DOI:
10.1039/C7RA00485K
(Paper)
RSC Adv., 2017,
7, 11355-11361
Shell thickness-dependent antibacterial activity and biocompatibility of gold@silver core–shell nanoparticles†
Received
12th January 2017
, Accepted 6th February 2017
First published on 14th February 2017
Introduction
To cope with the overwhelming challenges of infectious diseases resulting from pathogenic bacteria, nano-sized materials, emerging as new types of safe and cost-effective bactericidal materials, have been widely investigated. Among those numerous nanomaterials, silver nanoparticles (Ag NPs) are the most promising antibacterial agents because of their inherent properties of high thermal stability, limited microbial resistance, and broad-spectrum antimicrobial activity against bacteria, viruses and other eukaryotic microorganisms.1–4 Sondi and co-workers5 demonstrated that Ag NPs can easily adsorb to the membrane surface of bacteria through electrostatic interaction, form permeable pits, and cause an osmotic collapse in the cells. The other possible mechanism is the release of silver ions (Ag+) from the oxidized Ag NPs.6–8 Ag NPs are easy to oxidize and produce a high concentration of Ag+, the released Ag+ then destroys the respiratory chain and leads to the formation of reactive oxygen species (ROS), such as hydroxyl radicals, H2O2 and hydroperoxyl radicals, which finally trigger cell damage of the cellular components and leads to the death of the bacteria.9–11 In spite of this, the easy oxidation of pure Ag NPs and the toxicity in mammalian cells remain challenges in Ag NP applications.12–14
To address this problem, Ag NPs have been decorated with other nanomaterials by the formation of nanostructures. Song et al.15 synthesized the silver/polyrhodanine composite-decorated silica nanoparticles, which exhibited excellent antimicrobial activity toward Escherichia coli (E. coli) and Staphylococcus aureus (S. aureus) because of the antibacterial effects of both the silver nanoparticles and the polyrhodanine. Tan et al.16 synthesized antibacterial Ag@dsDNA@GO, which can wrap around the Xanthomonas perforans cell and results in rapid cell apoptosis, showing the synergistic antibacterial effect. While these nanostructures can effectively enhance the antibacterial activity of Ag NPs, they often require complex and tedious preparation methods, and suffering from low yields, poor long-term antibacterial activity, and biocompatibility problems.17,18
Bimetallic core–shell nanoparticles are gradually attracting more and more attention in recent years, because of their unique electronic, optical, chemical, and catalytic properties.19–24 These properties are distinct and superior to their constituent monometallic elements due to the new synergistic effects. Furthermore, core–shell nanoparticles are highly functional materials with modified properties. By changing the constituting core or shell materials, the properties of the overall particle such as thermal stability, antibacterial activity, and cytotoxicity can be tailored.25–28 Au NPs have advantages of easy preparation, homogeneity and biocompatibility, but suffer from low antibacterial property. Madhuchanda Banerjee et al.29 fabricated bimetallic Au@Ag NPs with positive charges on the surface. This structure showed enhanced antibacterial activity against both Gram negative and Gram positive bacteria at low concentration of silver present in the shell. The MIC values in terms of effective silver concentration in core–shell NPs are much lower than that of Ag NPs, indicating that their bactericidal efficiency was much higher than that of similar sized Ag NPs. However, the influence of shell thickness of Au@Ag NPs on antibacterial activities and toxicity have not been studied, and the antibacterial mechanism is still not very clear. Because the size, surface charge and coating of NPs play a significant role in the antibacterial properties, it is necessary to control the thickness of the silver shell to obtain excellent antibacterial activity against bacteria and low toxicity to healthy human cells.30
Here, we synthesize high yields of Au@Ag NPs with a fixed diameter of gold core and a tunable shell thickness using poly(vinyl pyrrolidone) (PVP) as stabilizing agent. Gram-negative bacteria E. coli and Gram-positive bacteria S. aureus as model bacteria were used to investigate antibacterial activities of the Au@Ag NPs. We studied the influence of the thickness of silver shell and the dosages of Au@Ag NPs on their bioactivities. The minimum inhibitory concentration (MIC) of Au@Ag NPs was also evaluated to qualitatively estimate the antibacterial efficiency. In parallel, Au NPs, Ag NPs, and the simple mixture of Ag NPs and Au NPs at the same concentration were used as controls for comparison. The biocompatibility of Au@Ag NPs toward mammalian cells (SH-SY5Y) were evaluated using MTT assay and the antibacterial mechanism of Au@Ag NPs were measured using the live/dead assay and the SEM images.
Results and discussion
Characterization of gold@silver core–shell nanoparticles
In this study, the bimetallic Au@Ag NPs were synthesized by deposition of Ag+ on the surface of gold core nanoparticles (10 nm) and then reduced by sodium ascorbate to form Ag shell, in the meantime, PVP was used as stabilizing agent binding to the surface of Au@Ag NPs. Transmission electron microscopy (TEM) images revealed that the prepared Au@Ag NPs were monodispersed and exhibited distinct variation in contrast between the dark gold core and the lighter silver shell (Fig. 1A–D and S1, ESI†). Statistical analysis of as many as 200 images indicated that the yield for the prepared Au@Ag NPs was as high as 98%. Besides, for three types of Au@Ag NPs, with the increase in the concentration of Ag+ ions, the shell thickness was increased. Based on the results of inductively coupled plasma mass spectrometry (ICP-MS) (Table S1, ESI†), the content of silver in Au@Ag-1, Au@Ag-2, and Au@Ag-3 NPs was 2.6 µg mL−1, 4.0 µg mL−1, 15.5 µg mL−1, respectively, and the Au/Ag ratio was 3
:
2, 1
:
1, and 1
:
4, respectively.
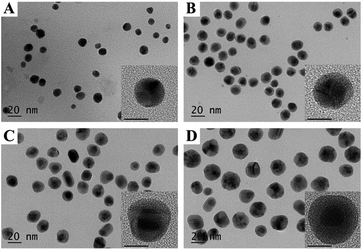 |
| Fig. 1 TEM and high-resolution TEM (inset) images of (A) Au NPs, (B) Au@Ag-1 NPs, (C) Au@Ag-2 NPs, (D) Au@Ag-3 NPs. The scale bars in high-resolution TEM images represent 10 nm. | |
Hydrodynamic diameters of Au@Ag NPs with different thickness was determined by dynamic light scattering (DLS) (Fig. S2A, ESI†). With the increase in the concentration of AgNO3, the diameter of Au@Ag NPs was increased from 13.5 to 28.2 nm, the average shell thickness of Au@Ag-1, Au@Ag-2, and Au@Ag-3 NPs were 1.5 nm, 5.0 nm, and 8.8 nm, respectively, (Fig. S2B, ESI†) based on DLS spectrum, which are in agreement with the statistical results of TEM images. Zeta potential of Au@Ag NPs was measured to be −5 mV using dynamic light scattering (results not shown). For the colloid solution, with the increased of the concentration of AgNO3, the color changed from original red (Au NPs) to yellow depending on the extent of coverage of Ag atoms on the surface of Au NPs (Fig. S2C, ESI†). The UV-Vis spectra of Au NPs and Ag NPs showed an absorbance peak at 520 nm and 400 nm, respectively, corresponding to a pure monometallic NPs. Au@Ag NPs showed double peaks at 400 nm and 500 nm, and the absorbance increased as the shell thickness increased, which consists with previous reports (Fig. S2D, ESI†).31,32
Shell thickness-dependent antibacterial activity of gold@silver core–shell nanoparticles
To investigate the influence of shell thickness of Au@Ag NPs on the antibacterial activity, we treated E. coli (Gram-negative bacteria) and S. aureus (Gram-positive bacteria) suspensions with three types of the synthetic Au@Ag NPs at different concentrations. The content of Au@Ag NPs was expressed by the total concentration of Ag and Au measured by ICP-MS. As shown in Fig. 2A and B, all NPs exhibited dose-dependent antibacterial activity against E. coli and S. aureus, the percent of dead bacteria was increased with the increase in the concentration of NPs. Furthermore, for three types of core–shell NPs, Au@Ag-2 NPs with the shell thickness of 5 nm and Au@Ag-3 NPs with the shell thickness of 8.8 nm showed the higher antibacterial activity compared with Au@Ag-1 NPs at the same concentration. Specifically, Au@Ag-2 NPs at the concentration of 5 µg mL−1 killed 99% E. coli and 97% S. aureus.
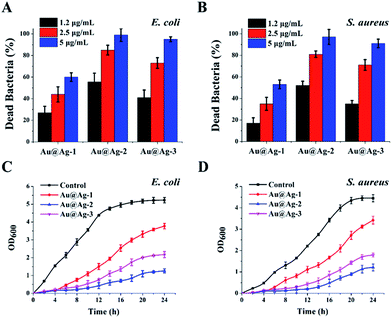 |
| Fig. 2 Percentage of dead bacteria of (A) E. coli and (B) S. aureus after treatment with three types of Au@Ag NPs at 37 °C for 24 h, the growth curves of (C) E. coli and (D) S. aureus obtained by culturing bacteria in broth medium containing 5 µg mL−1 Au@Ag NPs. Error bars represent the standard deviations (n ≥ 4). | |
We further studied the growth kinetics of E. coli and S. aureus in liquid media after treatment with Au@Ag NPs. The bacterial proliferation was monitored by measuring the optical density at 600 nm (OD600) within 24 h. Compared with the control group, the growth curve in the presence of Au@Ag NPs showed an extended lag phase, suggesting the inhibition of proliferation of E. coli and S. aureus. Among the three types of bimetallic Au@Ag NPs, the Au@Ag-2 NPs displayed a highest antibacterial activity during the whole time period regardless of NPs concentration and bacterial type. This result is interesting because compared with Au@Ag-3, the Au@Ag-2 NPs had lower content of Ag, yet still exhibited a significantly higher antibacterial activity than that of Au@Ag-3 NPs. We speculated that this might result from smaller size of Au@Ag-2 NPs or larger ratio of surface area over volume.33 Here, the calculated specific surface area are 0.44 for Au@Ag-1 NPs, 0.39 for Au@Ag-2 NPs and 0.21 for Au@Ag-3 NPs, respectively. Au@Ag-2 NPs have higher specific surface area and may have faster Ag+ release rate compared with larger Au@Ag-3 NPs. It has been reported that the release rate of Ag+ had a significant effect on the antibacterial efficiency of Au@Ag NPs.34
To test this hypothesis, we measured the concentration of Ag+ releasing from Au@Ag NPs solution incubated with E. coli at various time. As shown in Fig. 3, Au@Ag NPs with different sizes exhibited different release rate of Ag+. Au@Ag-1 NPs showed the fastest release rate, the concentration of Ag+ in solution reached to 0.57 µg mL−1 (maximum) after 6 h and 1.24 µg mL−1 (maximum) Ag+ was released from the Au@Ag-2 NPs after 12 h; however, though the content of silver in Au@Ag-3 NPs is the highest, they exhibited the slowest release rate of Ag+, only 1.2 µg mL−1 Ag+ was released after 24 h. Our results here demonstrate that the antibacterial activity of Au@Ag NPs is not only related to the content of silver in nanoparticles, but also related to the rate and extent of Ag+ release. Compared with the other two NPs, although the Au@Ag-2 NPs with 5 nm Ag shell thickness have a moderate concentration of Ag+, it releases the most content of Ag+ in 24 h, thus exhibiting the highest antibacterial activity during 24 h incubation. In follow-up studies, we explored antibacterial mechanisms of Au@Ag NPs with the example of Au@Ag-2 NPs since it showed the best antibacterial activities among three synthesized Au@Ag NPs.
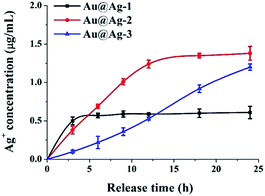 |
| Fig. 3 The cumulative Ag+ release profile of Au@Ag NPs (at 5 µg mL−1) at various time points after treatment with E. coli. | |
Synergistic antibacterial activity of gold@silver core–shell nanoparticles
In order to better understand the enhanced antibacterial activity of Au@Ag NPs, bacteria cells were treated with different concentrations of various NPs, including pure Au NPs (10 nm), pure Ag NPs (10 nm), the mixture of Au and Ag NPs (Au
:
Ag = 1
:
1), and Au@Ag-2 NPs. The percentage of dead bacteria was recorded, as shown in Fig. S4 (ESI†). Results showed that the percentage of dead bacteria of E. coli and S. aureus after treatment with Au@Ag NPs was obviously higher than that of the other NPs at the same concentration, suggesting that Au@Ag NPs have the highest antibacterial activity than that of pure Au NPs, pure Ag NPs and the simple mixture of Au and Ag NPs.
We further performed the minimum inhibitory concentration (MIC) test to quantitatively compare the antibacterial property of the Au@Ag-2 NPs and other NPs, the total metal concentrations of each NPs was calculated based on the mass ratios determined by ICP-MS. From the results shown in Table 1, it can be summarized as follows: first, the MIC for the Au@Ag-2 NPs were 10 µg mL−1 for E. coli and 15 µg mL−1 for S. aureus, which in terms of effective silver concentration were 5 µg mL−1 for E. coli and 7.5 µg mL−1 for S. aureus. These values are significantly lower than that of pure Ag NPs (15 µg mL−1 for E. coli and 20 µg mL−1 for S. aureus, respectively), indicating that Au@Ag NPs have significantly higher antibacterial activity than Ag NPs by themselves. Second, the MIC for the Au@Ag-2 NPs was much lower than that of the mixture of Au and Ag NPs, suggesting that the antibacterial activity of Au@Ag NPs is not simply the additive effects of Au NPs and Ag NPs. Third, E. coli is more sensitive to Au@Ag-2 NPs than that of S. aureus, which was attributed to the difference in the cell wall constituents between Gram-negative and Gram-positive bacteria. It has been shown that the Au@Ag NPs are more readily to penetrate and impair the thinner peptidoglycan layer in Gram-negative bacteria cells.35
Table 1 Minimum inhibitory concentrations of nanoparticles against E. coli and S. aureus
Bacterial strain |
Samples |
Concentration in MIC value (µg mL−1) |
|
|
Total |
Ag |
Au |
E. coli (ATCC25922) |
Au@Ag-2 |
10 |
5 |
5 |
Ag + Au |
30 |
15 |
15 |
Ag NPs |
15 |
15 |
— |
Au NPs |
>500 |
— |
>500 |
S. aureus (CMCC(B)26003) |
Au@Ag-2 |
15 |
7.5 |
7.5 |
Ag + Au |
36 |
18 |
18 |
Ag NPs |
20 |
20 |
— |
Au NPs |
>500 |
— |
>500 |
The Au@Ag NPs contained less content of silver, but exhibited higher antibacterial activity than Ag NPs, indicating that Ag+ is not the main contributor to highly effective antibacterial activity, though the ions definitely had impact on the overall bactericidal activity.34 Pure Ag NPs have been reported to easily oxidize and aggregate in air, which often leads to significant reduction of antibacterial activity.36 We then performed the stability test using Ag NPs and Au@Ag-2 NPs, two types of NPs were stored in light for 2 days and the UV-Vis spectra were measured. It can be noticed that the absorption peak of Ag NPs at 400 nm shifted to 417 nm with a large decrease in intensity, suggesting aggregation of Ag NPs (Fig. S5, ESI†). By contrast, the UV-Vis spectra of the Au@Ag-2 NPs changed little when stored in light for 2 days. Therefore, the Au@Ag-2 NPs are much more stable and resistant to oxidation and/or aggregation than pure Ag NPs. As we speculated, it is the outstanding stability that endows Au@Ag NPs with an excellent and a long-term antibacterial activity (Fig. S6, ESI†). Another probable reason for enhanced antibacterial activity of Au@Ag NPs may be correlated with the unique physicochemical structure of core–shell NPs. Besides, the electronic ligand effect in bimetallic NPs and the high free energy of the surface Ag atoms have been attributed to the synergistic effect with Au@Ag NPs.29,37
Disruption of bacterial cell membrane by Au@Ag NPs
To investigate the relationship between Au@Ag NPs and bacterial cell membrane damage, E. coli cells, after being treated with NPs and stained with SYTO9 and PI, were observed under confocal fluorescence microscopy. The green fluorescent dye SYTO9 stains both live and dead bacteria; but the red dye propidium iodide (PI) penetrates through only the ruptured membranes of the dead cells. Thus, the differences between the two-dye images have been widely used to distinguish dead and live bacteria and determine the integrity of the bacterial cell membranes.38 As shown in Fig. 4, the untreated E. coli was stained in only green color, indicating cells were intact and in living state. However, E. coli cells treated with either Ag NPs or Au@Ag NPs also showed red color in addition to green color, suggesting either Ag NPs or Au@Ag NPs can affect the cell membrane integrity or disrupt bacterial cell membrane. It should be noticed that the fluorescent density of PI-stained E. coli cells relative to SYTO9-stained cells in the Au@Ag NPs treatment appeared much higher than that in the Ag NPs treatment, demonstrating that the Au@Ag core–shell structure result in more dead bacterial cells and can enhance antimicrobial activity of Ag NPs. SEM images shown that the morphology of E. coli cells after treatment with Au@Ag NPs was significant changed and exhibited obvious fragmentary pieces (Fig. 5), also demonstrating that Au@Ag NPs can damage the outer membrane of E. coli.
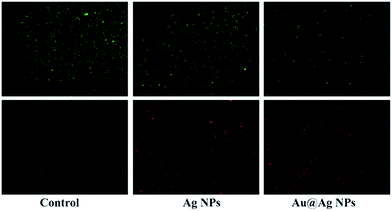 |
| Fig. 4 Fluorescence microscopy images of E. coli before and after treatment with Ag NPs and Au@Ag-2 NPs followed by staining with SYTO9 (green) and PI (red). | |
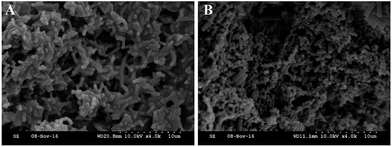 |
| Fig. 5 Scanning electron microscope (SEM) images of E. coli before (A) and after (B) treatment with Au@Ag-2 NPs for 24 h. | |
Cytotoxicity of gold@silver core–shell nanoparticles
Besides the antibacterial activities, the biocompatibility of Au@Ag NPs is another important factor in practical applications, in order to assess the toxicity effects of the nanoparticles on the cells, human neuroblastoma cell line (SH-SY5Y) was treated with various NPs at different concentration (Fig. 6). Based on a minimum cell viability of 70%,30 Ag NPs is considered to be cytotoxic at ≥10 µg mL−1, which is similar to the previous report that Ag NPs exhibited a significant cytotoxicity at >5.0 µg mL−1 to HepG2.39 However, at the same total metal ion concentration, Au@Ag NPs were more compatible than pure Ag NPs, even at a concentration up to 80 µg mL−1, we did not observe significant cytotoxicity of Au@Ag NPs to SH-SY5Y. Besides, for three types of Au@Ag NPs with different shell thickness, the order of the cytotoxicity of the core–shell NPs to SH-SY5Y cell is: Au@Ag-1 < Au@Ag-2 < Au@Ag-3, indicating that the high amount of silver is the main reason for the toxicity of Au@Ag NPs. Even so, 99% of SH-SY5Y cells remained viable after treated with 5 µg mL−1 Au@Ag-2 NPs for 24 h. These results demonstrated that the engineered Au@Ag NPs can inhibit the growth of bacteria, at the meantime, keep a great biocompatibility with human cells. We speculated that the two distinct influences of Au@Ag NPs on bacteria and human cells were attributed to the different trace of Au@Ag NPs in the mitochondria of the cells. It has been demonstrated that NPs can interact with different protein and lead to no/irreversible damage to the DNA depending on their nanostructures.25 This interesting results opens up the road for further researches on the effect of core–shell NPs at the single molecule level.
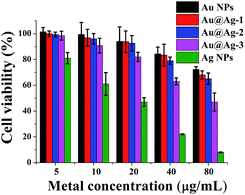 |
| Fig. 6 Cell viability assay of SH-SY5Y cell lines treated with various NPs at different concentrations for 24 h. Error bars represent the standard deviations (n = 4). | |
Conclusions
In summary, we synthesized Au@Ag NPs with varying Ag shell thickness, and investigated the influence of the shell thickness on the antibacterial activity and the cytotoxicity. Our data shown that among three thickness of NPs, the Au@Ag NPs with the shell thickness of 5 nm exhibited the strongest antibacterial activity with great biocompatibility with human cells. Most importantly, compared with individual Ag NPs and the simple mixture of Au and Ag NPs, bimetallic Au@Ag NPs with remarkable stability and a long-term antibacterial efficiency while possessed synergistically enhanced antibacterial activity against both Gram-negative and Gram-positive bacteria, even at a lower silver concentration. The result of fluorescence microscope and SEM images demonstrated that Au@Ag NPs can cause cell death by affecting the cell membrane integrity or causing cell membrane disruption. Given these advantages, we expect that Au@Ag NPs could be a promising candidate as a new bactericide in clinical and environmental applications.
Experimental section
Materials
Gold tetrachloroaurate trihydrate (HAuCl4), sodium citrate, sodium ascorbate, sodium borohydride (NaBH4), and poly(vinyl pyrrolidone) (PVP) were purchased from Sigma-Aldrich (Shanghai, China). Silver nitrate (AgNO3) and K2CO3 were purchased from Xilong Chemical Ltd (Shantou, China). Tannic acid was purchased from Ryon Biological Technology Ltd (Shanghai, China). Broth medium, Eosin-Methylene Blue agar (EMB), and Baird–Parker agar base were purchased from Hope Bio-technology Ltd (Qingdao, China). LIVE/DEAD®BacLight™ Bacterial Vibility Kit was purchased from invitrogen Ltd (America). The human neuroblastoma cell lines (SH-SY5Y) were supplied by Shengxing Biological Ltd (Nanjing, China). S. aureus (CMCC(B)26003) and E. coli (ATCC25922) were obtained from Huankai microbial sci. & tech. Co., Ltd (Guangdong, China). All other chemicals and reagents were obtained from either Sigma-Aldrich (Shanghai, China) or Sinopharm Chemical Reagent Ltd (Beijing, China).
Synthesis of silver nanoparticles
Silver nanoparticles were synthesized using a standard procedure by reduction of silver nitrate.40 Ag NPs with a diameter of 10 ± 3 nm were synthesized following the previously described method with some modifications. Briefly, 0.6 g of NaBH4 was dissolved in 20 mL of distilled water (ice-cold), and then 5 mL of 1% poly(vinyl pyrrolidone) (PVP) was added as stabilizer (protecting agent). The solution was kept in a water-ice bath with high-speed stirring. Next, 5 mL of 1% PVP and 5 mL of 10 mM AgNO3 were added to the solution prepared beforehand simultaneously by two constant-flow pumps at the rate of 30 mL h−1. The final mixture was kept at 80 °C for 3 h to remove the unreacted NaBH4. The finished sample was yellow and was stored at 4 °C.
Synthesis of gold nanoparticles
Au NPs were synthesized according to a previously reported procedure.30 Au NPs (10 ± 2 nm) were synthesized by sodium citrate–tannin reduction. First, 1 mL of HAuCl4 (1% by weight) was added into 79 mL of Millipore-Q water under stirring; the mixture was prepared as solution A. Second, 4 mL of sodium citrate (1% by weight), 0.1 mL of tannic acid (1% by weight), and 0.1 mL of K2CO3 (25 mM) were added into 15.8 mL of Millipore-Q water; the sample was mixed under stirring and named as solution B. Third, solutions A and B were separately heated to 60 °C for 30 min, and then solution B was quickly added into solution A under high-speed stirring. The solution was kept at 60 °C for 30 min until the color turned reddish orange and stable. Then the reddish orange solution was cooled to room temperature and stored at 4 °C.
Synthesis of gold@silver core–shell nanoparticles
Au@Ag NPs were prepared according to the procedure with some modification.31 50 µL of 5-fold concentrated Au NPs were dispersed into a solution containing 200 µL Millipore-Q water, 100 µL 1% PVP, and 50 µL 0.1 M sodium ascorbate. Different concentrations (3.5 mM, 7 mM, or 15 mM) of 10 µL AgNO3 solution were added and synthesized nanoparticles were named as Au@Ag-1, Au@Ag-2, and Au@Ag-3 NPs, respectively. The reaction solution was shaken for 3 h at room temperature and then was centrifuged at 12
000 rpm for 10 min. The precipitate was washed three times and resuspended in Millipore-Q water.
Antimicrobial activity assays
S. aureus (CMCC(B)26003) and E. coli (ATCC25922) were cultured in broth medium in a shaking incubator at 37 °C overnight and the bacteria were harvested by centrifugation at 6000 rpm for 10 min at 4 °C and washed with sterile PBS.
The antibacterial activities of nanoparticles against E. coli and S. aureus were evaluated using a cell counting method. Pre-prepared nanomaterials (1.2–5 µg mL−1) were added to bacteria (106 CFU mL−1) and incubated at 37 °C with shaking at 200 rpm for 24 h. A 0.9% NaCl solution with bacteria was used as the positive control. The samples were diluted serially 10-fold before spotting onto Baird–Parker and EMB agar plates. After an overnight incubation at 37 °C, the CFUs were counted and used to determine cell survival. The dead bacterial was calculated as (A − B)/A × 100 (where A is the number of surviving bacteria colonies in the control and B is that in the sample). Every experiment was repeated four times.
The growth curve was determined by measuring the time evolution of the optical density (OD600). S. aureus and E. coli (106 CFU mL−1) in the broth medium were incubated with 5 µg mL−1 Au@Ag NPs, respectively. The mixture of sterile water and bacterial solution was prepared as the control experiment. The bacterial growth rates were determined over a predefined time course by measuring the optical density (OD) at 600 nm by a UV-Vis spectrophotometer (UV-2600, Shimadzu).
For the minimum inhibitory concentration (MIC), bacterial cells were suspended in sterile broth medium to obtain a concentration of 106 CFU mL−1. Then, each type of nanoparticles (Au NPs, Ag NPs, Au@Ag-2 NPs and the mixture of Au and Ag) was added to bacteria solutions and shaken at 37 °C for 24 h. Each NPs sample was tested at three times for each concentration along with triplicate positive (broth and bacteria) and negative (broth) controls. The lowest concentration at which there was no visual turbidity was taken as the MIC value of NPs.
Measurement of ion release
5 µg mL−1 nanoparticles were added to 106 CFU mL−1 E. coli and incubated at 37 °C. After certain time, the mixture was centrifuged at 15
000 rpm for 20 min at 4 °C to remove the bacterial cells and nanoparticles. The concentration of Ag+ in the supernatant was determine by inductively coupled plasma mass spectrometry (ICP-MS) (Bruker-M90).
Live/dead bacterial assay
Live/dead bacterial assay was carried out to examine the effect of nanoparticles on the integrity of bacterial cell membranes.41 10 µg mL−1 Au@Ag NPs were added to 107 CFU mL−1 bacterial cells, the mixture was incubated at 37 °C for 24 h. Then the solutions were mixed with 0.5 mL of dye solution containing 1 µM SYTO9 and 5 µM propidium iodide (PI) for 20 min at room temperature. The bacteria cells were imaged using a confocal laser scanning microscope (TCS-SP2, Leica, Germany).
Cytotoxicity assay
The cell viability of human neuroblastoma cell line (SH-SY5Y) treated with each nanoparticles was measured using an MTT assay.42 100 µL of cells solution was added into a 96-well plate at a density of 5000 cells per plate and incubated at 37 °C, 5% CO2 for 24 h. Then, cells were treated with various concentrations of each NPs solution and incubated for an additional 24 h. In parallel, cells with the addition of water served as negative controls. Then, 50 µL 0.5 mg mL−1 MTT bromide aqueous solution was added to each well of a 96-well plate. Following 4 h incubation, the medium was removed and formazan crystals were solubilized by incubation in 100 µL of DMSO for 10 min. Finally, the absorbance of each well was measured at a wavelength of 570 nm on a microplate reader (Synergy HT, BIO-TEK). The percent of cell viability was determined by the absorbance of the test sample divided by the absorbance value of the positive control.
Acknowledgements
This work is financially supported by the National Natural Science Foundation of China (Grants 31601569), the Fundamental Research Funds for the Central Universities (Grants KJQN201731), the China Postdoctoral Science Foundation (Grants 2016M600420), and the National Science & Technology Pillar Program (Grants 2015BAD16B05).
References
- D. M. Eby, N. M. Schaeublin, K. E. Farrington, S. M. Hussain and G. R. Johnson, ACS Nano, 2009, 3, 984–994 CrossRef CAS PubMed.
- J. B. Fei, J. Zhao, C. L. Du, A. H. Wang, H. Zhang, L. R. Dai and J. B. Li, ACS Nano, 2014, 8, 8529–8536 CrossRef CAS PubMed.
- B. Le Ouay and F. Stellacci, Nano Today, 2015, 10, 339–354 CrossRef CAS.
- O. Pandoli, R. D. S. Martins, E. C. Romani, S. Paciornik, M. Mauricio, H. D. L. Alves, F. V. Pereira-Meirelles, E. L. Luz, S. M. L. Koller, H. Valiente and K. Ghavami, RSC Adv., 2016, 6, 98325–98336 RSC.
- I. Sondi and B. Salopek-Sondi, J. Colloid Interface Sci., 2004, 275, 177–182 CrossRef CAS PubMed.
- X. Yang, A. P. Gondikas, S. M. Marinakos, M. Auffan, J. Liu, H. Hsu-Kim and J. N. Meyer, Environ. Sci. Technol., 2012, 46, 1119–1127 CrossRef CAS PubMed.
- G. A. Sotiriou, A. Meyer, J. T. Knijnenburg, S. Panke and S. E. Pratsinis, Langmuir, 2012, 28, 15929–15936 CrossRef CAS PubMed.
- J. Y. Liu, D. A. Sonshine, S. Shervani and R. H. Hurt, ACS Nano, 2010, 4, 6903–6913 CrossRef CAS PubMed.
- A. Taglietti, Y. A. Diaz Fernandez, E. Amato, L. Cucca, G. Dacarro, P. Grisoli, V. Necchi, P. Pallavicini, L. Pasotti and M. Patrini, Langmuir, 2012, 28, 8140–8148 CrossRef CAS PubMed.
- J. A. Lemire, J. J. Harrison and R. J. Turner, Nat. Rev. Microbiol., 2013, 11, 371–384 CrossRef CAS PubMed.
- A. Ivask, A. Elbadawy, C. Kaweeteerawat, D. Boren, H. Fischer, Z. Ji, C. H. Chang, R. Liu, T. Tolaymat, D. Telesca, J. I. Zink, Y. Cohen, P. A. Holden and H. A. Godwin, ACS Nano, 2014, 8, 374–386 CrossRef CAS PubMed.
- S. George, S. Lin, Z. Ji, C. R. Thomas, L. Li, M. Mecklenburg, H. Meng, X. Wang, H. Zhang, T. Xia, J. N. Hohman, S. Lin, J. I. Zink, P. S. Weiss and A. E. Nel, ACS Nano, 2012, 6, 3745–3759 CrossRef CAS PubMed.
- O. Bar-Ilan, R. M. Albrecht, V. E. Fako and D. Y. Furgeson, Small, 2009, 5, 1897–1910 CrossRef CAS PubMed.
- S. Kittler, C. Greulich, J. Diendorf, M. Koller and M. Epple, Chem. Mater., 2010, 22, 4548–4554 CrossRef CAS.
- J. Song, H. Kim, Y. Jang and J. Jang, ACS Appl. Mater. Interfaces, 2013, 5, 11563–11568 CAS.
- I. Ocsoy, M. L. Paret, M. A. Ocsoy, S. Kunwar, T. Chen, M. X. You and W. H. Tan, ACS Nano, 2013, 7, 8972–8980 CrossRef CAS PubMed.
- A. Naskar, S. Bera, R. Bhattacharya, P. Saha, S. S. Roy, T. Sen and S. Jana, RSC Adv., 2016, 6, 88751–88761 RSC.
- J. L. Chen, M. L. Mei, Q. L. Li and C. H. Chu, RSC Adv., 2016, 6, 104025–104035 RSC.
- K. K. Haldar, S. Kundu and A. Patra, ACS Appl. Mater. Interfaces, 2014, 6, 21946–21953 CAS.
- J. Xu, A. R. Wilson, A. R. Rathmell, J. Howe, M. Chi and B. J. Wiley, ACS Nano, 2011, 5, 6119–6127 CrossRef CAS PubMed.
- S. Liu, Z. Sun, Q. Liu, L. Wu, Y. Huang, T. Yao, J. Zhang, T. Hu, M. Ge, F. Hu, Z. Xie, G. Pan and S. Wei, ACS Nano, 2014, 8, 1886–1892 CrossRef CAS PubMed.
- L. Tang, S. Li, L. Xu, W. Ma, H. Kuang, L. Wang and C. Xu, ACS Appl. Mater. Interfaces, 2015, 7, 12708–12712 CAS.
- W. Feng, X. Zhou, W. Nie, L. Chen, K. Qiu, Y. Zhang and C. He, ACS Appl. Mater. Interfaces, 2015, 7, 4354–4367 CAS.
- L. Zeng, Y. Pan, S. Wang, X. Wang, X. Zhao, W. Ren, G. Lu and A. Wu, ACS Appl. Mater. Interfaces, 2015, 7, 16781–16791 CAS.
- M. Mahmoudi and V. Serpooshan, ACS Nano, 2012, 6, 2656–2664 CrossRef CAS PubMed.
- C. Fasciani, M. J. Silvero, M. A. Anghel, G. A. Arguello, M. C. Becerra and J. C. Scaiano, J. Am. Chem. Soc., 2014, 136, 17394–17397 CrossRef CAS PubMed.
- X. Dai, Z. Fan, Y. Lu and P. C. Ray, ACS Appl. Mater. Interfaces, 2013, 5, 11348–11354 CAS.
- H. Alishah, S. Pourseyedi, S. E. Mahani and S. Y. Ebrahimipour, RSC Adv., 2016, 6, 73197–73202 RSC.
- M. Banerjee, S. Sharma, A. Chattopadhyay and S. S. Ghosh, Nanoscale, 2011, 3, 5120–5125 RSC.
- J. D. Padmos, M. Langman, K. MacDonald, P. Comeau, Z. Yang, M. Filiaggi and P. Zhang, J. Phys. Chem. C, 2015, 119, 7472–7482 CAS.
- Y. Zhao, L. Xu, W. Ma, L. Wang, H. Kuang, C. Xu and N. A. Kotov, Nano Lett., 2014, 14, 3908–3913 CrossRef CAS PubMed.
- R. Wang, Y. Yao, M. Shen and X. Wang, Colloids Surf., A, 2016, 492, 263–272 CrossRef CAS.
- V. R. Chelli and A. K. Golder, RSC Adv., 2016, 6, 95483–95493 RSC.
- Z. M. Xiu, Q. B. Zhang, H. L. Puppala, V. L. Colvin and P. J. Alvarez, Nano Lett., 2012, 12, 4271–4275 CrossRef CAS PubMed.
- H. Y. Wang, X. W. Hua, F. G. Wu, B. Li, P. Liu, N. Gu, Z. Wang and Z. Chen, ACS Appl. Mater. Interfaces, 2015, 7, 7082–7092 CAS.
- M. Lv, S. Su, Y. He, Q. Huang, W. Hu, D. Li, C. Fan and S. T. Lee, Adv. Biomater., 2010, 22, 5463–5467 CAS.
- Y. M. Zhai, L. Han, P. Wang, G. P. Li, W. Ren, L. Liu, E. K. Wang and S. J. Dong, ACS Nano, 2011, 5, 8562–8570 CrossRef CAS PubMed.
- Q. Cai, Y. Gao, T. Gao, S. Lan, O. Simalou, X. Zhou, Y. Zhang, C. Harnoode, G. Gao and A. Dong, ACS Appl. Mater. Interfaces, 2016, 8, 10109–10120 CAS.
- K. Kawata, M. Osawa and S. Okabe, Environ. Sci. Technol., 2009, 43, 6046–6051 CrossRef CAS PubMed.
- W. Yan, L. Xu, C. Xu, W. Ma, H. Kuang, L. Wang and N. A. Kotov, J. Am. Chem. Soc., 2012, 134, 15114–15121 CrossRef CAS PubMed.
- I. Irwansyah, Y. Q. Li, W. Shi, D. Qi, W. R. Leow, M. B. Tang, S. Li and X. Chen, Adv. Biomater., 2015, 27, 648–654 CAS.
- M. C. Wu, A. R. Deokar, J. H. Liao, P. Y. Shih and Y. C. Ling, ACS Nano, 2013, 7, 1281–1290 CrossRef CAS PubMed.
Footnote |
† Electronic supplementary information (ESI) available: Characterization data for TEM, size distribution by DLS, UV-Vis spectra, visual color change of NPs; ICP-MS results for Au@Ag NPs; percentage of dead bacteria of bacteria after treatments with NPs, absorption spectra of NPs used for stability comparison; antibacterial assessment of different nanoparticles solution. See DOI: 10.1039/c7ra00485k |
|
This journal is © The Royal Society of Chemistry 2017 |
Click here to see how this site uses Cookies. View our privacy policy here.