol., 2014, 25, 560–566 CrossRef CAS.
- M. T. Makhlouf, B. M. Abu-Zied and T. H. Mansoure, Met. Mater. Int., 2013, 19, 489–495 CrossRef CAS.
- Z. M. El-Bahy, M. M. Mohamed, F. I. Zidan and M. S. Thabet, J. Hazard. Mat., 2008, 153, 364–371 CrossRef CAS PubMed.
- D. Dumitriu, R. Bârjega, L. Frunza, D. Macovei, T. Hu, Y. Xie, V. I. Pârvulescu and S. Kaliaguine, J. Catal., 2003, 219, 337–351 CrossRef CAS.
- Y. Yan, L. Wang, H. Zhang and X. Zhang, Sep. Purif. Technol., 2017, 175, 213–221 CrossRef CAS.
- T. Xue, Y. M. Wang and M.-Y. He, Microporous Mesoporous Mater., 2012, 156, 29–35 CrossRef CAS.
- M. A. Mohamed, S. A. Halawy and M. M. Ebrahim, J. Therm. Anal., 1994, 41, 387–404 CrossRef CAS.
- H. Huang, H. Huang, Q. Feng, G. Liu, Y. Zhan, M. Wu, H. Lu, Y. Shu and D. Y. C. leung, Appl. Catal., B, 2017, 203, 870–878 CrossRef CAS.
- W. Xia, F. Wang, X. Mu, K. Chen, A. Takahashi, I. Nakamura and T. Fujitani, Catal. Commun., 2017, 91, 62–66 CrossRef CAS.
- M. O. Daramola, K. matamela and O. O. Sadare, J. Environ. Chem. Eng., 2017, 5, 54–62 CrossRef CAS.
- A. Mohamed, S. A. Halawy and M. M. Ebrahim, J. Therm. Anal., 1994, 41, 387–404 CrossRef.
- X. Zhang, Q. Shen, C. He, C. Ma, J. Cheng, Z. Liu and Z. Hao, Catal. Sci. Technol., 2012, 2, 1249 CAS.
- E. F. Iliopoulou, S. Stefanidis, K. Kalogiannis, A. C. Psarras, A. Delimitis, K. S. Triantafyllidis and A. A. Lappas, Green Chem., 2014, 16, 662 RSC.
- Y. Ding, Y. Wang, L. Zhang, H. Zhang, C. M. Li and Y. Lei, Nanoscale, 2011, 3, 1149 RSC.
- M. M. Mohamed and B. M. Abu-Zied, Thermochim. Acta, 2000, 359, 109–117 CrossRef CAS.
- M. Mhamdi, S. Khaddar-Zine and A. Ghorbel, Appl. Catal., A, 2009, 357, 42–50 CrossRef CAS.
- Y. Zhang, Z. Cui, L. Li, L. Guo and S. Yang, Phys. Chem. Chem. Phys., 2015, 17, 14656 RSC.
- J. Liu, H. Chen, Z. Lin and J. M. Lin, Anal. Chem., 2010, 82, 7380 CrossRef CAS PubMed.
- M. Faisal, S. B. Khan, M. M. Rahman, A. Jamal, A. M. Asiri and M. M. Abdullah, Appl. Surf. Sci., 2011, 258, 672 CrossRef CAS.
- W. Zhou, Y. Zhou, Y. Liang, X. Feng and H. Zhou, RSC Adv., 2015, 5, 50505 RSC.
- Y. Zhang, Z. Cui, L. Li, L. Guo and S. Yang, Phys. Chem. Chem. Phys., 2015, 17, 14656 RSC.
- J. Liu, H. Chen, Z. Lin and J. M. Lin, Anal. Chem., 2010, 82, 7380 CrossRef CAS PubMed.
- D. Y. Wang, M. Gong, H. L. Chou, C. J. Pan, H. A. Chen, Y. Wu, M. C. Lin, M. Guan, J. Yang, C. W. Chen, Y. L. Wang, B. J. Hwang, C. C. Chen and H. Dai, J. Am. Chem. Soc., 2015, 137, 1587 CrossRef CAS PubMed.
- M. M. Rahman and A. M. Asiri, RSC Adv., 2015, 5, 63252 RSC.
- M. M. Rahman, A. Jamal, S. B. Khan, M. Faisal and A. M. Asiri, Microchim. Acta, 2012, 178, 99 CrossRef CAS.
- M. M. Rahman, S. B. Khan, A. M. Asiri and A. G. Al-Sehemi, Electrochim. Acta, 2013, 112, 422 CrossRef CAS.
- L. M. F. Dantas, A. P. dos Reis, S. M. C. N. Tanaka, J. H. Zagal, Y. Y. Chen and A. A. Tanaka, J. Braz. Chem. Soc., 2008, 19, 720 CrossRef CAS.
- J. Wu, T. Zhou, Q. Wang and A. Umar, Sens. Actuators, B, 2016, 224, 878 CrossRef CAS.
- S. Nazir and U. Schwingenschlögl, RSC Adv., 2013, 3, 4518 RSC.
- M. M. Rahman, A. Jamal, S. B. Khan and M. Faisal, ACS Appl. Mater. Interfaces, 2011, 3, 1346 CAS.
- A. Salimi, L. Miranzadeh and R. Hallaj, Talanta, 2008, 75, 147 CAS.
- D. Jayasri and S. S. Narayanan, J. Hazard. Mater., 2007, 144, 348 CrossRef CAS PubMed.
- S. Ivanov, U. Lange, V. Tsakova and V. M. Mirsky, Sens. Actuators, B, 2010, 150(271), 60–65 Search PubMed.
- S. J. R. Prabakar and S. S. Narayanan, J. Electroanal. Chem., 2008, 617, 111 CrossRef CAS.
- I. Tiwari, M. Gupta, P. Sinha and C. E. Banks, Mater. Res. Bull., 2014, 60, 166 CrossRef CAS.
- J. S. Pinter, K. L. Brown, P. A. DeYoung and G. F. Peaslee, Talanta, 2007, 71, 1219 CrossRef CAS PubMed.
- S. K. Mehta, Khushboo and A. Umar, Talanta, 2011, 85, 2411 CrossRef CAS PubMed.
- M. A. Aziz and A. N. Kawde, Talanta, 2013, 115, 214 CrossRef PubMed.
- B. Zargar and A. Hatamie, Sens. Actuators, B, 2013, 182, 706 CrossRef CAS.
- M. M. Rahman, J. Ahmed, A. M. Asiri, I. A. Siddiquey and M. A. Hasnat, RSC Adv., 2016, 6, 90470–90479 RSC.
- M. M. Rahman, M. M. Hussain and A. M. Asiri, RSC Adv., 2016, 6, 65338–65348 RSC.
- H. Akhter, J. Murshed, M. A. Rashed, Y. Oshima, Y. Nagao, M. M. Rahman, A. M. Asiri, M. A. Hasnat, M. N. Uddin and I. A. Siddiquey, J. Alloys. Compounds, 2017, 698, 921–929 CrossRef CAS.
Footnote |
† Electronic supplementary information (ESI) available. See DOI: 10.1039/c7ra00952f |
|
This journal is © The Royal Society of Chemistry 2017 |