DOI:
10.1039/C7RA00678K
(Paper)
RSC Adv., 2017,
7, 18803-18814
Stability behaviour of antiretroviral drugs and their combinations. 6: evidence of formation of potentially toxic degradation products of zidovudine under hydrolytic and photolytic conditions†
Received
16th January 2017
, Accepted 3rd March 2017
First published on 28th March 2017
Abstract
This study explored the comprehensive degradation behaviour of zidovudine (ZDV) under solution and solid stress conditions. In total, nine degradation products were detected by high performance liquid chromatography (HPLC). The same were tentatively characterized with the help of high resolution and multistage mass spectrometry. Among them, five degradation products were also enriched and isolated with the help of semi-preparative HPLC and subjected to 1D and 2D nuclear magnetic resonance and/or infrared spectrometric studies to confirm their structures. The characterization of all the degradation products helped in outlining the comprehensive degradation pathway of ZDV. A significant finding was the formation of 3′-amino-3′-deoxythymidine (AMT) upon base hydrolysis as well as photolysis of the drug. This product is a known catabolite of the drug with a high degree of toxicity. Also, a few other degradation products formed during the study were predicted to have potential toxicity.
Introduction
In this sixth part of the series of studies on stability behaviour of antiretroviral drugs and their combinations,1–5 we re-examined the stress degradation behaviour of zidovudine (ZDV, formerly AZT, 1-[(2R,4S,5S)-4-azido-5-(hydroxymethyl)tetrahydrofuran-2-yl]-5-methylpyrimidine-2,4(1H,3H)-dione). The drug is a first nucleoside reverse transcriptase inhibitor, which was approved by the United States Food and Drug Administration (USFDA) in 1987 for the treatment of acquired immunodeficiency syndrome (AIDS).6 In our previous reports,7,8 we identified 5-methylpyrimidine-2,4(1H,3H)-dione (thymine) as the major degradation product of the drug under hydrolytic and photolytic conditions. In a recent paper, Devrukhakar et al.9 identified 1-5-(hydromethyl)teterahydrofuran-2-yl-5-methylpyrimidine-2,4(1H,3H)-dione, along with thymine as the degradation products of ZDV under acid conditions. Multiple other publications exist in the literature on stability of ZDV,10–13 but there is no report outlining comprehensive degradation chemistry of the drug.
In the present systematic study, we detected nine degradation products of ZDV under hydrolytic and photolytic forced degradation conditions. While four were observed in stressed neutral, acidic and basic solutions, exposure of the drug to a combination of UV and visible light resulted in total seven products, with two being common. Additionally, a stress study in the solid state resulted in six degradation products, which were same to those formed during solution state stress studies. All the nine were subjected to hyphenated liquid chromatography-mass spectrometry (LC-MS) to assign the structures. At least five of them were isolated and subjected to 1D and 2D nuclear magnetic resonance (NMR) and/or infrared (IR) spectroscopy for further confirmation of their structures. The results and observations allowed outlining of degradation pathway of the drug under the investigated stress conditions.
A significant finding was the formation of 3′-amino-3′-deoxythymidine (AMT) upon base hydrolysis and photolysis of ZDV. This degradation product is a known catabolite of the drug and is reported to exhibit high degree of toxicity towards human hemopoietic cells.14–16 The toxicity of all other characterised degradation products was also predicted using TOPKAT software.
In recent years, intensive global efforts have led to increased number (∼17 million) of people receiving life-long HIV treatment.17 Owing to this reason, the presence of HIV drugs and their related substances in environmental matrices has come under recent focus.18 Hence the results of this study assumingly are important even with respect to the fate of ZDV in the environment.
Experimental
Chemicals and reagents
Pure ZDV was obtained as a gratis sample from M/S Aurobindo Pharma Ltd. (Hyderabad, India). Hydrochloric acid (HCl) and sodium hydroxide (NaOH) were purchased from LOBA Chemie Pvt. Ltd. (Mumbai, India) and Ranbaxy Laboratories (S.A.S. Nagar, India), respectively. HPLC grade methanol (CH3OH) was purchased from Aldrich (St. Louis, MO, USA). Deuterated methanol (CD3OD) of 99.8% atom D purity was also purchased from Aldrich. Buffer salts and all other chemicals were of analytical reagent grade. Ultra pure water (H2O) was obtained from ELGA water purification unit (Bucks, England).
Apparatus and equipments
Accelerated stability studies were carried out in humidity (KBF720, WTC Binder, Tuttlingen, Germany) and photostability (KBWF240, WTC Binder, Tuttlingen, Germany) chambers set at 40 °C/75% RH and 25 °C, respectively. The photostability chamber was equipped with an illumination bank on inside top consisting of a combination of three UV (OSRAM L18 W/73) and three white fluorescent (Philips, Trulite) lamps. Lux meter (model ELM 201, Escorp, New Delhi, India) and near UV radiometer (model 206, PRC Krochmann GmbH, Berlin, Germany) were used to measure visible illumination and near UV energy, respectively.
HPLC studies were carried out on LC-2010C HT liquid chromatograph (Shimadzu, Kyoto, Japan), which was equipped with a SPD-M20A prominence diode array detector. Pursuit XRs LC column (5 μ, C18, 250 × 4.6 mm, Varian Inc., Lake Forest, CA, USA) was used for analytical studies, while Kingsorb column (5 μ, C18, 250 × 10.0 mm, Phenomenex, Torrance, CA, USA) was used for isolation of the degradation products.
Liquid chromatography-high resolution mass spectrometry (LC-HRMS) studies were carried out using LC-ESI-Q-TOF-MS, in which LC part consisted of 1100 series HPLC (Agilent Technologies, Waldbronn, Germany) comprising of an on-line degasser (G1379A), binary pump (G1312A), auto injector (G1313A), column oven (G1316A) and a PDA detector (G1315B). The HRMS system consisted of MicrOTOF-Q spectrometer (Bruker Daltonics, Bremen, Germany). System control and data acquisition were done by Hystar software (version 3.1) from the same source. The calibration solution used was 5 mM sodium formate. Liquid chromatography-multistage mass spectrometry (LC-MSn) data were acquired on Accela LC that was connected to a linear trap quadrupole mass spectrometer LTQ-XL-MS 2.5.0 (Thermo, San Jose, CA, USA). The mass spectra were acquired and processed using Xcalibur software (version 2.0.7 SP1). NMR studies were performed on JNM-ECA 500 MHz NMR spectrometer (JEOL, Tokyo, Japan). The data were processed by Delta v5.0.4.4 software. IR spectra of the drug and the selected degradation products were recorded on Spectrum Two IR spectrometer (Perkin Elmer, Waltham, MA, USA).
pH/Ion analyzer model PB-11 from Sartorius (Goettingen, Germany) was used to check pH of all the solutions. Other equipments used were sonicator (3210, Branson Ultrasonics Corporation, Danbury, CT, USA), precision analytical balance (AG 135, Mettler Toledo, Schwerzenbach, Switzerland) and auto pipettes (Eppendorf, Hamburg, Germany).
Solution stress degradation studies
The drug was subjected to hydrolytic, oxidative and photolytic solution stress studies according to the protocol described in our previous publications.19,20 Final drug concentration in all the stress solutions was 2 mg ml−1. Hydrolytic studies were carried out in water (neutral), 1 N HCl and 1 N NaOH solutions. The temperature of hydrolytic reactions was 80 °C. Oxidative stress studies were conducted in 15% H2O2 solution and in the presence of 100 mM% AIBN (200 mM% AIBN solution in CH3CN
:
CH3OH (80
:
20) diluted with equal volume of drug stock solution in water (4 mg ml−1)). Photolytic study was conducted on the drug solution prepared in water. The same was exposed to 1.2 million lx h cool white and 200 W h m−2 UV-A radiations. Samples were neutralized with acid/alkali and/or diluted two times with water before injecting into HPLC. The HPLC method involved a mobile phase composed of methanol (A) and 10 mM ammonium formate (pH 3.75) (B) that was run in a gradient mode (Tmin/%B; T0/95; T5/95; T50/10; T55/95; T62/95). The column oven temperature was 30 °C. The detection wavelength, flow rate and injection volume were 254 nm, 1 ml min−1 and 10 μl, respectively.
Solid state stress studies
Four sets were prepared for solid state stress investigations. The first and second sets were further divided into three subsets, i.e., ZDV; ZDV
:
H3BO3 (1
:
2), and ZDV
:
Na2CO3 (1
:
2). The first set (all three subsets) contained the drug and stressors along with 50 μl H2O in closed vials, and was charged to accelerated stability test chamber for 1 month. The second set (three subsets) was exposed to accelerated stability condition of 40 °C/75% RH for 3 months in an open container. The third set containing drug alone in cotton-plugged ampoules was kept at 60 °C for 1 month. The fourth set comprised of the drug in open Petri dish, which was charged to photostability chamber for a sufficient duration so as to provide a total dose of 6 million lx h cool white light and 1193 W h m−2 near-UV. After completion of the study, all the samples were withdrawn and diluted in water to a final concentration of 2 mg ml−1. The samples were monitored for chemical changes by HPLC using the same method that was employed for evaluation of the solution state stressed samples, except that the injection volume was halved to 5 μl.
LC-HRMS and LC-MSn studies
The LC method used for MS studies was similar to the HPLC method employed for analyses of the stressed samples. However, to prevent condensation in the ionization source, the solvent flow into MS system was reduced from 1 ml min−1 to 200 μl min−1, using a diversion valve. The HRMS and MSn instrument parameters were optimized suitably to obtain best intensity of the molecular ions (Table S1†). LC-MSn studies were conducted by defining the segments for individual degradation products.
NMR studies on the drug and selected degradation products
The drug itself was initially subjected to 1D and 2D NMR studies in CD3OD to compare its spectra with those of the degradation products, in order to characterize the latter. Five out of the nine degradation products were enriched and isolated using semi-preparative HPLC by employing suitable HPLC methods. The mobile phase used for isolation contained methanol and acidified water. In each case, the collected HPLC fraction was dried on a rotary evaporator and the residue was subjected to 1D and 2D NMR studies after preparing solution in CD3OD. Pre-saturation method was used to suppress the signal due to water.
IR studies on the drug and isolated degradation products
The IR spectra of the drug and certain isolated degradation products were recorded in KBr.
In silico toxicity prediction
TOPKAT (TOxicity Prediction by Komputer Assisted Technology, Discovery Studio 2.5, Accelrys, Inc., San Diego, CA, USA) was employed to predict potential toxicity of the degradation products.21 The software employs statistically robust and cross-validated quantitative structure toxicity relationship (QSTR) models to predict toxicological end points in term of probability values. To develop the models, it uses a range of two-dimensional molecular, electronic and spatial descriptors. The program applies the patented optimal predictive space (OPS) validation method to estimate the assurance in the prediction. Probability values from 0.0 to 0.30 are considered as low probabilities for any toxicological end point, whereas those greater than 0.70 are considered as high probabilities.22 The values from 0.3 to 0.7 fall in an in-determinate zone.
Results and discussion
Solution state degradation behaviour of the drug
In total nine degradation products (Z1–Z9) were detected under various solution stress conditions (Fig. 1a). In neutral hydrolytic condition (80 °C, 48 h), only one degradation product Z3 was formed, while stressing under acidic condition (1 N HCl, 80 °C, 36 h) resulted in Z3, Z7 and Z9. In basic stress condition (1 N NaOH, 80 °C, 48 h), the drug primarily degraded to Z2 and Z3. Exposure of the drug to UV and visible light resulted in seven products, viz., Z1–Z6 and Z8. The drug proved to be stable upon oxidative stress.
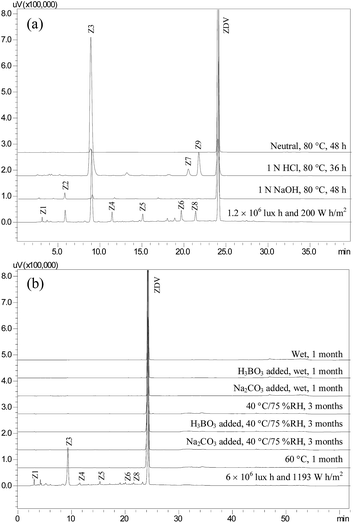 |
| Fig. 1 Chromatograms showing degradation products of zidovudine (ZDV) formed in solution (a) and solid state (b) stress conditions. | |
Solid state degradation behaviour of the drug
No degradation of the drug was observed in the first three sets of the four subjected to solid state stress studies. The drug showed instability in the fourth set, which was subjected to light, and resulted in Z3 as a major degradation product. Additionally, Z1, Z4–Z6, and Z8 were formed, but only in detectable amounts (Fig. 1b).
Mass spectrometric studies on the drug
HRMS line spectrum of ZDV (Fig. S1a†) showed accurate mass of m/z 268.1042, which had difference of 0.2 mmu with respect to the exact mass of 268.1040 Da (Table 1). As divulged by MSn studies, the drug molecular ion fragmented into product ion of m/z 225 on loss of HN3. The latter ion further dissociated into fragments of m/z 207 and 127 on loss of H2O and C5H6O2, respectively (Fig. 2).
Table 1 HRMS and MSn data of zidovudine (ZDV) and its degradation products Z1–Z9
|
HRMS data |
MSn data |
Accurate mass |
Exact mass |
Error (mmu) |
Accurate masses of fragments |
Precursor ion |
Product ion(s) |
Fragments were not captured for further MSn studies. |
[ZDV + H]+ |
268.1042 |
268.1040 |
0.2 |
225.0848, 207.0747, 127.0515 |
268 |
225, 127a |
268 → 225 |
207a, 127a |
[Z1 + H]+ |
240.0969 |
240.0979 |
−1.0 |
223.0712, 193.0623, 127.0635 |
240 |
223, 193a, 127a |
240 → 223 |
193a, 127a |
[Z2 + H]+ |
242.1134 |
242.1135 |
−0.1 |
225.0867, 207.0761, 127.0482 |
242 |
225, 127a |
242 → 225 |
207a, 127a |
[Z3 + H]+ |
127.0522 |
127.0502 |
2.0 |
— |
127a |
|
[Z4 + H]+ |
366.1360 |
366.1408 |
−4.8 |
251.0842, 242.1095, 240.0918, 214.0815, 196.0705, 184.0681, 142.0614 |
MSn data were similar to the fragment of m/z 366 of degradation product Z6 as shown below |
[Z5 + H]+ |
270.1071 |
270.1084 |
−1.3 |
144.0659, 127.0567 |
270 |
144a, 127a |
[Z6 + H]+ |
507.1968 |
507.1946 |
2.2 |
392.1345, 381.1502, 366.1406, 355.1364, 337.1254, 325.1256, 283.1130, 266.0879, 251.0764, 242.1122, 223.0657, 214.0944, 205.0687 |
507 |
392, 381, 366, 355, 283, 266, 251, 242, 240, 223, 205a |
507 → 392 |
251 |
507 → 381 |
283, 266, 240, 223, 205a, 142, 125a |
507 → 366 |
251, 242, 240, 214, 142, 125a, 116a |
507 → 355 |
337a, 325, 266, 223, 214, 196a, 184a, 125a |
507 → 325 |
184a |
507 → 283 |
266, 223, 142, 125a |
507 → 266 |
223, 125a |
507 → 251 |
127a |
507 → 242 |
116a |
507 → 240 |
142, 125a |
507 → 223 |
205a, 153a, 125a |
507 → 214 |
196a, 184a |
507 → 142 |
125a |
[Z7 + H]+ |
268.1017 |
268.1040 |
−2.3 |
225.0870, 127.0520 |
MSn data were similar to the drug (ZDV), as shown above |
[Z8 + H]+ |
272.0841 |
272.0877 |
−3.6 |
127.0482 |
272 |
212a, 127a |
[Z9 + H]+ |
268.1029 |
268.1040 |
−1.1 |
225.0848, 207.0744, 127.0515 |
MSn data were similar to the drug (ZDV), as shown above |
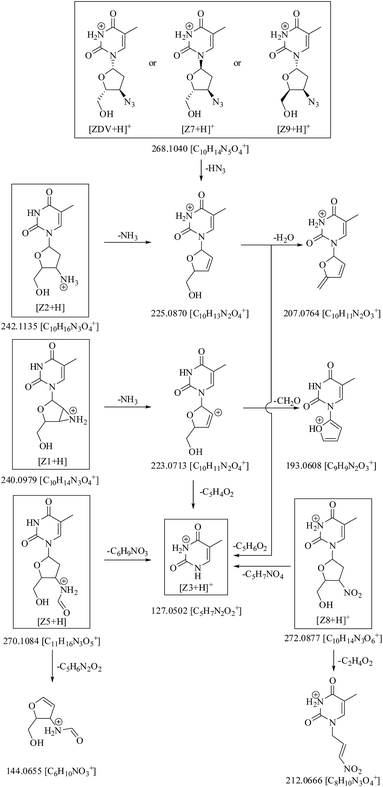 |
| Fig. 2 Mass fragmentation pathway of zidovudine (ZDV) and its degradation products Z1–Z3, Z5 and Z7–Z9 (solid boxes) under ESI +ve mode. | |
NMR spectroscopic studies on the drug
NMR spectroscopy studies on the drug were carried out in CD3OD. The resultant 1D and 2D NMR spectra are shown in Fig. S2,† while corresponding data are compiled in Table 2. 1H NMR spectrum showed a total of eleven non-labile protons, whereas the remaining two labile hydrogens were not observed due to H/D exchange. The following were the observed signals: (i) a singlet of methine proton of pyrimidine ring of position H-6 at δ 7.78 ppm; (ii) one singlet at δ 1.86 ppm corresponding to methyl protons of position H-7; (iii) a triplet at δ 6.15 ppm for methine proton of position H-8; (iv) multiplet at δ 2.34–2.42 ppm for methylene protons of position H-9; (v) two multiplets at δ 4.32–4.36 ppm and δ 3.88–3.90 ppm for methine proton of positions H-10 and H-11, respectively, and (vi) two pairs of multiplets at δ 3.71–3.74 ppm and δ 3.80–3.83 ppm for non-equivalent methylene protons of position H-12.
Table 2 1H, COSY, 13C, DEPT-135, HSQC and HMBC data of zidovudine (ZDV) in CD3ODa
Structure |
Position |
1H (δ ppm) |
COSY |
13C (δ ppm) |
DEPT-135 |
HSQC |
HMBC |
Signals were not observed; d, doublet; s, singlet, and m, multiplet. |
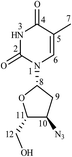 |
2 |
— |
— |
150.96 |
— |
— |
— |
4 |
— |
— |
165.06 |
— |
— |
— |
5 |
— |
— |
110.27 |
— |
— |
— |
6 |
7.78 (s) |
H-7 |
136.74 |
CH |
C-6 |
C-2, 4, 7, 8 |
7 |
1.86 (s) |
H-6 |
11.12 |
CH3 |
C-7 |
C-4, 5, 6 |
8 |
6.15 (t) |
H-9 |
84.70 |
CH |
C-8 |
C-2, 6 |
9 |
2.34–2.42 (m) |
H-8, 10 |
36.93 |
CH2 |
C-9 |
C-8, 10, 11 |
10 |
4.32–4.36 (m) |
H-9, 11 |
60.32 |
CH |
C-10 |
C-8, 11 |
11 |
3.88–3.90 (m) |
H-10, 12a, b |
84.74 |
CH |
C-11 |
* |
12a, b |
3.71–3.74 (m), 3.80–3.83 (m) |
H-11 |
61.07 |
CH2 |
C-12 |
C-10 |
13C NMR spectrum showed a total of ten carbons in the structure. The positions of methylene, methyl and methine carbons were differentiated using DEPT-135. Two signals arose at δ 150.96 ppm and δ 165.06 ppm for carbonyl carbons of positions C-2 and C-4, respectively. A single signal was observed at δ 110.27 ppm for quaternary carbon C-5. One methyl carbon corresponding to position C-7 was observed at δ 11.12 ppm. The signals for tertiary carbons, viz., C-6, C-8, C10 and C-11 arose at δ 136.74 ppm, δ 84.70 ppm, δ 60.32 ppm and δ 84.74 ppm, respectively. Another set of signals for two methylene carbons were observed at δ 36.93 ppm (C-9) and δ 61.07 ppm (C-12). While 2D (COSY, HSQC and HMBC) data helped to assign the connectivities between the atoms, stereochemistry of positions 8, 10 and 11 was confirmed by NOESY spectrum (Fig. 3). H-8 showed NOE correlations with H-11, which confirmed that both the protons were in the same plane, while absence of correlation between H-8 and H-10 indicated their presence in an opposite plane.
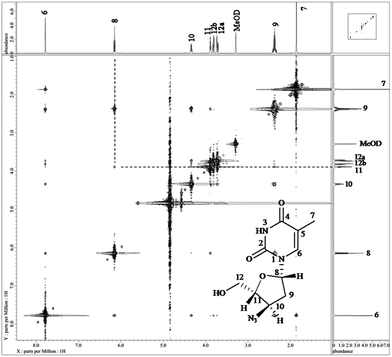 |
| Fig. 3 NOESY spectrum of zidovudine (ZDV) showing correlation between protons H-8 and H-11. | |
IR studies on the drug
IR spectrum of the drug (Fig. S3a†) showed bands for N–H stretching at 3463 cm−1, C–H stretching at 2932 cm−1, azide stretching at 2083 cm−1 (characteristic), two C
O stretching bands near 1687 cm−1, while scissoring, twisting, wagging and rocking vibrations were observed below 1500 cm−1.
Characterization of the degradation products Z1–Z9
The degradation products Z1–Z9 were characterized based on HRMS, MSn, NMR and IR results. HRMS line spectra of all the degradation products are shown in Fig. S1b–j,† while their accurate and exact masses and errors (mmu) along with MSn data are included in Table 1. NMR data were obtained for the degradation products Z5–Z9 (Table 3), while IR spectra were recorded for Z5, Z7 and Z9 (Fig. S3b–d,† respectively). The characterization of the degradation products is discussed below individually or in groups, categorized on the basis of similarities in their structures.
Table 3 1H, COSY, 13C, DEPT-135, HSQC and HMBC data of ZDV degradation products Z5–Z9 in CD3ODa
Structure |
Position |
1H (δ ppm) |
COSY |
13C (δ ppm) |
DEPT-135 |
HSQC |
HMBC |
Signals were not observed. Key: d, doublet; m, multiplet; s, singlet, and q, quartet. |
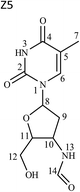 |
2 |
— |
— |
151.00 |
— |
— |
— |
4 |
— |
— |
165.11 |
— |
— |
— |
5 |
— |
— |
110.23 |
— |
— |
— |
6 |
7.86 (s) |
H-7 |
136.77 |
CH |
C-6 |
C-2, 4, 7, 8 |
7 |
1.87 (s) |
H-6 |
11.11 |
CH3 |
C-7 |
C-4, 5, 6 |
8 |
6.22 (t) |
H-9 |
84.55 |
CH |
C-8 |
C-2, 6 |
9 |
2.27–2.39 (m) |
H-8, 10 |
37.43 |
CH2 |
C-9 |
C-8, 10, 11 |
10 |
4.55–4.56 (m) |
H-9, 11 |
47.67 |
CH |
C-10 |
C-12 |
11 |
3.85–3.87 (m) |
H-10, 12a, b |
85.14 |
CH |
C-11 |
C-10 |
12a, b |
3.71–3.74 (m), 3.82–3.85 (m) |
H-11 |
60.96 |
CH2 |
C-12 |
C-10 |
14 |
8.06 (s) |
|
162.42 |
CH |
C-14 |
C-10 |
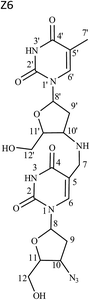 |
2 |
— |
— |
150.42 |
— |
— |
|
2′ |
— |
— |
150.95 |
— |
— |
|
4 |
— |
— |
163.74 |
— |
— |
|
4′ |
— |
— |
165.01 |
— |
— |
|
5 |
— |
— |
104.43 |
— |
— |
|
5′ |
— |
— |
110.53 |
— |
— |
|
6 |
8.31 (s) |
— |
142.93 |
CH |
C-6 |
C-2, 4, 7, 8 |
6′ |
7.75 (s) |
H-7′ |
136.83 |
CH |
C-6′ |
C-2′, 4′, 7′, 8′ |
7 |
3.97 (s) |
— |
42.84 |
CH2 |
C-7 |
C-4, 5, 6, 10′ |
7′ |
1.87 (s) |
H-6′ |
11.11 |
CH3 |
C-7′ |
C-4′, 5′, 6′ |
8 |
6.14 (t) |
H-9 |
85.45 |
CH |
C-8 |
C-6 |
8′ |
6.30 (t) |
H-9′a, b |
85.02 |
CH |
C-8′ |
C-2′, 6′ |
9 |
2.43–2.49 (m) |
H-8, 10 |
37.35 |
CH2 |
C-9 |
C-10 |
9′a, b |
2.54–2.60 (m), 2.69–2.73 (m) |
H-8′, 10′ |
34.58 |
CH2 |
C-9′ |
C-8′, 11′ |
10 |
4.37 (q) |
H-9, 11 |
59.94 |
CH |
C-10 |
C-8, 11 |
10′ |
4.00–4.04 (m) |
H-9′, 11′ |
57.37 |
CH |
C-10′ |
* |
11 |
3.92–3.93 (m) |
H-10, 12 |
85.16 |
CH |
C-11 |
* |
11′ |
4.25–4.29 (m) |
H-10′, 12a′, b′ |
82.15 |
CH |
C-11′ |
* |
12 |
3.85–3.87 (m) |
H-11 |
60.84 |
CH2 |
C-12 |
* |
12a′, b′ |
3.74–3.77 (m), 3.88–3.90 (m) |
H-11′ |
61.28 |
CH2 |
C-12′ |
C-11′ |
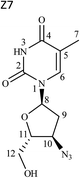 |
2 |
— |
— |
150.60 |
— |
— |
— |
4 |
— |
— |
164.91 |
— |
— |
— |
5 |
— |
— |
110.61 |
— |
— |
— |
6 |
7.69 (s) |
H-7 |
136.55 |
CH |
C-6 |
C-2, 4, 7, 8 |
7 |
1.88 (s) |
H-6 |
11.02 |
CH3 |
C-7 |
C-4, 5, 6 |
8 |
5.66–5.69 (m) |
H-9a, b |
79.99 |
CH |
C-8 |
C-2, 6 |
9a, b |
1.94–1.97 (m), 2.11–2.18 (m) |
H-8, 10 |
29.25 |
CH2 |
C-9 |
C-8, 10, 11 |
10 |
3.61–3.65 (m) |
H-9a, b, 11 |
58.29 |
CH |
C-10 |
* |
11 |
3.86 (s) |
H-10, 12a, b |
65.69 |
CH |
C-11 |
C-9, 10 |
12a, b |
3.72–3.75 (m), 4.01–4.04 (m) |
H-11 |
70.34 |
CH2 |
C-12 |
C-8, 10, 11 |
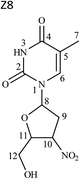 |
2 |
— |
— |
150.95 |
— |
— |
— |
4 |
— |
— |
164.97 |
— |
— |
— |
5 |
— |
— |
110.65 |
— |
— |
— |
6 |
7.78 (s) |
H-7 |
136.57 |
CH |
C-6 |
C-2, 4, 7, 8 |
7 |
1.87 (s) |
H-6 |
11.10 |
CH3 |
C-7 |
C-4, 5, 6 |
8 |
6.37 (t) |
H-9a, b |
85.25 |
CH |
C-8 |
C-2, 6 |
9a, b |
2.48–2.54 (m), 2.95–3.00 (m) |
H-8, 10 |
35.74 |
CH2 |
C-9 |
C-8, 10 |
10 |
5.30–5.33 (m) |
H-9a, b, 11 |
83.83 |
CH |
C-10 |
C-8 |
11 |
4.48–4.49 (m) |
H-10, 12 |
85.39 |
CH |
C-11 |
* |
12 |
3.81–3.89 (m) |
H-11 |
61.87 |
CH2 |
C-12 |
C-10 |
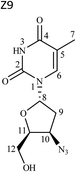 |
2 |
— |
— |
150.70 |
— |
— |
— |
4 |
— |
— |
164.88 |
— |
— |
— |
5 |
— |
— |
110.55 |
— |
— |
— |
6 |
7.48 (s) |
H-7 |
136.38 |
CH |
C-6 |
C-2, 4, 7, 8 |
7 |
1.85 (s) |
H-6 |
11.00 |
CH3 |
C-7 |
C-4, 5, 6 |
8 |
5.76–5.79 (m) |
H-9a, b |
77.39 |
CH |
C-8 |
C-2, 6 |
9a, b |
1.93–1.97 (m), 2.07–2.12 (m) |
H-8, 10 |
33.34 |
CH2 |
C-9 |
C-8, 10, 11 |
10 |
4.14–4.15 (m) |
H-9, 11 |
60.91 |
CH |
C-10 |
C-8, 11 |
11 |
3.97–4.01 (m) |
H-10, 12 |
66.71 |
CH |
C-11 |
C-10, 12 |
12a, b |
3.72–3.77 (m), 3.81–3.84 (m) |
H-11 |
66.41 |
CH2 |
C-12 |
C-8, 10, 11 |
Z1. The mass to charge ratio of Z1 was observed to be 240.0969 Da. Its molecular formula worked out as C10H14N3O4+, with exact mass of 240.0979 Da (error −1.0 mmu). The proposed structure and fragmentation pattern are shown in Fig. 2. The molecular ion fragmented in MS2 step into product ion of m/z 223 on loss of NH3, which on MS3 run reduced into fragments of m/z 193 and 127 on loss of CH2O and C5H4O2, respectively. The product was characterized as 1-(4-(hydroxymethyl)-3-oxa-6-azabicyclo[3.1.0]hexan-2-yl)-5-methylpyrimidine-2,4(1H,3H)-dione. It was previously proposed by us as an intermediate in the process of degradation of ZDV into thymine,7 but was not characterized experimentally at that time. Thus our previous hypothesis stands confirmed through characterization of the same intermediate in this study.
Z2. It was formed on base hydrolysis as well as on exposure of the drug solution to ICH recommended dose of light in a photostability chamber. Its accurate and exact masses were 242.1134 and 242.1135 Da, respectively (error = −0.1 mmu). The molecular formula for the product was determined to be C10H16N3O4+. It was characterized as 3′-amino-3′-deoxythymidine (AMT) (Fig. 2). This product is previously reported as a metabolite of the drug.23,24 The parent of m/z 242 fragmented into product ion of m/z 225 on loss of NH3, which further showed losses of H2O and C5H6O2 to result into fragment species of m/z 207 and 127, respectively.
Z3. This was formed as a major degradation product under almost all the stress conditions. Its accurate mass was determined to be 127.0522 Da, whereas exact mass calculated out to be 127.0502 Da (error = 2 mmu). The molecular formula was found to be C5H7N2O2+. These data helped in characterization of the product as 5-methyluracil (thymine, Fig. 2). The same is mentioned as an impurity in multiple compendial monographs.25–27 Even we detected Z3 as the major degradation product of the drug in our earlier studies.7,8
Z4 and Z6. [Z4 + H]+ and [Z6 + H]+ had accurate masses of 366.1360 and 507.1968 Da; exact masses of 366.1408 (error = −4.8 mmu) and 507.1946 Da (error = 2.2 mmu); and molecular formulae C15H20N5O6+ and C20H27N8O8+, respectively. The characterized structures of both the products, along with their fragmentation behaviour, are shown in Fig. 4. The product Z6 was postulated as 1-(4-azido-5-(hydroxymethyl)tetrahydrofuran-2-yl)-5-((2-(hydroxymethyl)-5-(5-methyl-2,4-dioxo-3,4-dihydropyrimidin-1(2H)-yl)tetrahydrofuran-3-ylamino)methyl)pyrimidine-2,4(1H,3H)-dione. Its generation involved interaction of the methyl group of one drug molecule with the azide moiety of another, releasing N2 in the process.
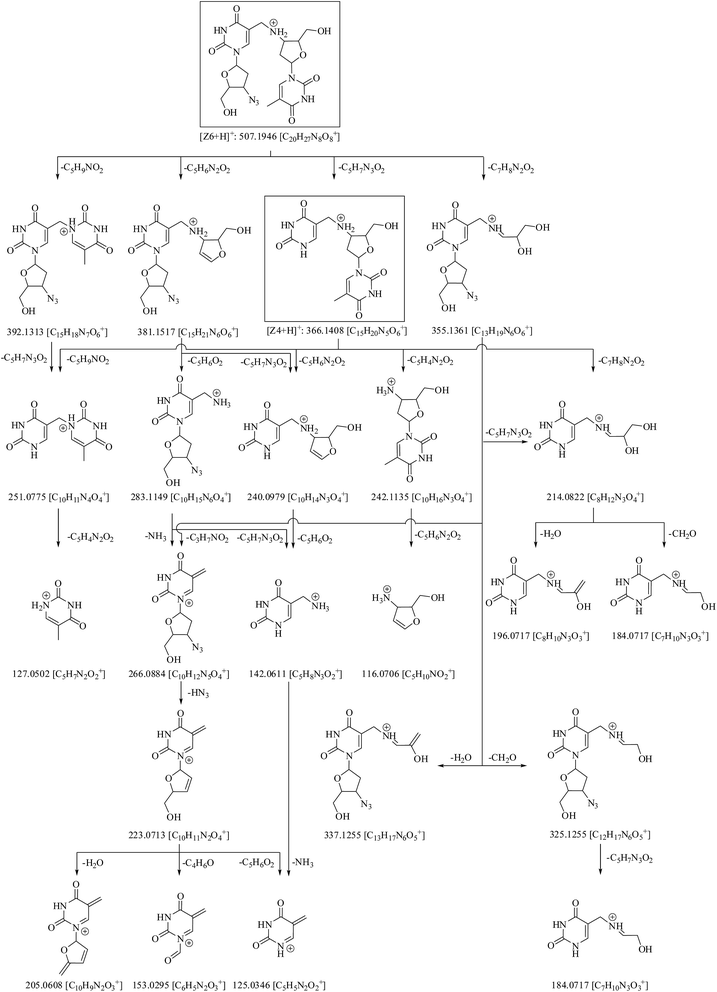 |
| Fig. 4 Structures and fragmentation behaviour of degradation products Z4 and Z6 (solid boxes). Fragments of m/z 153, 127, 125 and 116 were observed only during MSn study. | |
Fig. 4 shows that molecular ion of Z6 fragmented into product ions of m/z 392, 381, 366 and 355 on the loss of C5H9NO2, C5H6N2O2, C5H7N3O2 and C7H8N2O2, respectively. Of these, the species of m/z 366 had the same mass as molecular ion of Z4. The precursor ion of m/z 392 fragmented into product ion of m/z 251 on loss of C5H7N3O2. The latter product ion was also formed from fragment of m/z 366 on loss of C5H9NO2. The ion of m/z 381 reduced into species of m/z 283 and 240 on loss of C5H6O2 and C5H7N3O2, respectively. The precursor of m/z 283 further degraded via routes: (i) m/z 283 → 266 (loss of NH3) → 223 (loss of HN3) → 205, 153 and 125 (on loss of H2O, C4H6O and C5H6O2, respectively) and (ii) m/z 283 → 142 (loss of C5H7N3O2) → 125 (loss of NH3). The fragment of m/z 366 also dissociated into product ions of m/z 251 and 240 on loss of C5H9NO2 and C5H6N2O2, respectively, along with ions of m/z 242 and 214 on loss of C5H4N2O2 and C7H8N2O2, respectively. The precursor of m/z 242 further reduced into fragment of m/z 116 on loss of C5H6N2O2, while the precursor of m/z 214 dissociated into ions of m/z 196 and 184 on loss of H2O and CH2O, respectively. Another MS2 product ion of m/z 355 fragmented into species of m/z 337, 325 and 266 on loss of H2O, CH2O and C3H7NO2, respectively. The fragment of m/z 325 reduced into ion of m/z 184 on loss of C5H7N3O2.
The mass fragmentation spectra of Z6 and Z4 were overlapping beyond m/z 366 (Table 1, Fig. S1†). Therefore, Z4 was indicated to be breakdown product of Z6 arising on loss of 4-azido-5-(hydroxymethyl)tetrahydrofuran-2-yl moiety. Its structure was proposed as 1-(4-((2,4-dioxo-1,2,3,4-tetrahydro pyrimidin-5-yl)methylamino)-5-(hydroxymethyl)tetrahydro furan-2-yl)-5-methylpyrimidine-2,4(1H,3H)-dione.
Due to unusual structure of Z6, and it being precursor to generation of Z4, therefore, it was considered prudent to confirm its structure by 1H, COSY, 13C, DEPT-135, HSQC and HMBC NMR studies. The spectra are shown in Fig. S4† and corresponding data are compiled in Table 3. The following were the key observations: (i) twenty one protons appeared in 1H NMR spectrum of Z6 (Fig. S4a†) against eleven in the drug (Fig. S2a†), highlighting that Z6 was truly formed via interaction of two ZDV molecules; (ii) two signals were observed corresponding to individual signal of proton(s) in the drug molecule, except methyl protons, where only one signal was observed (Fig. S4a versus S2a†); (iii) DEPT-135 experiment substantiated that specific signal at δ 3.97 ppm appeared because of methylene protons in Z6 (Fig. S4a and c†), and (iv) HMBC data showed connectivity of H-7 to C-10′, thus supporting coupling of two molecules via methylene and amino groups.
Z5. The molecular ion of Z5 had accurate and exact masses as 270.1071 and 270.1084 Da, respectively (error −1.3 mmu). The molecular formula worked out to be C11H16N3O5+. On collision induced dissociation, it formed product ions of m/z 144 and 127 on loss of C5H6N2O2 and C6H9NO3, respectively. The structure was assigned as N-(2-(hydroxymethyl)-5-(5-methyl-2,4-dioxo-3,4-dihydropyrimidin-1(2H)-yl)tetrahydrofuran-3-yl)formamide (Fig. 2). The same was confirmed through 1D and 2D NMR results (Fig. S5†, Table 3). Twelve protons appeared in the 1H NMR spectrum (Fig. S5a†) in comparison to eleven in case of the drug (Fig. S2a†). The change in the azide group was highlighted through chemical shift values of 1H (δ 4.55–4.56 ppm) and 13C (δ 47.67 ppm) for position 10 as compared to the drug (δ 4.32–4.36 ppm and δ 60.32 ppm, respectively) (Table 3). The addition of CHO and removal of N2, which was highlighted by HRMS results, was confirmed by one additional signal for 1H and 13C at δ 8.06 and 162.42 ppm, respectively. The same was substantiated by HMBC data where H-14 showed connectivity with C-10 (Fig. S5e†). The structure was further supported by IR spectrum (Fig. S3b†), in which absorption band of azide (∼2083 cm−1) was absent as compared to the drug spectrum (Fig. S3a†).
Z7 and Z9. Accurate masses of [Z7 + H]+ and [Z9 + H]+ were 268.1017 and 268.1029 Da, respectively (Table 1). The two had same exact mass and molecular formula as the drug, viz., m/z 268.1040 and C10H14N5O4+, respectively. Both also showed similar fragmentation behaviour to the drug, following the route: m/z 268 → 225 (loss of HN3) → 207, 127 (loss of H2O, C5H6O2, respectively) (Fig. 2). Accordingly, the two were considered to be isomeric in nature, not only among themselves, but also with respect to the drug. Characterization of their structures was attempted through 1H, 13C, COSY, HSQC, HMBC and NOESY NMR spectra (Table 3, and Fig. S6, S7† and 5) and also IR studies (Fig. S3c and d†).
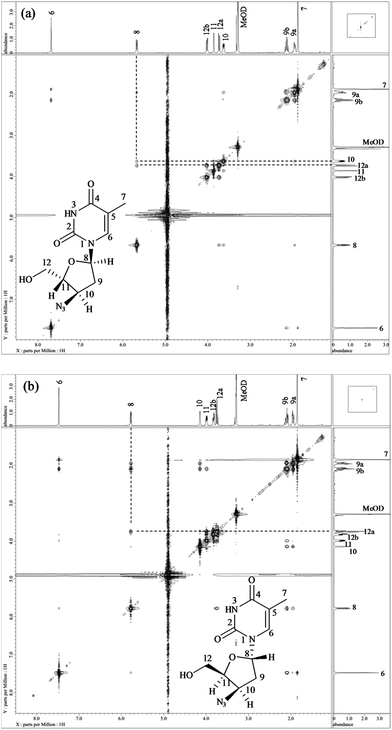 |
| Fig. 5 NOESY spectra of degradation products Z7 (a) and Z9 (b) showing correlation of proton H-8 with H-10, 12a in Z7 and H-8 with 12a in Z9. | |
1H and 13C data of both Z7 and Z9 were almost similar to the drug, except minor changes in chemical shifts for protons at positions H-8 to H-11. Even COSY, HSQC and HMBC correlations were similar. However, in case of Z7, NOE correlations of H-8 existed with positions H-10 and H-12a (Fig. 5a), which were absent in case of the drug (Fig. 3). Oppositely, similar correlation was absent between positions H-8 and H-11 that otherwise was present in the drug. This indicated that proton of H-8 was positioned in opposite plane to the drug. Hence the structure of Z7 was characterized as 1-((2S,4R,5R)-4-azido-5-(hydroxymethyl)tetrahydrofuran-2-yl)-5-methyl pyrimidine-2,4(1H,3H)-dione. Against it, NOESY spectrum of Z9 showed correlation of H-8 with H-12 (Fig. 5b), which was absent in the drug (Fig. 3). In this case, correlation of H-8 and H-11 was oppositely absent, which existed in the drug. This indicated that H-11 proton changed its plane as compared to the drug. Hence the structure of Z9 was characterized as 1-((2S,4R,5S)-4-azido-5-(hydroxymethyl)tetrahydrofuran-2-yl)-5-methylpyrimidine-2,4(1H,3H)-dione. As expected, the two products had almost identical IR spectra among themselves and also to the drug (Fig. S3a versus c and d†).
Z8. This degradation product had accurate and exact masses of 272.0841 and 272.0877 Da (error −3.6 mmu), respectively. For the same, the molecular formula was determined as C10H14N3O6+. The molecular ion in this case fragmented into product ions of m/z 212 and 127 on loss of C2H4O2 and C5H7NO4, respectively (Fig. 2).1D and 2D NMR spectra of this product are shown in Fig. S8† and the data are included in Table 3. 1H NMR spectrum showed signals for eleven protons, the same number as the drug. While proton of position H-10 was deshielded to δ 5.30–5.33 ppm in comparison to the drug (δ 4.32–4.36 ppm), C-10 was also shifted to higher value of δ 83.83 ppm due to the presence of nitro group at position 10, instead of the azide moiety in the drug. However, there was no difference in 2D correlations. The structure was characterized as 3′-deoxy-3′-nitrothymidine (Fig. 2). The compound is known in the literature, but not as a degradation product of ZDV.
Degradation pathway
The mechanism of formation of the major degradation product Z3 (thymine) under hydrolytic (acid, neutral and alkaline) and photolytic conditions was proposed by us earlier.7 Fig. 6 outlines the complete pathway of degradation of ZDV to products Z1–Z9. Formation of thymine from ZDV under acidic conditions is postulated to involve release of (2R,3R)-3-azido-2-(hydroxymethyl)-3,4-dihydro-2H-furanium cation. The attack of thymine back on this cation, but from α face, is the most likely explanation to the formation of Z7. The same cation on rearrangement to (2S,3R)-3-azido-2-(hydroxymethyl)-3,4-dihydro-2H-furanium cation, and again attack of thymine from the α face, explains the formation of Z9. The drug possesses an azide moiety and the same is well known to be responsible for the lability of the drug under photochemical conditions.7,28,29 It is also established that azide groups undergo elimination of molecular nitrogen to generate nitrene as a reactive intermediate, which can undergo intramolecular C–H insertion reaction and photo-oxidation, leading to formation of aziridine and nitro products.30–32 This chemistry explains formation of degradation products Z1 and Z8, respectively. The conversion of nitrene intermediate (generated in the presence of light) to amine in the presence of water33 can be considered responsible for the formation of Z2 under photolytic conditions. Also, it is conceived that methyl group of the drug undergoes hydrogen abstraction and forms methylene radical, which interacts with azide group of another drug molecule, leading to Z6 on the removal of N2. Z4 is postulated to be formed further from Z6 on photolytic cleavage of 3-azido-2-(hydroxymethyl)-3,4-dihydro-2H-furyl moiety. Finally, Z5 is probably generated upon hydrogen abstraction from methylene group of Z6, and photooxidation of the resultant methine radical.
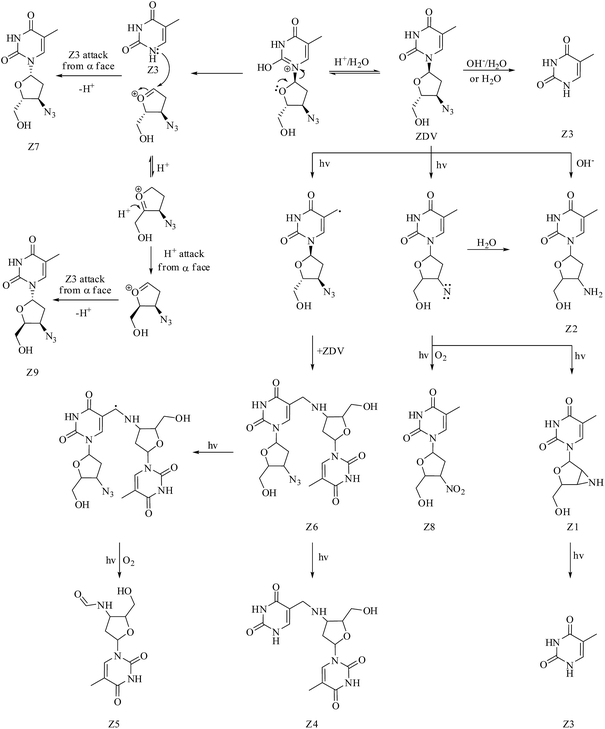 |
| Fig. 6 Postulated degradation pathway of zidovudine (ZDV) into degradation products Z1–Z9. | |
In silico toxicity of degradation products as compared to drug
Table 4 lists in silico toxicity data for ZDV and its degradation products Z1–Z9, which were predicted using extended TOPKAT model. The results of Z7 and Z9 were not distinguished from the drug due to stereo-isomeric nature of these compounds. The analysis of the probability values for remaining degradation products concluded that toxicity profiles for many parameters were mostly similar to the drug. For example, mouse female NTP model highlighted carcinogenic potential of all the products. Probability values for all the degradation products for skin (none versus irritant) and ocular (none versus irritant and mild versus moderate) irritancy were also in the toxic zone, similar to the drug. Of course, there were exceptions, like mouse female FDA (none versus carcinogen) model, which showed high risk in the case of degradation products Z5–Z9. The value of rat maximum tolerated dose gavage (g kg−1) was 42.3725 for the drug, 15.8685 for Z6, and for all other products. This indicated potential towards toxicity of the latter.
Table 4 Data of different toxicity models of zidovudine (ZDV) and its degradation products by TOPKAT analyses
Modela |
ZDV/Z7/Z9 |
Z1 |
Z2 |
Z3 |
Z4 |
Z5 |
Z6 |
Z8 |
Values in bold numbers highlight significant response and —, not predicted. |
NTP carcinogenicity call (male rat) (v3.2) |
0.601205 |
0.635117 |
0.621936 |
0.6338 |
0.5827 |
0.614807 |
0.569039 |
0.651193 |
NTP carcinogenicity call (female rat) (v3.2) |
0.389191 |
0.451652 |
0.425216 |
0.471237 |
0.353482 |
0.398239 |
0.380975 |
0.420601 |
NTP carcinogenicity call (male mouse) (v3.2) |
0.956751 |
0.6046 |
0.609786 |
0.539936 |
0.603602 |
0.605863 |
0.900116 |
0.689328 |
NTP carcinogenicity call (female mouse) (v3.2) |
0.98775 |
0.832232 |
0.896836 |
0.595784 |
0.862657 |
0.895145 |
0.974164 |
0.872737 |
FDA carcinogenicity male rat none versus carcinogen (v3.1) |
0.223355 |
0.229735 |
0.227367 |
0.262078 |
0.226143 |
0.230836 |
0.207828 |
0.228379 |
FDA carcinogenicity female rat none versus carcinogen (v3.1) |
0.565062 |
0.379458 |
0.436632 |
0.294931 |
0.406877 |
0.416319 |
0.434721 |
0.429074 |
FDA carcinogenicity female rat single versus multiple (v3.1) |
0.365768 |
0.369808 |
0.319705 |
0.469442 |
0.320719 |
0.415096 |
0.266249 |
0.441739 |
FDA carcinogenicity male mouse none versus carcinogen (v3.1) |
0.270298 |
0.340455 |
0.325645 |
0.401572 |
0.278675 |
0.357296 |
0.197168 |
0.348016 |
FDA carcinogenicity male mouse single versus multiple (v3.1) |
0.178152 |
0.214562 |
0.211127 |
0.351254 |
0.232026 |
0.177657 |
— |
0.185946 |
FDA carcinogenicity female mouse none versus carcinogen (v3.1) |
0.880708 |
0.512474 |
0.657823 |
0.332135 |
0.611698 |
0.701862 |
0.716486 |
0.707546 |
FDA carcinogenicity female mouse single versus multiple (v3.1) |
0.212682 |
0.255724 |
0.245871 |
0.363404 |
0.247947 |
0.227505 |
0.218432 |
0.252767 |
Weight of evidence carcinogenicity call (v5.1) |
0.343849 |
0.455159 |
0.417765 |
0.551344 |
0.426989 |
0.446128 |
0.296666 |
0.444503 |
Ames mutagenicity (v3.1) |
0.770937 |
0.51182 |
0.488866 |
0.663337 |
0.402557 |
0.44671 |
0.68296 |
0.534997 |
Developmental toxicity potential (v3.1) |
0.532854 |
0.581491 |
0.575856 |
0.538579 |
0.609412 |
0.551531 |
0.591402 |
0.524646 |
Rat oral LD50 (v3.1) (g kg−1) |
1.45463 |
1.75821 |
6.51255 |
1.79644 |
8.05157 |
7.03513 |
1.65473 |
5.69872 |
Rat maximum tolerated dose-feed/water (v6.1) (g kg−1) |
0.084385 |
0.069803 |
0.198136 |
0.050081 |
0.150368 |
0.138981 |
0.06585 |
0.161187 |
Rat maximum tolerated dose-gavage (v6.1) (g kg−1) |
42.3725 |
0.791777 |
0.054089 |
0.76531 |
0.216738 |
0.226869 |
15.8685 |
0.0965 |
Rat inhalational LC50 (v6.1) (mg m−3 H) |
10.8997 |
6.47236 |
2.37268 |
4.6922 |
1.95652 |
4.233 |
0.976777 |
3.5073 |
Chronic LOAEL (v3.1) (g kg−1) |
0.025337 |
0.014277 |
0.0171 |
0.0464 |
0.01105 |
0.009848 |
0.013132 |
0.029086 |
Skin irritancy none versus irritant (v6.1) |
0.972763 |
0.973142 |
0.973568 |
0.972897 |
0.976674 |
0.973586 |
0.976146 |
0.975179 |
Skin irritancy mild versus moderate severe (v6.1) |
— |
— |
— |
— |
0.183925 |
— |
0.115629 |
0.076978 |
Skin sensitization none versus sensitizer (v6.1) |
0.687369 |
0.660831 |
0.663507 |
0.69953 |
0.58999 |
0.633592 |
0.611812 |
0.655309 |
Ocular irritancy none versus irritant (v5.1) |
0.999058 |
0.97282 |
0.999136 |
0.998792 |
0.999228 |
0.999031 |
0.99891 |
0.999092 |
Ocular irritancy mild versus moderate severe (v5.1) |
0.854634 |
0.853354 |
0.851304 |
0.826827 |
0.829871 |
0.853052 |
0.864532 |
0.848511 |
Ocular irritancy moderate versus severe (v5.1) |
0.621798 |
0.686695 |
0.676626 |
— |
— |
0.549875 |
0.688957 |
0.621227 |
Aerobic biodegradability (v6.1) |
0.614863 |
0.63815 |
0.663655 |
0.594296 |
0.668784 |
0.675824 |
0.58103 |
0.525429 |
Fathead minnow LC50 (v3.2) (g l−1) |
0.353352 |
2.09353 |
1.35531 |
2.30437 |
4.03792 |
1.26157 |
0.714022 |
0.444518 |
Daphnia EC50 (v3.1) (mg l−1) |
10.406 |
4.02122 |
17.7645 |
1.58969 |
9.17126 |
10.0216 |
3.95292 |
6.60556 |
Conclusion
The study explored forced degradation chemistry of ZDV in both solution and solid states. The degradation products were characterized using HRMS, MSn, NMR and IR data. Their toxicity potential was assessed using extended TOPKAT model. The drug was labile only under hydrolytic and photolytic stress conditions. A total of nine degradation products were formed. A noteworthy observation was the generation of 3′-amino-3′-deoxythymidine (AMT, Z2) upon base hydrolysis and photolysis of the drug. This product is a known toxic catabolite of ZDV. In silico prediction also revealed toxicity potential of other degradation products. Since hydrolysis and photolysis are the two key mechanisms responsible for degradation of the drugs in the environmental matrices, the formation of more toxic degradation products Z2 and Z5–Z9 of ZDV under the same conditions may be a valid case to suggest their monitoring in the environment.
References
- M. Kurmi, V. M. Golla, S. Kumar, A. Sahu and S. Singh, RSC Adv., 2015, 5, 96117–96129 RSC.
- M. Kurmi, B. S. Kushwah, A. Sahu, M. Narayanam and S. Singh, J. Pharm. Biomed. Anal., 2016, 125, 245–259 CrossRef CAS PubMed.
- M. Kurmi, D. K. Singh, S. Tiwari, P. Sharma and S. Singh, J. Pharm. Biomed. Anal., 2016, 128, 438–446 CrossRef CAS PubMed.
- V. M. Golla, M. Kurmi, K. Shaik and S. Singh, J. Pharm. Biomed. Anal., 2016, 131, 146–155 CrossRef CAS PubMed.
- M. Kurmi, A. Sahu and S. Singh, J. Pharm. Biomed. Anal., 2017, 134, 372–384 CrossRef CAS PubMed.
- RETROVIR, http://www.accessdata.fda.gov/scripts/cder/drugsatfda/index.cfm?fuseaction=Search.DrugDetails, accessed Sep 2016.
- A. Dunge, A. K. Chakraborti and S. Singh, J. Pharm. Biomed. Anal., 2004, 35, 965–970 CrossRef CAS PubMed.
- A. Dunge, N. Sharda, B. Singh and S. Singh, J. Pharm. Biomed. Anal., 2005, 37, 1109–1114 CrossRef CAS PubMed.
- P. S. Devrukhakar, M. S. Shankar, G. Shankar and R. Srinivas, J. Pharm. Anal., 2017 DOI:10.1016/j.jpha.2017.01.006.
- P. Aparna, S. V. Rao, K. M. Thomas, K. Mukkanti, P. B. Gupta, K. Rangarao, G. K. Narayan, T. Sandip and K. Upendra, Pharmazie, 2010, 65, 331–335 CAS.
- J. V. dos Santos, L. A. E. B. de Carvalho and M. E. Pina, Anal. Sci., 2011, 27, 283–289 CrossRef CAS PubMed.
- D. Mandloi, P. Tripathi, P. Mohanraj, N. S. Chauhan and J. R. Patel, J. Liq. Chromatogr. Relat. Technol., 2011, 34, 601–612 CrossRef CAS.
- R. M. Kulkarni, V. S. Bhamare and B. Santhakumari, Desalin. Water Treat., 2016, 57, 24999–25010 CrossRef CAS.
- E. M. Cretton, M. Y. Xie, R. J. Bevan, N. M. Goudgaon, R. F. Schinazi and J.-P. Sommadossi, Mol. Pharmacol., 1991, 39, 258–266 CAS.
- M. P. Stagg, E. M. Cretton, L. Kidd, R. B. Diasio and J.-P. Sommadossi, Clin. Pharmacol. Ther., 1992, 51, 668–676 CrossRef CAS PubMed.
- L. Placidi, E. M. Cretton, M. Placidi and J.-P. Sommadossi, Clin. Pharmacol. Ther., 1993, 54, 168–176 CrossRef CAS PubMed.
- AIDS by the numbers, UNAIDS 2016, http://www.unaids.org/sites/default/files/media_asset/AIDS-by-the-numbers-2016_en.pdf, accessed Sep. 2016.
- C. Zhou, J. Chen, Q. Xie, X. Wei, Y. Zhang and Z. Fu, Chemosphere, 2015, 138, 792–797 CrossRef CAS PubMed.
- S. Singh and M. Bakshi, Pharm. Technol., 2000, 4, 1–14 Search PubMed.
- S. Singh, M. Junwal, G. Modhe, H. Tiwari, M. Kurmi, N. Parashar and P. Sidduri, Trends Anal. Chem., 2013, 49, 71–88 CrossRef CAS.
- J. C. Dearden, J. Comput.-Aided Mol. Des., 2003, 17, 119–127 CrossRef CAS PubMed.
- K. Enslein, V. K. Gombar and B. W. Blake, Mutat. Res., 1994, 305, 47–61 CrossRef CAS PubMed.
- E. P. Acosta, L. M. Page and C. V. Fletcher, Clin. Pharmacokinet., 1996, 30, 251–262 CrossRef CAS PubMed.
- G. J. Veal and D. J. Back, Gen. Pharmacol., 1995, 26, 1469–1475 CrossRef CAS PubMed.
- The International Pharmacopoeia, http://apps.who.int/phint/pdf/b/Jb.6.1.435.pdf, accessed Sep. 2016 Search PubMed.
- Zidovudine, United States Pharmacopeia, USP30-NF25, 2007, p. 3489 Search PubMed.
- Zidovudine, Indian Pharmacopoeia, IP, 2010, pp. 2329–2330 Search PubMed.
- S. V. Zelentsov, N. V. Zelentsova, A. B. Zhezlov and A. V. Oleinik, High Energy Chem., 2000, 34, 164–171 CrossRef CAS.
- S. Brase, C. Gil, K. Knepper and V. Zimmermann, Angew. Chem., Int. Ed., 2005, 44, 5188–5240 CrossRef CAS PubMed.
- F. D. Lewis and W. H. Saunders Jr, in Nitrenes, Interscience, ed. W. Lwosski, New York, 1970, p. 47 Search PubMed.
- O. E. Edwards, in Nitrenes, Interscience, ed. W. Lwosski, New York, 1970, p. 225 Search PubMed.
- S. V. Zelentsov, N. V. Zelentsova and A. A. Shchepalov, High Energy Chem., 2002, 36, 326–332 CrossRef CAS.
- H. Peng, K. H. Dornevil, A. B. Draganov, W. Chen, C. Dai, W. H. Nelson, A. Liu and B. Wang, Tetrahedron, 2013, 69, 5079–5085 CrossRef CAS PubMed.
Footnote |
† Electronic supplementary information (ESI) available. See DOI: 10.1039/c7ra00678k |
|
This journal is © The Royal Society of Chemistry 2017 |