DOI:
10.1039/C7RA00910K
(Paper)
RSC Adv., 2017,
7, 21128-21135
Cu@Ni core–shell nanoparticles/reduced graphene oxide nanocomposites for nonenzymatic glucose sensor†
Received
21st January 2017
, Accepted 6th March 2017
First published on 12th April 2017
Abstract
In this work, the Cux@Ni100−x core–shell nanoparticles (CSNPs) are deposited on reduced graphene oxide (rGO) sheets, and this nanocomposites (in a Nafion matrix) are shown to be a viable materials for nonenzymatic sensing of glucose. A novel nonenzymatic glucose sensor based on a glass carbon electrode modified with Cu53@Ni47 CSNPs/rGO (referred to as Cu53@Ni47 CSNPs/rGO/GCE) displays an enhanced electrocatalytic activity to glucose oxidation in 0.1 M NaOH solution than that of Cu/GCE, Ni/GCE, Cu/rGO/GCE, Ni/rGO/GCE, and Cu52@Ni48 CSNPs/GCE, respectively. This is attributed to the three-in-one synergetic effects from their bimetallic compositions, specific core–shell structures, and interactions from the bimetallic CSNPs and support materials of rGO sheets. At an applied potential of +0.575 V (vs. SCE), the electrode has a low detection limit (0.5 μM; S/N = 3), a very wide linear range (0.001 mM to 4.1 mM), high sensitivity (780 μA mM−1 cm−2), and a fast response time (3 s). Thus, it has great potential for the development of nonenzymatic glucose sensors.
1 Introduction
The development of electrochemical glucose sensors has attracted considerable attention over the past few years because of the importance of fast and reliable glucose concentration determination in clinical diagnostics.1 However, enzyme-modified electrodes have several disadvantages, namely, high enzyme costs, instability, complicated immobilization procedures, and critical operating conditions.1 In order to resolve these problems, numerous efforts have focused on developing nonenzymatic electrodes. Various nanostructured metals, alloys, and metal oxides had been explored extensively because of their unique physical and chemical properties, such as increased surface area, fast mass transport, and notable catalytic activity.2 Among these, non-noble metal Ni- or Cu-based nanomaterials exhibit remarkable electrocatalytic abilities for glucose.3,4 However, pure metals, such as Ni and Cu, are difficult to prepare and have poor stability for electroanalysis because they readily oxidize in air and in solution.5 Therefore, much more attention needs to be paid to develop Ni- or Cu-based nanostructures and their composites for improving the oxidation rate and stability of active materials.
On the one hand, the stability, chemical activity, and poisoning resistance of bimetallic or intermetallic compound electrode materials can be adjusted by controlling their morphologies, structures, compositions, or sizes. For instance, PdCu/GE,6a bilayer Ni/Cu porous nanostructured film,6b Ni–Cu/TiO2 NTs,6c Ni/Cu/MWCNT,6d Cu–Co alloy dendrite,6e and Pt/Ni NWAs6f electrodes are prepared by different methods, and which all show a higher sensitivity for the quantitative determination of glucose than single-metal composite because of their large exposed area and excellent diffusion properties.6 In recent years, nanoparticles (NPs) with core–shell (CS) structures are attracting attention because of their three major effects: ligand effect, ensemble effects, and geometric effects, which can be enhance the catalytic activities.7
On the other hand, carbon materials have been used as a matrix to enhance electron transfer rates and electrocatalytic activities.8 As one important carbon material, graphene reveal a significant impact in fields of science and technology because of its remarkable physical and chemical properties.9 The unique properties of graphene, such as remarkable surface area, excellent conductivity, and wide electrochemical range, make it an ideal material in electrochemical sensors.10 For example, Ni–Co NSs/rGO11a and Cu–Co NSs/rGO–CHIT11b composites exhibit higher catalytic activities than their bimetallic nanostructures without graphene.
According to the previous reports, the catalytic performances of composites should be enhanced by alloy, bimetal, or multimetallic, and suitable supports. These studies indicated that use of graphene-modified bimetallic electrodes resulted in well-performing glucose sensors. And yet for all that, the higher detection limit and narrower linear range of those sensors are observed.6a,11a,b Thereby, taking advantages of reduced graphene oxide sheets together with advanced features of the bimetallic Cu/Ni and its specific core–shell structure, which can enhance the electrocatalytic activity, biocompatibility, and sensitivity for biosensor applications.
Herein, we report a facile and one-pot solvothermal method to prepare of Cux@Ni100−x core–shell nanoparticles (CSNPs) decorated reduced graphene oxide (rGO) nanocomposites (NCs) (namely, Cux@Ni100−x CSNPs/rGO NCs). In this work, a series of enzymatic-free glucose sensors based on Cux@Ni100−x CSNPs/rGO NCs modified glassy carbon electrode (GCE), Cu/GCE, Ni/GCE, and Cu52@Ni48 CSNPs/GCE are constructed, and their electrochemical properties and electrocatalytic activities are investigated in details. What's exciting is that the Cu53@Ni47 CSNPs/rGO/GCE shows a good electrocatalytic performance for glucose oxidation, and which displays a lower detection limit (0.5 μM; S/N = 3), and a wider linear range (0.001 mM to 4.1 mM), high sensitivity (780 μA mM−1 cm−2), and a fast response time (3 s), respectively.
2 Experimental
Chemicals and reagents
Glucose was purchased from Sinopharm Chemical Reagent Co. (China). Graphene oxide (GO) was purchased from Nanjing XFNANO Materials Tech Co. Ltd (China). Ultrapure water was used throughout the experiments. All reagents were of analytical grade and without further purifications.
Synthesis of Cu53@Ni47 CSNPs/rGO NCs
20 mg GO sheets was ultrasonically dispersed in 20 mL ethylene glycol (EG) for 5 h to form GO suspension. Then 20 mL EG solution including 0.5 mmol CuCl2·2H2O and 0.5 mmol NiCl2·6H2O was added drop wise the GO suspension under stirring to form a uniform dispersion. Subsequently, 5 mL EG solution with 0.01 mol NaOH was added dropwise into the above solution and being vigorously stirred for 1 h and then the mixture was transferred into a Teflon-lined stainless-steel autoclave with a capacity of 60 mL. The autoclave was sealed and put into a furnace, which was heated to and maintained at 200 °C for 5 h, and taken out and allowed to cool naturally to room temperature. Finally, the precipitates was collected by centrifugation and washed repeatedly with water and ethanol, and then vacuum dried for overnight and the Cu53@Ni47 CSNPs/rGO NCs were obtained. By the same conditions, the Cux@Ni100−x CSNPs/rGO NCs with different initial Cu/Ni molar ratios, Cu NPs, Ni NPs, and Cu52@Ni48 CSNPs were also prepared.
Characterization
The obtained samples were characterized by X-ray diffraction measurement (XRD, Shimadzu XRD-6000, Cu-Kα radiation, λ = 1.5406 Å), field emission scanning electron microscope (SEM, Hitachi S-4800) and transmission electron microscopy (TEM, FEI Tecnai G2 20). The chemical compositions of final products were determined by inductively coupled plasma-atomic emission spectrometer (ICP-AES, Varian 710-ES) and X-ray photoelectron spectra (XPS, Thermo ESCALAB 250XI). Elemental mapping and high-angle annular dark field-scanning transmission electron microscope (HAADF-STEM) were carried out on a JEM-2100F microscope equipped with an energy-dispersive X-ray analysis system. All electrochemical experiments were performed with the CHI 660C (Chenhua, Shanghai) electrochemical workstation. A three electrode cell was used for the electrochemical experiments. The working electrodes were as-prepared Cux@Ni100−x CSNPs/rGO, Cu NPs, Ni NPs, and Cu52@Ni48 CSNPs/Nafion modified glassy carbon electrode (GCE, 3 mm in diameter) with a saturated calomel electrode (SCE) and a platinum wire as the reference auxiliary and the auxiliary electrode.
Preparation of modified electrode
A GCE was used as the conducting substrate for the development of the sensor platform. The GCE was carefully polished in 0.3 and 0.05 μm alumina slurry, respectively, and washed with ultrapure water followed by sonication in water. The catalyst suspension was prepared by dispersed 4 mg Cux@Ni100−x CSNPs/rGO NCs into 4 mL DMF containing 0.05% Nafion through ultrasonic treatment for 60 min. Then 6 μL of the corresponding suspension was coated on the GCE and dried in air to obtain sensors. For comparison, Cu NPs, Ni NPs, and Cu52@Ni48 CSNPs modified GCEs were also prepared by the same method.
3 Results and discussion
Material characterization
A facile and one-pot solvothermal method synthesis of Cux@Ni100−x CSNPs/rGO NCs is presented in this study. The hybrid NCs are prepared through the chemical reduction of Ni2+ and Cu2+ by using EG as a reducing agent at alkaline condition. Moreover, Cu NPs, Ni NPs, and Cu52@Ni48 CSNPs are also fabricated via the same procedures without GO sheets. Phase structure and purity of as-synthesized samples were examined via X-ray powder diffraction (XRD). Fig. 1 shows the typical XRD patterns of GO (Fig. 1A), Cu NPs (Fig. 1B), Cu/rGO (Fig. 1C), Ni NPs (Fig. 1D), and Ni/rGO (Fig. 1E), Cu52@Ni48 CSNPs (Fig. 1F), and Cu53@Ni47 CSNPs/rGO NCs (Fig. 1G), and the samples (Fig. 1B–E) are clearly identified using the standard patterns of cubic-structured Ni (JCPDS No. 70-0989) and Cu (JCPDS No. 89-2838). Peaks of impurities are not detected, which indicate the high purity of the samples. As shown in Fig. 1F and G, the proposed Cu52@Ni48 CSNPs and Cu53@Ni47 CSNPs/rGO NCs exhibit patterns corresponding to Ni (JCPDS No. 70-0989) and Cu (JCPDS No. 89-2838), which indicate that the Cu and Ni are separated phase in NPs. Graphite oxide is known to exhibit a sharp peak at 2θ = 9.7°, which corresponds to the (001) reflection of graphene oxide, and can be reduced to graphene during the in situ solvothermal process.12a,b Given that Cu53@Ni47 CSNPs are formed on graphene surface, the diffraction peaks from the stack rGO sheets almost disappear in the XRD pattern (Fig. 1G) of Cu53@Ni47 CSNPs/rGO hybrid NCs.
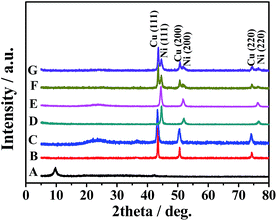 |
| Fig. 1 XRD patterns of the obtained GO (A), Cu NPs (B), Cu/rGO NCs (C), Ni NPs (D), Ni/rGO NCs (E), Cu52@Ni48 CSNPs (F), and Cu53@Ni47 CSNPs/rGO NCs (G). | |
Raman spectroscope is a powerful technique for investigating the structural and electronic properties of carbon compounds. The significant structural changes occurring during the chemical processing from GO to rGO are also reflected in Raman spectra.12 GO (Fig. S1, ESI†) displays two prominent peaks at 1347 and 1597 cm−1, corresponding to the D and G bands, respectively. The G band is usually assigned to the plane bond stretching of the C–C sp2 bond and D band is associated with structural defects and disorders.12a Here, the intensity ratio of D to G band (ID/IG) is used as a measure of structural defects and disorder in graphene structures,12 and the ID/IG ratio varies from 1.12 for graphene oxide to 1.79 for the Cu53@Ni47 CSNPs/rGO NCs. The reason for increasing of ID/IG ratio is reasonable because after chemical reduction of GO. We can also observe that the G band of Cu53@Ni47 CSNPs/rGO NCs moves from 1597 to about 1578 cm−1, close to the value of the pristine graphite.12 Furthermore, we use XPS to characterize detailed information about the as-obtained Cu53@Ni47 CSNPs/rGO NCs. As shown in Fig. S2 (ESI†), the peaks locate at 853.2 and 870.9 eV are assigned to the metallic Ni, and the peak locate at 932.1 eV is assigned to the metallic Cu. Above results indicate that GO in Cu53@Ni47 CSNPs/rGO NCs has been well deoxygenated and reduced, and which support the results from XRD pattern (Fig. 1G).
Morphologies and sizes of the final products were observed via scanning electron microscopy (SEM). Fig. 2 shows the SEM images of Cu NPs (Fig. 2A), Cu/rGO (Fig. 2C), Ni NPs (Fig. 2B), Ni/rGO (Fig. 2D), Cu52@Ni48 CSNPs (Fig. 2E), and Cu53@Ni47 CSNPs/rGO NCs (Fig. 2F), and irregular particle morphologies are obtained for each sample. From the SEM images, we obtain the size distributions of Cu NPs, Cu/rGO, Ni NPs, Ni/rGO, Cu52@Ni48 CSNPs, and Cu53@Ni47 CSNPs/rGO were 120–250, 30–130, 20–120, 40–100, 50–110, and 35–110 nm, respectively. Transmission electron microscopy (TEM) was applied to characterize the Cu53@Ni47 CSNPs/rGO NCs. As shown in Fig. 3A, the rGO sheets are decorated with irregular NPs, which is consistent with SEM results (Fig. 2F). High resolution TEM (HRTEM, Fig. 3B) image indicate that the NPs with high crystallinity are observed. From the interior to exterior of the NPs, the two different fringe spacing are 0.21 nm (Fig. 3C) and 0.20 nm (Fig. 3D), and which correspond to the interplanar spacing of {111} planes for fcc Cu and fcc Ni, respectively. Thereby, energy-dispersive X-ray spectroscopy (EDS, which attached to JEOL JEM-2100F TEM) mapping was further used to reveal different elements distribution in the Cu52@Ni48 CSNPs/rGO NCs. Fig. 3E–H show dark-field TEM image together with the elemental maps of Cu and Ni in the NCs, and we clearly see that Cu is the core and Ni is the shell. Namely, the as-prepared Cu–Ni NPs in the NCs have a core–shell structure, and which is in accordance with the XRD (Fig. 1G) and HRTEM (Fig. 3B–D) results. By using the same element-mapping technique, the samples of Cux@Ni100−x CSNPs/rGO NCs with different Cu/Ni molar ratios and Cu52@Ni48 CSNPs without rGO sheets were also identified (Fig. S3, ESI†), and all results reveal that those samples with core–shell structures are obtained. Furthermore, the chemical compositions (atomic percent) of CSNPs and NCs were determined by inductively-coupled plasma-atomic emission spectroscopy (ICP-AES). Based on the ICP-AES results (Table S1, ESI†), Cu/Ni molar ratios on Cu@Ni CSNPs and Cu@Ni CSNPs/rGO NCs are 52
:
48 and 53
:
47, and which are close to the initial molar ratio of Cu2+ to Ni2+. Meanwhile, the quality ratios of mCu/Ni (wt%) to mrGO sheets (wt%) in all Cux@Ni100−x CSNPs/rGO NCs were also evaluated by ICP-AES analysis (Table S1, ESI†).
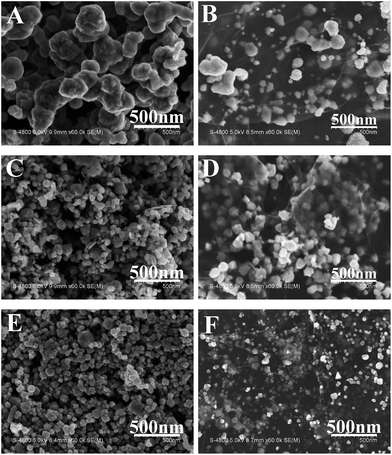 |
| Fig. 2 Typical SEM images of (A) Cu NPs, (B) Cu/rGO NCs, (C) Ni NPs, (D) Ni/rGO NCs, (E) Cu52@Ni48 CSNPs, and (F) Cu53@Ni47 CSNPs/rGO NCs were obtained by solvothermal method. | |
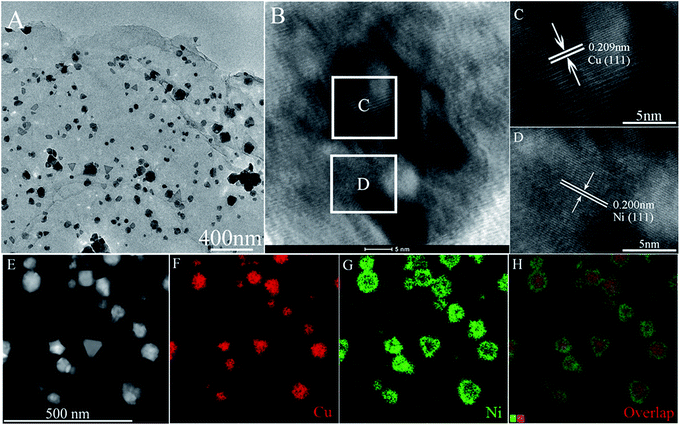 |
| Fig. 3 TEM image (A), HRTEM images (B–D), HAADF-STEM image (E), STEM-EDX maps in Cu Kα1 signals (F) and Ni Kα1 signals (G), and overall map spectrum (H) of Cu53@Ni47 CSNPs/rGO NCs. | |
Generally, the Cu@Ni or Ni@Cu CS structures are prepared by electrodeposition process.13 Here, a facile and one-pot solvothermal method is developed to prepare Cu@Ni NPs with CS structure in this paper. Based on the above results, the possible growth process for the synthesis of Cux@Ni100−x CSNPs is discussed as follows. First, the GO sheets have big specific surface area and abundant functional groups,9a,b,10 and the Cu2+ and Ni2+ can easy load on the surface of GO sheets by physical and chemical effects. And when the NaOH is added, Cu2+ and Ni2+ become the intermediate phase of Cu(OH)2 and Ni(OH)2, and then reduce to single metals by EG under alkaline condition. There are two key factors for controlling synthesis of Cu–Ni NPs with CS structures in this reaction system: enough potential difference in competitive redox reactions13a,14 and moderate reductant (when the strong reductant of hydrazine hydrate is used, CuNi alloy is obtained).12a According to the redox potentials (−0.222 V for Cu(OH)2/Cu and −0.72 V for Ni(OH)2/Ni), the Cu2+ is reduced into Cu0 at first,13a,14 and next the Ni2+ is reduced into Ni0 on the Cu surface. Therefore, Cu and Ni with CS structure NPs are formed. Simultaneously, the Cu–Ni NPs with CS structure (Fig. S3E1–E4, ESI†) are also obtained in the absence of GO sheets or other additives. This phenomenon reveals that Cu NPs not only act the load-island role, but also act interface catalyst for the next Ni nucleation on Cu NPs's surfaces. Thereby, the Cu–Ni NPs and Cu–Ni NPs in GO-based NCs with CS structures are successfully synthesized by this facile and one-pot solvothermal method.
Electrochemical behavior of Cu53@Ni47 CSNPs/rGO/Nafion/GCE
Normally, cyclic voltammetry is used to investigate electrochemical behavior of NCs. Here, the electrochemical properties of Cu53@Ni47 CSNPs/rGO NCs are also investigated by cyclic voltammetry in 0.1 M NaOH at different scan rates and the results are shown in Fig. 4A. When the scan rate is increased from 20 mV s−1 to 250 mV s−1 (Fig. 4B), both anodic and cathodic peak currents are found proportional to the scan rates with a linear equation of ipa = −3.487 − 0.016v (R = 0.9996, n = 10) and ipc = 2.418 + 0.009v (R = 0.9986, n = 10), respectively. These results indicate that the electrochemical kinetics of Cu53@Ni47 CSNPs/rGO/Nafion/GCE is surface controlled. The anodic peak potential shift more positively with increasing scan rates, whereas the cathodic potential shifted more negatively, which result in a larger peak-to-peak potential separation. These results are possibly due to NiO(OH) nucleation followed by increased active sites for both Ni3+ and Ni2+ species.15
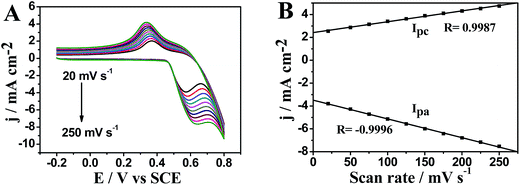 |
| Fig. 4 (A) CVs of Cu53@Ni47 CSNPs/rGO/Nafion/GCE in 0.1 M NaOH at different scan rates: 20, 50, 75, 100, 125, 150, 175, 200, 225, and 250 mV s−1. (B) Plot of peak current versus the potential scan rate. | |
Electrocatalytic oxidation of glucose on Cu53@Ni47 CSNPs/rGO/Nafion/GCE
A comparative study on the electrocatalytic performance toward glucose oxidation at different modified electrodes are carried out by cyclic voltammograms (CVs) in 0.1 M NaOH with and without 2 mM glucose at a scan rate of 50 mV s−1 (Fig. 5). As shown in Fig. 5A–F, the Cu/Nafion/GCE, Cu/rGO/Nafion/GCE, Ni/Nafion/GCE, Ni/rGO/Nafion/GCE, Cu52@Ni48 CSNPs/Nafion/GCE, and Cu53@Ni47 CSNPs/rGO/Nafion/GCE show an obvious oxidation peak toward glucose at +0.60 V, 0.55 V, +0.48 V, +0.47 V, +0.52 V, and +0.575 V, respectively. According to literatures,1a,3a,16 the oxidation potentials of Cu- or Ni-based electrodes may be corresponded to a redox couple of Cu(II)/Cu(III) or Ni(II)/Ni(III). It is accepted that the oxidation of glucose to gluconolactone is catalyzed by Cu(II)/Cu(III) and Ni(II)/Ni(III) redox couples: CuO(OH) + glucose → Cu(OH)2 + gluconolactone and NiO(OH) + glucose → Ni(OH)2 + gluconolactone, respectively. This suggests that compositions of Cu and Ni play a major role in the oxidation of glucose. The Cu53@Ni47 CSNPs/rGO/Nafion/GCE also shows higher electrocatalytic oxidation to glucose than that of Cu/Nafion/GCE or Ni/Nafion/GCE. This is explained by the synergistic effect between Cu53@Ni47 CSNPs with rGO sheets on the oxidation of glucose. In absence of glucose, the background current of Cu53@Ni47 CSNPs/rGO/GCE (Fig. 5F) is higher than that of other modified electrodes (Fig. 5A–E), which indicate that Cu53@Ni47 CSNPs/rGO/GCE has the highest active surface area.
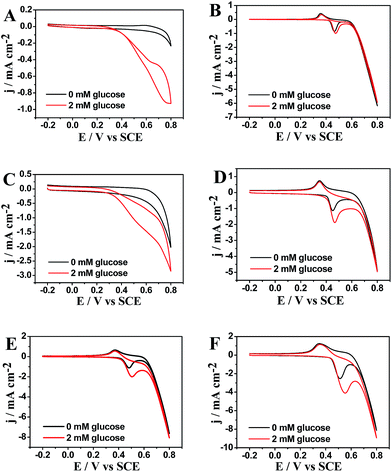 |
| Fig. 5 CVs of (A) Cu/Nafion/GCE, (B) Ni/Nafion/GCE, (C) Cu/rGO/Nafion/GCE, (D) Ni/rGO/Nafion/GCE, (E) Cu53@Ni47 CSNPs/Nafion/GCE, and (F) Cu53@Ni47 CSNPs/rGO/Nafion/GCE examined in 0.1 M NaOH with absence/presence of 2 mM glucose at a scan rate of 50 mV s−1. | |
Up addition of glucose, Cu/rGO/Nafion/GCE, Ni/rGO/Nafion/GCE and Cu53@Ni47 CSNPs/rGO/Nafion/GCE exhibit enhanced glucose electrocatalytic oxidation with 2.7-, 1.4- and 2.9-fold higher electrochemical responses than that of Cu/Nafion/GCE, Ni/Nafion/GCE and Cu52@Ni48 CSNPs/Nafion/GCE respectively and display the substantially negative shift in peak potential. This result indicates that the higher current response is partially attributed to the high conductivity of the graphene sheets. The graphene-based modified electrode provide a more sterically hindered structure and a larger conducting surface area to support more active species.9,17 In addition, the Cu53@Ni47 CSNPs/rGO/Nafion/GCE shows a uniquely high net current that is approximately 2.9 times greater than those in other modified electrodes. The much higher current response of the Cu53@Ni47 CSNPs/rGO/Nafion/GCE may be due to its uniform and compact structure. This rGO sheets provide a more conductive surface area to load Cu53@Ni47 CSNPs active species, resulting in a uniform, compact and active hybrid NCs. Thus, the NCs show specific activity toward glucose oxidation.
Bimetal molar ratio in NCs is a major parameter for active materials.3e,6,11 Effects of Cu to Ni molar ratio on the catalytic performance of Cux@Ni100−x CSNPs/rGO/Nafion/GCE are also investigated. Table S2 (ESI†) shows the net current estimate on the electrocatalytic activity of the Cux@Ni100−x CSNPs/rGO/Nafion/GCE on glucose oxidation in the absence or presence of 0.5 mM glucose. The highest net current value is obtained from the 53
:
47 of Cu to Ni molar ratio, which suggests that the composite exhibits high activity toward glucose oxidation because of the synergistic effect of Cu53@Ni47 CS structure with rGO sheets. Moreover, this result also indicates that 53
:
47 is the optimum Cu to Ni molar ratio for the synthesis of active Cu53@Ni47 CSNPs/rGO NCs.
To improve the sensing performance of the electrode, the effect of applied potentials on sensor response is systematically investigated (Fig. S4, ESI†). Current–time curves are recorded at different applied potentials (+0.525 V to +0.625 V) with successive addition of 0.5 mM glucose to 0.1 M NaOH. The results illustrate that the maximum response is also observed at +0.575 V applied potential, and which is consistent with previous test (Fig. 5F). So, the +0.575 V is chosen as the optimal potential for amperometric glucose sensing due to this potential is strongly related to the oxidation peaks of Cu(II)/Cu(III) and Ni(II)/Ni(III).6a–c,15,16
Amperometric response of the Cu53@Ni47 CSNPs/rGO/Nafion/GCE towards glucose
The amperometric response of Cu53@Ni47 CSNPs/rGO/Nafion/GCE upon successive addition of different concentration of glucose is further evaluated at the optimum experimental conditions. The modified electrode responds quickly to the change of glucose concentration and reaches about 95% of the steady-state current is no more than 3 s (inset in Fig. 6A) indicating an extraordinarily fast rapid and sensitive response to glucose. The calibration curve for the modified electrode is shown in Fig. 6B. The current response of the sensor exhibits a linear dependence on glucose concentration from 1 μM to 4.1 mM (i = 0.08 + 0.78c, R = 0.9969). The detection limit of glucose using a Cu53@Ni47 CSNPs/rGO/GCE is found to be 0.5 μM (S/N = 3) with the sensitivity of 780 μA mM−1 cm−2. For comparison, the performances of other non-enzymatic glucose sensors based on reported in literatures have been listed in Table 1. It can be concluded that our sensor exhibits a very faster response, a lower detection limit and a wider linear range. These are attributed to the following reasons: (i) synergetic effect from bimetallic compositions.6,11,19 Based on the above results, the electrocatalytic glucose oxidation ability decreased when the Cu/Ni molar ratio is too smaller or too higher, and the optimized Cu/Ni molar ratio resulting the high activity towards glucose oxidation. Thereby, the Cu53@Ni47 CSNPs/rGO/GCE exhibits high activity toward glucose oxidation. (ii) Synergetic effect from the specific CS structures.14b,19 In the previous reports, the core–shell structure as a typical structure there are three major synergistic interactions (such as ligand effect, ensemble effects, and geometric effects) between the core and shell which resulting the enhanced electrocatalytic activity. Furthermore, the thickness of the Ni coating layer is importance to oxidation resistance. The molar ratio of Cu to Ni is 53
:
47 reveals a highest electrocatalytic activity for Cu53@Ni47 CSNPs/rGO NCs. (iii) Synergetic effect between the NPs and support materials.6,11,17,19 Cu@Ni CSNPs decorating the reduced graphene oxide sheets on the electrode surface, which significantly increases the number of electrocatalytic active areas and promotes electron transfer in the oxidation of glucose.
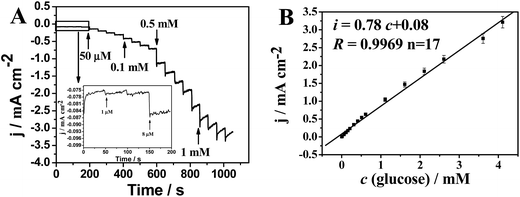 |
| Fig. 6 (A) Current–time responses at +0.575 V with an increasing glucose concentration per 50 s for the Cu53@Ni47 CSNPs/rGO/Nafion/GCE. (B) The linear relationship between the catalytic current and glucose concentration. | |
Table 1 Comparison of the performances of our Cu@Ni CSNPs/rGO sensor with other published non-enzymatic glucose sensors
Electrode material |
Linear range (mM) |
Detection limit (μM) |
Reference |
Cu53@Ni47 CSNPs/rGO NCs |
0.001–4.1 |
0.5 |
This work |
PdCu/GE |
1–18 |
20 |
6a |
ITO/Ni/Cu |
0.001–2 |
0.23 |
6b |
Ni–Cu/TiO2NTs |
0.01–3.2 |
5 |
6c |
Pt/Ni NWAs |
0–2 |
1.5 |
6f |
Ni–Co NSs/rGO |
0.01–2.65 |
3.79 |
11a |
Cu–Co NSs/rGO–CHIT |
0.015–6.95 |
10 |
11b |
Ni NPs/SMWNTs |
0.001–1 |
0.5 |
16 |
rGO–Ni NPs |
0.002–2.1 |
0.1 |
18a |
BDD/Ni |
0.005–10 |
2.7 |
18b |
Ni–CNF |
1–10 |
— |
18c |
Cu NPs–GR |
0.01–1.2 |
3.4 |
18d |
Cu/PMo12-GR |
0.0001–1.0 |
0.03 |
18e |
Reproducibility, stability and anti-interference property of the Cu53@Ni47 CSNPs/rGO/Nafion/GCE
The reproducibility of Cu53@Ni47 CSNPs/rGO/Nafion/GCE is examined by measuring its amperometric response to 0.5 mM glucose. A 3.4% relative standard deviation is obtained from 10 successive amperometric determinations, which indicates a relatively good reproducibility. The stability of the modified electrode is evaluated by storing it for 72 h at 4 °C. The response current is maintained at 93.0% of its initial response, which demonstrates a good stability, whereas Cu53@Ni47 CSNPs/rGO/Nafion/GCE retains 89% of its initial current value, which clearly confirms the importance of the graphene scaffold in enhancing the stability of Cu53@Ni47 CSNPs.
The anti-interference property and stability of Cu53@Ni47 CSNPs/rGO/Nafion/GCE are crucial in developing nonenzymatic electrochemical biosensors. Given that chemical species such as uric acid (UA), dopamine (DA), ascorbic acid (AA), and NaCl that easily oxidize are always present with glucose in human blood, their electrochemical response are also examined using Cu53@Ni47 CSNPs/rGO/Nafion/GCE. The normal physiological glucose level is considered to be considerably higher than the above analytes.20 In the present study, the interference experiment is conducted by adding 0.1 mM interfering species into 0.5 mM glucose in 0.1 M NaOH. As shown in Fig. S5 (ESI†), there is no obvious current response observed with the addition of 0.1 mM DA, 0.1 mM UA and 0.1 mM AA. Moreover, the addition of NaCl does not show any effect on the current of glucose oxidation, suggesting high chloride tolerance of Cu53@Ni47 CSNPs/rGO/Nafion/GCE. Furthermore, the response of other sugars, such as 0.5 mM glucose, 0.1 mM sucrose, 0.1 mM maltose, 0.1 mM fructose, 0.1 mM D-galactose, 0.5 mM glucose compares to that of glucose, is also evaluated as shown in Fig. S6 (ESI†). Current responses of the corresponding interfering species are also very weak, and demonstrate that the low level of saccharides will not affect the detection of glucose by this excellent sensor. Overall, it can be concluded that the small amounts of interfering species have a weakly influence on the glucose response. In other words, the Cu53@Ni47 CSNPs/rGO/Nafion/GCE shows high selectivity for glucose detection.
Simulative sample analysis
However, despite the complexity of the serum components, only glucose, AA, and UA are electrochemically active. Therefore, a simulative serum sample containing 1 mM glucose, 0.08 mM AA, and 0.04 mM UA is prepared according to the approximate concentrations of the three components in an actual 20% serum sample.21 Glucose is evaluated in the simulated serum by using Cu53@Ni47 CSNPs/rGO/Nafion/GCE; the results are listed in Table 2. The results show that other electroactive components in serum do not significantly interfere with the glucose determination. Hence, the Cu53@Ni47 CSNPs/rGO/Nafion/GCE can be potentially used for routine blood glucose testing.
Table 2 Determination of glucose concentration in the simulative sample (n = 3)
Sample |
Add (mM) |
Found (mM) |
Recovery (%) |
R.S.D. (%, n = 3) |
1 |
0.5 |
0.476 |
95.2 |
4.12 |
2 |
0.6 |
0.627 |
104.3 |
4.69 |
4 Conclusions
In summary, the Cux@Ni100−x (x = 70, 63, 53, 38, and 20) CSNPs/rGO NCs are synthesized by a facile and one-pot solvothermal method. We demonstrate that Cu53@Ni47 CSNPs/rGO/GCE shows the highest electrochemical response towards glucose oxidation due to the three-in-one synergistic effects from Cu/Ni bimetallic system, Cu@Ni core–shell structure, and interactions from the bimetallic CSNPs and reduced graphene oxide sheets. Cu53@Ni47 CSNPs/rGO/GCE reveals a low cost, high sensitivity, and good reproducibility in glucose determination. A wide linear range of 0.001 to 4.1 mM, a low detection limit of 0.5 μM (S/N = 3), a high sensitivity of 780 μA mM−1 cm−2, and a fast response time (3 s) are obtained. Furthermore, the oxidable species shows insignificant interference in determination of glucose, and which indicates that Cu53@Ni47 CSNPs/rGO NCs-based sensor has potential applications for the determination of glucose in biological fluids.
Acknowledgements
This work is financially supported by the National Natural Science Foundation of China (Nos 21501004, 21071005, 21271006, 21201008), and the Research Culture Funds of Anhui Normal University (No. 2014xmpy13), and the Innovation Funds of Anhui Normal University (No. 2014cxjj13).
References
-
(a) G. F. Wang, X. P. He, L. L. Wang, A. X. Gu, Y. Huang, B. Fang, B. Y. Geng and X. J. Zhang, Microchim. Acta, 2013, 180, 161 CrossRef CAS;
(b) A. Heller and B. Feldman, Chem. Rev., 2008, 108, 2482 CrossRef CAS PubMed;
(c) J. Wang, Chem. Rev., 2008, 108, 814 CrossRef CAS PubMed;
(d) C. Chen, Q. J. Xie, D. W. Yang, H. L. Xiao, Y. C. Fu, Y. M. Tan and S. Z. Yao, RSC Adv., 2013, 3, 4473 RSC;
(e) X. Niu, X. Li, J. Pan, Y. He, F. Qiu and Y. Yan, RSC Adv., 2016, 6, 84893 RSC.
-
(a) L. Su, X. Yu, W. Qin, W. Dong, C. Wu, Y. Zhang, G. Mao and S. Feng, J. Mater. Chem. B, 2017, 5, 116 RSC;
(b) P. Si, Y. J. Huang, T. H. Wang and J. M. Ma, RSC Adv., 2013, 3, 3487 RSC;
(c) L. Pu, M. Baig and V. Maheshwari, Anal. Bioanal. Chem., 2016, 408, 2697 CrossRef CAS PubMed.
-
(a) Y. Yang, Y. Wang, X. Bao and H. Li, J. Electroanal. Chem., 2016, 775, 163 CrossRef CAS;
(b) L. Wang, X. Lu, C. Wen, Y. Xie, L. Miao, S. Chen, H. Li, P. Li and Y. Song, J. Mater. Chem. A, 2015, 3, 608 RSC;
(c) S.-J. Li, N. Xia, X.-L. Lv, M.-M. Zhao, B.-Q. Yuan and H. Pang, Sens. Actuators, B, 2014, 190, 809 CrossRef CAS;
(d) A. Rengaraj, Y. Haldorai, C. H. Kwak, S. Ahn, K.-J. Jeon, S. H. Park, Y.-K. Han and Y. S. Huh, J. Mater. Chem. B, 2015, 3, 6301 RSC;
(e) W. Yan, D. Wang and G. G. Botte, Electrochim. Acta, 2012, 61, 25 CrossRef CAS;
(f) B. Yuan, C. Xu, D. Deng, Y. Xing, L. Liu, H. Pang and D. Zhang, Electrochim. Acta, 2013, 88, 708 CrossRef CAS;
(g) Z. Liu, Y. Guo and C. Dong, Talanta, 2015, 137, 87 CrossRef CAS PubMed.
-
(a) Y. Li, J. J. Fu, R. S. Chen, M. Huang, B. Gao, K. F. Huo, L. Wang and P. K. Chu, Sens. Actuators, B, 2014, 192, 474 CrossRef CAS;
(b) J. Zhao, L. Wei, C. Peng, Y. Su, Z. Yang, L. Zhang, H. Wei and Y. Zhang, Biosens. Bioelectron., 2013, 47, 86 CrossRef CAS PubMed;
(c) A. A. Ensafi, M. Jafari-Asl, N. Dorostkar, M. Ghiaci, M. V. Martínez-Huert and J. L. G. Fierro, J. Mater. Chem. B, 2014, 2, 706 RSC;
(d) X. Lu, Y. Ye, Y. Xie, Y. Song, S. Chen, P. Li, L. Chen and L. Wang, Anal. Methods, 2014, 6, 4643 RSC;
(e) L. Liu, Y. Chen, H. Lv, G. Wang, X. Hu and C. Wang, J. Solid State Electrochem., 2015, 19, 731 CrossRef CAS;
(f) Q. Wang, Q. Wang, M. Li, S. Szunerits and R. Boukherroub, RSC Adv., 2015, 5, 15861 RSC;
(g) J. Zheng, W. Zhang, Z. Lin, C. Wei, W. Yang, P. Dong, Y. Yan and S. Hu, J. Mater. Chem. B, 2016, 4, 1247 RSC.
- C. M. Welch and R. G. Compton, Anal. Bioanal. Chem., 2006, 384, 601 CrossRef CAS PubMed.
-
(a) M. Yuan, A. P. Liu, M. Zhao, W. J. Dong, T. Y. Zhao, J. J. Wang and W. H. Tang, Sens. Actuators, B, 2014, 190, 707 CrossRef CAS;
(b) P. Salazar, V. Rico and A. R. González-Elipe, Sens. Actuators, B, 2016, 226, 436 CrossRef CAS;
(c) X. L. Li, J. Y. Yao, F. L. Liu, H. C. He, M. Zhou, N. Mao, P. Xiao and Y. H. Zhang, Sens. Actuators, B, 2013, 181, 501 CrossRef CAS;
(d) K. C. Lin, Y. C. Lin and S. M. Chen, Electrochim. Acta, 2013, 96, 164 CrossRef CAS;
(e) H.-B. Noh, K.-S. Lee, P. Chandra, M.-S. Won and Y.-B. Shim, Electrochim. Acta, 2012, 61, 36 CrossRef CAS;
(f) S. S. Mahshid, S. Mahshid, A. Dolati, M. Ghorbani, L. Yang, S. Luo and Q. Cai, Electrochim. Acta, 2011, 58, 551 CrossRef CAS.
-
(a) M. B. Gawande, A. Goswami, T. Asefa, H. Guo, A. V. Biradar, D.-L. Peng, R. Zboril and R. S. Varma, Chem. Soc. Rev., 2015, 44, 7540 RSC;
(b) Y. Holade, A. Lehoux, H. Remita, K. B. Kokoh and T. W. Napporn, J. Phys. Chem. C, 2015, 119, 27529 CrossRef CAS;
(c) J. Wang, L. Xu, Y. Lu, K. Sheng, W. Liu, C. Chen, Y. Li, B. Dong and H. Song, Anal. Chem., 2016, 88, 12346 CrossRef CAS PubMed.
-
(a) C. Zhao, P. Gai, R. Song, J. Zhang and J.-J. Zhu, Anal. Methods, 2015, 7, 4640 RSC;
(b) G.-H. Yang, Y.-H. Zhou, J.-J. Wu, J.-T. Cao, L.-L. Li, H.-Y. Liu and J.-J. Zhu, RSC Adv., 2013, 3, 22597 RSC;
(c) G. Yang, Y. Li, R. K. Rana and J.-J. Zhu, J. Mater. Chem. A, 2013, 1, 1754 RSC;
(d) Y. Chen, Y. Li, D. Sun, D. Tian, J. Zhang and J.-J. Zhu, J. Mater. Chem., 2011, 21, 7604 RSC;
(e) J. J. Gooding, Electrochim. Acta, 2005, 50, 3049 CrossRef CAS;
(f) H. Sun, J. Ren and X. Qu, Acc. Chem. Res., 2016, 49, 461 CrossRef CAS PubMed;
(g) K. Qu, L. Wu, J. Ren and X. Qu, ACS Appl. Mater. Interfaces, 2012, 4, 5001 CrossRef CAS PubMed;
(h) M. Li, Y. Guan, C. Ding, Z. Chen, J. Ren and X. Qu, J. Mater. Chem. B, 2016, 4, 4072 RSC;
(i) L. Wu, L. Feng, J. Ren and X. Qu, Biosens. Bioelectron., 2012, 34, 57 CrossRef CAS PubMed.
-
(a) D. Chen, L. Tang and J. Li, Chem. Soc. Rev., 2010, 39, 3157 RSC;
(b) D. Chen, H. Feng and J. Li, Chem. Rev., 2012, 112, 6027 CrossRef CAS PubMed;
(c) R. Que, Q. Shao, Q. Li, M. Shao, S. Cai, S. Wang and S. T. Lee, Angew. Chem., Int. Ed., 2012, 51, 5418 CrossRef CAS PubMed;
(d) A. Rengaraj, Y. Haldorai, C. H. Kwak, S. Ahn, K.-J. Jeon, S. H. Park, Y.-K. Han and Y. S. Huh, J. Mater. Chem. B, 2015, 3, 6301 RSC;
(e) S. Pattnaik, K. Swain and Z. Lin, J. Mater. Chem. B, 2016, 4, 7813 RSC;
(f) B. Thirumalraj, C. Rajkumar, S.-M. Chen and P. Barathi, J. Mater. Chem. B, 2016, 4, 6335 RSC;
(g) S.-J. Li, Y.-F. Shi, L. Liu, L.-X. Song, H. Pang and J.-M. Du, Electrochim. Acta, 2012, 85, 628 CrossRef CAS;
(h) S. Zhuo, M. Shao and S.-T. Lee, ACS Nano, 2012, 6, 1059 CrossRef CAS PubMed.
-
(a) S. Bong, Y. R. Kim, I. Kim, S. Woo, S. Uhm, J. Lee and H. Kim, Electrochem. Commun., 2010, 12, 129 CrossRef CAS;
(b) X. W. Liu, J. J. Mao, P. D. Liu and X. W. Wei, Carbon, 2011, 49, 477 CrossRef CAS;
(c) X.-W. Liu, Z.-J. Yao, Y.-F. Wang and X.-W. Wei, Colloids Surf., B, 2010, 81, 508 CrossRef CAS PubMed.
-
(a) L. Wang, X. P. Lu, Y. J. Ye, L. L. Sun and Y. H. Song, Electrochim. Acta, 2013, 114, 484 CrossRef CAS;
(b) L. Wang, Y. L. Zheng, X. P. Lu, Z. Li, L. L. Sun and Y. H. Song, Sens. Actuators, B, 2014, 195, 1 CrossRef CAS.
-
(a) J. Yang, X. Shen, Z. Ji, H. Zhou, G. Zhu and K. Chen, Appl. Surf. Sci., 2014, 316, 575 CrossRef CAS;
(b) J. Yang, X. Shen, Z. Ji, H. Zhou, G. Zhu and K. Chen, Ceram. Int., 2015, 41, 4056 CrossRef CAS;
(c) Z. Zafar, Z. H. Ni, X. Wu, Z. X. Shi, H. Y. Nan, J. Bai and L. T. Sun, Carbon, 2013, 61, 57 CrossRef CAS;
(d) S. Stankovich, D. A. Dikin, R. D. Piner, K. A. Kohlhaas, A. Kleinhammes, Y. Jia, Y. Wu, S. T. Nguyen and R. S. Ruoff, Carbon, 2007, 45, 1558 CrossRef CAS;
(e) B. Li, H. Cao, J. Yin, Y. A. Wu and J. H. Warner, J. Mater. Chem., 2012, 22, 1876 RSC.
-
(a) Z. Liu, L. Guo, C.-L. Chien and P. C. Searson, J. Electrochem. Soc., 2008, 155, D569 CrossRef CAS;
(b) Z. Liu, G. Xia, F. Zhu, S. Kim, N. Markovic, C.-L. Chien and P. C. Searson, J. Appl. Phys., 2008, 103, 064313 CrossRef;
(c) Z. Liu, D. Elbert, C.-L. Chien and P. C. Searson, Nano Lett., 2008, 8, 2166 CrossRef CAS PubMed;
(d) M. Hennes, J. Buchwald and S. G. Mayr, CrystEngComm, 2012, 14, 7633 RSC;
(e) M. Hennes, J. Buchwald, U. Ross, A. Lotnyk and S. G. Mayr, Phys. Rev. B: Condens. Matter Mater. Phys., 2015, 91, 245401 CrossRef.
-
(a) T. Yamauchi, Y. Tsukahara, T. Sakata, H. Mori, T. Yanagida, T. Kawai and Y. Wada, Nanoscale, 2010, 2, 515 RSC;
(b) J. Chen, J. Chen, Y. Li, W. Zhou, X. Feng, Q. Huang, J.-G. Zheng, R. Liu, Y. Ma and W. Huang, Nanoscale, 2015, 7, 16874 RSC;
(c) J. Feng and C. P. Zhang, J. Colloid Interface Sci., 2006, 293, 414 CrossRef CAS PubMed;
(d) S. Zhang and H. C. Zeng, Chem. Mater., 2009, 21, 871 CrossRef CAS.
-
(a) M. Jafarian, M. G. Mahjani, H. Heli, F. Gobal and M. Heydarpoor, Electrochem. Commun., 2003, 5, 184 CrossRef CAS;
(b) B. Ballarin, R. Seeber, D. Tonelli and A. Vaccari, J. Electroanal. Chem., 1999, 463, 123 CrossRef CAS.
- H. G. Nie, Z. Yao, X. M. Zhou, Z. Yang and S. M. Huang, Biosens. Bioelectron., 2011, 30, 28 CrossRef CAS PubMed.
-
(a) V. Georgakilas, M. Otyepka, A. B. Bourlinos, V. Chandra, N. Kim, K. C. Kemp, P. Hobza, R. Zboril and K. S. Kim, Chem. Rev., 2012, 112, 6156 CrossRef CAS PubMed;
(b) F. Xiao, J. Song, H. Gao, X. Zan, R. Xu and H. Duan, ACS Nano, 2012, 6, 100 CrossRef CAS PubMed.
-
(a) Y. Zhang, X. Xiao, Y. Sun, Y. Shi, H. Dai, P. Ni, J. Hu, Z. Li, Y. Song and L. Wang, Electroanalysis, 2013, 25, 959 CrossRef CAS;
(b) Y. Liu, H. Teng, H. Hou and T. You, Biosens. Bioelectron., 2009, 24, 3329 CrossRef CAS PubMed;
(c) K. E. Toghill, L. Xiao, M. A. Phillips and R. G. Compton, Sens. Actuators, B, 2010, 147, 642 CrossRef CAS;
(d) P. Salazar, V. Rico, R. Rodríguez-Amaro, J. P. Espinós and A. R. González-Elipe, Electrochim. Acta, 2015, 169, 195 CrossRef CAS;
(e) W.-Y. Jeon, Y.-B. Choi and H.-H. Kim, Sensors, 2015, 15, 31083 CrossRef CAS PubMed.
- K. D. Gilroy, A. Ruditskiy, H.-C. Peng, D. Qin and Y. Xia, Chem. Rev., 2016, 116, 10414 CrossRef CAS PubMed.
- J. P. Wang, D. F. Thomas and A. C. Chen, Anal. Chem., 2008, 80, 997 CrossRef CAS PubMed.
- Y. Q. Yang, C. L. Yi, J. Luo, R. Liu, J. K. Liu, J. Q. Jiang and X. Y. Liu, Biosens. Bioelectron., 2011, 26, 2607 CrossRef CAS PubMed.
Footnote |
† Electronic supplementary information (ESI) available: Fig. S1–S5; Tables S1 and S2. See DOI: 10.1039/c7ra00910k |
|
This journal is © The Royal Society of Chemistry 2017 |
Click here to see how this site uses Cookies. View our privacy policy here.