DOI:
10.1039/C7RA00357A
(Paper)
RSC Adv., 2017,
7, 15005-15019
Effect of gasoline–bioethanol blends on the properties and lubrication characteristics of commercial engine oil
Received
10th January 2017
, Accepted 27th February 2017
First published on 7th March 2017
Abstract
Concerns over depleting fossil fuel reserves, energy security, and climate change have resulted in stringent legislation demanding that automobiles use more renewable fuels. Bioethanol is being given significant attention on a global scale and is being considered as a long-term gasoline replacement that helps reduce exhaust emissions. The piston ring and cylinder wall interface is generally the largest contributor to engine friction and these regions of the engine also suffer the highest levels of fuel dilution into the lubricant from unburned fuel, especially for bioethanol as it has a high heat of vaporization, which enhances the tendency of the fuel to enter the oil sump. As bioethanol is being blended with gasoline at increasingly higher concentrations and the accumulation of fuel in the crankcase is significant, it is crucial to study the effect of various bioethanol blends on the degradation of engine oil's properties and the friction and wear characteristics of engine oil. A fully synthetic oil was homogenously mixed with five formulated fuels such as gasoline blend (E0), gasoline–10% ethanol (E10), gasoline–20% ethanol (E20), gasoline–30% ethanol (E30), and gasoline–85% ethanol (E85). These mixtures were then tested in a four-ball wear tester according to the ASTM D4172 standard test. Under selected operating conditions, the results show that the addition of a gasoline–bioethanol blend decreases the oil viscosity, whereas the acid number increases because bioethanol is more reactive compared to gasoline, which enhances oil degradation and oxidation. Fuel dilution reduces the lubricating efficiency and the wear protection of the engine oil. All fuel-diluted oil samples have higher friction and wear losses, compared to the fresh synthetic oil. E10 has slight effects on the friction and wear behaviors of the engine oil. Thus, it still has a high potential to be widely used as a transportation fuel for existing gasoline engines.
1. Introduction
Fossil fuels such as diesel and gasoline which are non-renewable energy resources, are being depleted day by day. It is believed that these conventional fuels will become scarce during next several decades.1,2 To reduce the concern over this undesirable issue, researchers have been putting in much effort to reduce the use of fossil fuels by using alternative biofuels, in particular biodiesel and bioethanol fuels, which are being blended with diesel and gasoline at increasingly higher concentrations. USA is now the leading producer of bioethanol in the world, whereby 18.3 billion liters of bioethanol was produced by the USA in 2006.3 Nowadays most vehicles are fuelled with 10–15% ethanol, and the so-called ‘biofuel’ engines are typically designed to run on pure hydrated ethanol (93% ethanol, 7% water) or E85 blends which are made up of 85% ethanol and 15% gasoline. Interestingly, in Brazil, flex fuel vehicles (FFV) have been manufactured to use with any bioethanol–gasoline blends.4 This can enhance energy independence whilst combating fundamental issues such as fossil depletion, oil price escalation, carbon emission, and particulate mass concentrations in the vehicle exhaust.5,6 According to the National Association of Automotive Vehicle Manufacturers, more than 85% of flex-fuel vehicles are manufactured in Brazil.7 Both Sweden and Belgium aim to increase the use of bioethanol as a transportation fuel.8,9 More importantly, ethanol has become one of the alternative fuels of interest in Sweden ever since the 1990s. The annual use of ethanol was 65
000 m3 in 2001. About 3% of the public service bus fleet (more than 400 buses) use ethanol as fuel, and about 4450 flex-fuel vehicles run on E85 blends.10
However, the bioethanol employment also poses some undesirable issues. Firstly, bioethanol is miscible with water which can cause a corrosive effect on engine components such as fuel injector and electric fuel pump.4,11 Moreover, bioethanol attracts more water from the environment because it is hygroscopic in nature.12 Secondly, vehicle start-up problem can happen in cold weather when the engine is fuelled with pure ethanol which is hard to vaporize.13 Thirdly, when bioethanol is used to fuel the engine, the tribological effect on lubricant properties and performance resulting from fuel dilution always appears. During the combustion process, some amount of unburned fuel will impinge on the cold wall of the combustion chamber and then be scrapped into the crankcase of engine oil through cylinder liner.14 It shall be notified that the impact of bioethanol on lubricating oil's properties and performance is completely different from that of gasoline due to the fact that bioethanol has a higher tendency to enter the oil sump of an engine due to its high heat of evaporation compared to gasoline.11 The amount of bioethanol inside lubricant can degrade the properties and performance of engine oil significantly. As mentioned earlier, bioethanol is miscible with water but immiscible with oil, so that there would be the formation of emulsions inside bioethanol–water–oil mixture, which leads to serious engine wear and catastrophic engine failure.15 As a consequence, engine oil needs to be drained on a frequent basis. It has been observed that even a small amount of fuel dilution is possible to degrade the physicochemical properties of lubricant (viscosity, total base number, and total acid number) which play an important role in the lubricating system.16,17
In gasoline engines, various sliding components produce more friction between metal-to-metal contact surfaces, especially the piston assembly consisting of the piston ring and cylinder liner which contribute to engine friction to approximately 40–60%.18 This friction, in turn, affects engine durability as well as fuel consumption. Therefore, various methods are used to improve the performance of automotive engines such as coatings,19 laser texturing,20–23 reducing the weight of components and modifying the composition of automotive lubricants.24 Thus engine oil having high lubricity is needed to reduce friction and wear in order to extend engine life. Synthetic oil is widely used as lubricating oil in most vehicle engines due to its high thermal and oxidative stability, alkalinity, and viscosity index. It has a strong film strength which can help improve its frictions behavior in long operation.25–27
1.1. Objective of this study
Although bioethanol has been widely used as an alternative fuel, it is deemed important to look at its effects on the tribological characteristics of engine oil. This current work aims to investigate the oil degradation, and lubricating efficiency (friction and wear losses) of fully-synthetic oil diluted with various bioethanol–gasoline blends. It is believed that this paper will provide useful insights of the current work pertaining to fuel dilution so that practitioners and researchers can select the optimum gasoline–bioethanol blend which has a slight lubricating effect on the engine and engine oil.
2. Literature review
The study of bioethanol dilution is limited and its effect on lubricating oil is not clearly established. However, there is some literature in this field as described in Table 1. Mostly, it has been observed that the use of ethanol with fossil fuel can cause a significant effect on automotive lubricant's properties such a drop in engine oil viscosity, and total base number (TBN), as well as an increase in total acid number (TAN), and an effect on oil performance such as an increase in friction, wear losses and deposit formation resulting from oxidation and corrosion of bioethanol.28 The addition of bioethanol to gasoline enhances the tendency of the fuel to reach the crankcase of engine oil due to its higher heat of vaporization, thus increasing the rate of fuel dilution.11,29 Significant amounts of diluted ethanol fuel, in particular, E85 (between 6% and 25%) have been found in the engine oil crankcase after field tests12 and bench sequence tests.11,30 A study of fuel dilution was done by T. Hu et al.31 They explained that turbocharged gasoline direct-injection (TGDI) engine operated at high torque had a significant influence on fuel dilution (gasoline) which was found to be up to 9%. This percentage caused a decrease in oil viscosity, an increase in fuel consumption and the formation of carbon on the piston ring area. Tippayawong and Sooksarn32 investigated into the lubricating performance of lubricant diluted with ethanol and gasoline. They prepared four combinations of fuels and lubricants to be tested with motorcycle engine: (1) gasoline and mineral oil, (2) gasoline and synthetic oil, (3) 5% ethanol and mineral oil and (4) 5% ethanol and synthetic oil. The results indicated that viscosity of all oil samples decreased with operating time. After 3000 km mileage, the viscosity changed about 20% and 45% for synthetic-based and mineral-based oil, respectively. Mineral oil produced larger scar areas, compared to synthetic oil. For the same type of engine oil, the wear scar areas from the gasohol-run engine were about 10% larger than those from the gasoline-run engine. It also explains that lubricant properties changed with operating time because of additive depletion, oxidation, thermal degradation, reaction with sliding surface and engine blow-by contamination. Another experiment on friction and wear characteristics was conducted by Ajayi et al.33 They diluted lubricant with three different fuels: gasoline (E0), gasoline–10% ethanol (E10), gasoline–16% isobutanol (i-B16) and used with a marine machine which was operated under start-and-stop and on-water conditions. It was shown that ethanol showed the least amount of fuel dilution due to its high evaporation while i-B16 showed the highest rate of fuel dilution. It is also notified that high engine speed enhanced fuel dilution, leading to a significant decrease in oil viscosity as well as producing more wear. However, friction was slightly reduced probably due to the drop in viscosity. Costa and Spikes17 investigated the effect of temperature on the lubricating performance of fully-formulated oil contaminated with 5% of both anhydrous and hydrated ethanol fuels and a solution of ZDDP antiwear additive. They found that this addition destroyed the pre-formed antiwear tribofilm and deteriorated the rubbing surface.
Table 1 Previous studies of lubricant diluted with fuel contaminant
Ref. |
Methods/material |
Conditions |
Results |
32 |
Engine testing |
Mileage: 3000 km |
Viscosity falls up to 45% |
Bench wear tester, mineral oil, synthetic oil, gasoline/gasohol |
Load: 33 N, speed: 500 rpm, Temp: 25 °C |
Gasohol engine has 10% higher wear, mineral oil: high wear, synthetic oil: wear increased, friction (unknown) |
33 |
Engine testing |
Load: 15 N |
Approx. 3.7–6% fuel dilution |
Unidirectional sliding, reciprocating sliding, four-ball wear test, gasoline, ethanol, isobutanol, SAE 10W-30 |
Velocity: 0.1–20.0 cm s−1, speed: 10–300 rpm, frequency: 0.1–5 Hz, four-ball load: 15 kg, speed: 1200 rpm, Temp: 70 °C, time: 1 h |
E0: viscosity 30–43% decreased, E10: viscosity 25–42% decreased, i-B16: viscosity 28–46% decreased, all fuels have slight effect on friction, wear protection of the oil reduced with fuel dilution |
15 |
Plint TE77 |
Duration: 2 h |
Friction reduction when tested with |
Shell Helix HX7, SAE 5W-30, no friction modifier, ethanol 95% purity, water |
Liner T: 70–110 °C, test Temp: 25–40 °C, speed: 2000 rpm, load: 150 N (4 MPa), Stroke length: 5 mm, cold-start/short-journey |
Separated phase of ethanol–water–oil mixture, viscosity decreased, implication: fuel economy in a fired gasoline engine |
34 |
Tribotest (ball on discs) |
Duration: 20 min |
No effect on friction significantly |
Ball: AISI 52100, discs: AISI H13, engine oil: SAE 5W30, E22, (E100, 7% water) |
Test sample: 3 ml, Temp: 40 °C, load: 35 N, Stroke length: 5 mm, frequency: 10 Hz, Max. speed: 0.159 m s−1 |
Aged oil showed FC value similar to those obtained from base oil. Viscosity and TAN analysis show that E22 is more oxidized and may cause oxidative wear |
35 |
Ball on disk wear test |
Dilution rate: 5 wt% |
Tribofilm thickness was reduced by the addition of ethanol to both oils. Anhydrous ethanol caused more film thickness reduction than hydrated ethanol. Tribofilm was allowed to form during rubbing using an ethanol-free oil. Hydrated destroyed pre-formed antiwear tribofilm and rubbed surface |
A sliding/rolling contact of ball and disc, ball: AISI 52100 steel, disc: AISI 52100 steel, fully formulated oil with 0.08 wt% ZDDP, hydrated ethanol & anhydrous ethanol |
Speed: 3500 mm s−1, reduce 25 steps down to 7 mm s−1, load: 31 N, Hertz contact pressure: 0.95 GPa, contact diameter: 250 μm, slide-to-roll ratio: 50% |
36 |
Modified Plint TE77 |
Cold-start: dilution (5 wt% ethanol + 8 wt% water) |
Interaction between ethanol and water contributes significantly to friction, independently. Ethanol does not significantly impact on friction reduction. Interaction (water & temperature) has a small impact on friction reduction |
Taguchi method, engine oil: SAE 5W30 Shell Helix HX7, ethanol: 95% purity, distilled water, real piston ring and cylinder liner |
Temp: 25 °C; load: 100 N, time: 2 h; speed: 1500 rpm, warm-up: dilution (10 wt% ethanol + 16 wt% water), Temp: 40 °C; load: 150 N, time: 2 h; speed: 2000 rpm |
37 |
Minitraction machine ball-on-disc test, base oil (additive), fully formulated oil without additive, hydrated ethanol and anhydrous ethanol |
Dilution rate: 5 wt%, speed: 1000 mm s−1, reduce 31 steps down to 1 mm s−1, load: 20 N; Temp: 40 °C, 70 °C, 100 °C, Hertz pressure: 0.82 GPa |
At low speed, the addition of ethanol produces a boundary film, which was not present in the base oils, but the addition of ethanol to formulated oil reduced film thickness in all lubrication regimes, and ethanol reduces friction at higher speed |
3. Methodology
3.1. Material used
Fully-synthetic oil (SO, SAE 5W40) was purchased from the market in Malaysia. This lubricant is mostly used under the most heavy-duty condition. Table 2 shows the properties of the engine oil. Gasoline and pure bioethanol which were also collected from Malaysian market were added to the fully-synthetic oil. The properties of gasoline and bioethanol are shown in Table 3.
Table 2 The physicochemical properties of fully-synthetic oil (SO, SAE 5W40)
Oil parameter |
Units |
Value |
ASTM method |
Density 15 °C |
kg l−1 |
0.854 |
D4052 |
Viscosity 40 °C |
mm2 s−1 |
82.03 |
D445 |
Viscosity 100 °C |
mm2 s−1 |
13.2 |
D445 |
Viscosity index, VI |
— |
171 |
D2270 |
Total base number, TBN |
mg KOH per kg |
9.1 |
D2896 |
Total acid number, TAN |
mg KOH per kg |
1.4 |
D664 |
Table 3 The properties of gasoline and ethanol
Properties |
Units |
Gasoline (RON 95) |
Ethanol |
Density 15 °C |
g cm−3 |
0.750 |
0.799 |
Kinematic viscosity 15 °C |
mm2 s−1 |
0.542 |
1.713 |
Kinematic viscosity 20 °C |
mm2 s−1 |
0.529 |
1.478 |
Boiling point |
°C |
35 |
78 |
3.2. Test sample preparation
Formulated fuels such as gasoline (E0), gasoline–10% v/v bioethanol (E10), gasoline–20% v/v bioethanol (E20), gasoline–30% v/v bioethanol (E30), and gasoline–85% v/v bioethanol (E85) were blended at a fixed volume of 100 ml using shaking machine. The properties of each gasoline–bioethanol blends were measured and shown in Table 4. Each fuel blend was then added to fully-synthetic oil at the dilution rate of 6% v/v in order to prepare fuel–oil samples for the test (i.e. E10–SO = 10% v/v bioethanol fuel + 90% v/v synthetic oil). 6% v/v concentration is selected because the permissible dilution rate of fuel in engine oil is limited to 4% for a gasoline engine. This study aims to investigate the effect on lubrication performance of engine oil at this 6% v/v dilution. To obtain well-blended test samples, each fuel–oil sample was mixed using magnetic stirrer at an appropriate volume of 100 ml. The shaker and stirrer were continuously blending the fuel–oil mixtures for 30 min, at the speed of 400 rpm and at ambient temperature.
Table 4 The physicochemical properties of bioethanol–gasoline blended fuels
Properties |
Unit |
E10 |
E20 |
E30 |
E85 |
Density at 15 °C |
kg m−3 |
0.754 |
0.758 |
0.763 |
0.788 |
Kinematic viscosity at 15 °C |
mm2 s−1 |
0.576 |
0.647 |
0.748 |
1.411 |
Kinematic viscosity at 20 °C |
mm2 s−1 |
0.546 |
0.614 |
0.705 |
1.292 |
3.3. Properties of fuel–oil blends
In order to observe oil degradation by fuel dilution, the physicochemical properties of fuel diluted lubricant such as density, viscosity, viscosity index (VI), acid number were measured by using instruments mentioned in Table 5. All measurements of the properties were done according to ASTM standard methods. Table 6 indicates the properties of synthetic oil diluted with 6% v/v of gasoline–bioethanol blends. Stabinger viscometer was used to measure viscosity index, density at 15 °C and kinematic viscosity at 40 °C and 100 °C. The acid number were measured using TAN analyzers. Flash temperature parameter was determined by using the formula mentioned in Section 3.6. The values of flash temperature parameter (FTP) of each sample at 40 kg and 80 kg are also shown in Table 6.
Table 5 List of equipment for fuel–oil properties measurement
Properties |
Equipment |
Manufacturer |
Accuracy |
Density |
Stabinger viscometer |
Anton Paar, UK |
±0.1 kg m−3 |
Kinematic viscosity |
Stabinger viscometer |
Anton Paar, UK |
±0.1 mm2 s−1 |
Viscosity index |
Stabinger viscometer |
Anton Paar, UK |
±0.1 |
Acid number |
TAN analyzer |
Metrohm |
±0.001 mg KOH per g |
Table 6 The physicochemical properties of each fuel–oil blend, NM = not measurable
Test sample |
Viscosity (mm2 s−1) |
VI |
Density (g cm−3) 15 °C |
Acid number (mg KOH per g) |
FTP |
40 °C |
100 °C |
40 kg |
80 kg |
SO only |
82.03 |
13.2 |
171 |
0.8540 |
1.4 |
178.19 |
33.96 |
E0–SO |
58.52 |
10.99 |
183 |
0.8517 |
2.11 |
155.00 |
33.10 |
E10–SO |
56.63 |
10.86 |
182 |
0.8517 |
2.17 |
155.58 |
29.38 |
E20–SO |
57.14 |
N.M. |
N.M. |
0.8517 |
2.28 |
156.74 |
31.57 |
E30–SO |
57.69 |
N.M. |
N.M. |
0.8523 |
2.36 |
152.76 |
30.74 |
E85–SO |
62.45 |
N.M. |
N.M. |
0.8524 |
2.89 |
145.29 |
28.41 |
3.4. Friction test and procedure
Fig. 1 illustrates the schematic diagram of the four-ball wear tester. Four-ball wear tester (TR-30H) was used to investigate friction and wear characteristics of fuel diluted synthetic oil. This test is one of the ASTM standards (D4172) for evaluating the performance of lubricant under relatively severe sliding contact under boundary condition.33 Referring to the Stribeck curve, there are a few main sliding engine components (piston ring/cylinder liner, valve train, and camshaft) which mainly or partly experience boundary lubrication regime.38 The balls used in this experiment were chrome alloy steel balls, AISI 52-100 standard, 64-66 Rc hardness, diameter (12.7 mm) and surface roughness (0.1 μm, C.L.A: Center Line Average). The 52100 steel is a common material used for internal combustion engine (ICE) piston rings. To set up each test, the test balls were cleaned by using toluene and wiped by using a neat tissue to make them completely dry. Three test balls were placed into the oil cup and a fuel–oil sample was poured into the cup until it fully covered the balls. In this four-ball test, three balls in the oil cup were kept stationary and another ball was rotating in contact with these stationary balls. Four-ball machine was performed under conditions (time: 60 min, temperature: 75 °C, load: 40 kg and 80 kg, and rotational speed: 1200 rpm). The reason for choosing these conditions is due to the fact that the parameters closely response to speed, load, and temperature of the piston-ring assembly conditions.39,40 The normal temperature of engine oil during warm-up and short-journey driving condition is 40–80 °C. Therefore, to prevent frictional heating and to reduce the evaporation of bioethanol which boils off quickly at the temperature above the boiling point (78 °C), the test was performed at 75 °C. After completing the test, frictional data, and the stationary balls were collected for friction coefficient and wear scar diameter (WSD) evaluations, respectively. After collecting all the data, the test was started over again using the same procedure.
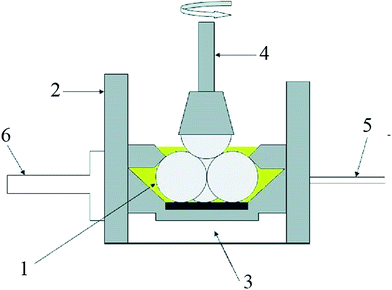 |
| Fig. 1 The schematic diagram of the four-ball wear machine [1: stationary balls, 2: steel cup, 3: heating zone, 4: rotating arm, 5: thermocouple, 6: torque arm]. | |
3.5. Friction and wear evaluations
The friction coefficient (FC) can be evaluated from frictional torque and spring constant.41 In four-ball wear test, the lower balls create the maximum torque which is determined by a load cell. The eqn (1) is used to calculate the friction coefficient: |
 | (1) |
where, T, W, and r can be expressed as friction torque (kg × mm), applied load (kg) and distance (mm), respectively. The distance (r) measured from the center of the lower ball contact surface to the rotating axis is 3.67 mm.
According to the ASTM D4172 method, wear scar diameter (WSD) produced on the three tested balls was measured by optical microscope model C2000 (IKA, UK) with ±0.01 mm accuracy. To obtain a clear wear scar image, the magnifying lens was adjusted to a better position on the wear scar surface of the ball. After measuring the wear scar diameter of those three balls, the arithmetic average result was calculated between them.
3.6. Flash temperature parameter (FTP) calculation
Flash temperature parameter (FTP) is a number used to express the critical temperature above which an engine oil will fail under selected conditions.42 The lubricating oil has failed at the critical flash temperature. The FTP also refers to the possibility of lubricant breakdown.43 The higher the FTP indicates the less possibility of lubricant film to breakdown. This flash temperature can be measured by the thermocouple and expressed by a solitary number of FTP. The discussion of FTP number prediction model was discussed in ref. 44. For conditions existing in four-ball machine, the FTP was calculated using the following relationship:45 |
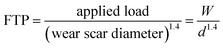 | (2) |
where, W expresses applied load (kg), d expresses wear scar diameter (mm), FTP is the maximum value of the ratio W/d1.4 obtained from various run. It shall be notified that the FTP (kg mm−1.4) relationship is expressed as a number (maximum value) in most previous studies42,46 and therefore, the FTP (kg mm−1.4) is also considered as unit less in this study.
3.7. Surface analysis
The wear morphology of the tested ball was analyzed accordingly using a scanning electron microscope (SEM) which is the most known instrument for the surface analytical techniques. High-resolution images of surface topography, with excellent depth of field, were taken using a highly-focused, scanning (primary) electron beam. Three areas of wear scar surfaces were captured and discussed respectively to prove friction and wear behaviors of each fuel diluted synthetic oil.
3.8. Error analysis
Error analysis was calculated to determine the level of accuracy and uncertainty of the collected data due to the fact that, during the experiment, there might be errors arising from instrument selection, testing condition, environmental condition, observation, calibration and reading and collecting data.47 The test was repeated three times, and the collected data was averaged and performed through graph plotting and precision measuring. The accuracy for WSD and FC are ±0.01 mm and ±0.05, respectively. The acceptable uncertainty is less than or equal to 5%. Appendix (A) shows the statistical and error analyses associated with each measurement and Appendix (B) shows the summary of relative uncertainty and accuracy of this experiment. The overall uncertainty of this study was calculated by using eqn (3). |
 | (3) |
where OU = overall uncertainty; UFC = uncertainty of FC; UWSD = uncertainty of WSD.
4. Result and discussion
4.1. Viscosity
Viscosity is an essential property of automotive engine oil, which should be high enough to resist the internal flow and low enough to prevent substantial energy losses.48 Viscosity is high when used oil has been deteriorated by oxidation or by solid contaminants while it is low when used oil is diluted with lower viscosity oil or by fuel. Change in the engine oil viscosity as a result of fuel dilution is undesirable in the lubrication system of an engine as it influences the lubricating efficiency and oil film thickness. Inadequate oil viscosity affects lubricating film and load bearing capacity leading to excessive wear of bearings, journals and other moving components, low oil pressure and poor oil economy.49
Fig. 2 shows the degradation of the viscosity of synthetic oil (SO) diluted with different types of fuel blends, measured at 40 °C. It is investigated that the viscosity decreased significantly when synthetic oil was diluted with bioethanol blends. This is due to the lower viscosity of the diluted fuels. The variations in the viscosity of each diluted oil sample were almost identical. Synthetic oil diluted with E85 fuel had slightly higher viscosity compared to other fuel diluted oil samples. The viscosity of E85–SO was dropped to 62.45 mm2 s−1 from the base oil value of 82.03 mm2 s−1. E85–SO sample also contains a large amount of ethanol, which may cause more interference with the formation of the boundary layer as well as corrosive wear, leading to a thinner boundary film (low lubricating performance) and also to higher friction, compared to fresh oil because the decrease in viscosity may also cause more contact between the asperities of the surface.37 However, during full hydrodynamic lubrication, the reduction in viscosity may contribute to a decrease in friction due to the fact that low viscosity plays a very important role in reducing friction in this full film lubrication regime. The drop in viscosity indicates that the amount of fuel deteriorated the lubricating efficiency of the oil. E0–SO had the viscosity of 58.52 mm2 s−1 which was slightly higher than the viscosities of E10–SO, E20–SO, and E30–SO. This indicates that oil contaminated with gasoline which is the hydrocarbon compound has lower lubricity and undergo more severe degradation compared to the oil contaminated with a small amount of bioethanol. In Table 6, it can be seen that some viscosities of the oil samples could not be determined at 100 °C. This was due to the evaporation of bioethanol at high temperature. It can be stated that fuel dilution has a significant effect on the oil viscosity. It can result in two opposing effects: one beneficial effect and one detrimental effect. A decrease in viscosity is expected to lower the viscous losses of the lubricating oil, which reduce the overall engine friction and increase the efficiency of the engine. However, in boundary lubrication, the difference in viscosity has no significant effect on friction. The reduction in lubricating oil viscosity is also known to reduce engine oil film thickness. As a result, it will push the contact surface more into the boundary lubrication regime.33 This will result in high friction and wear after a long period of four-ball testing.
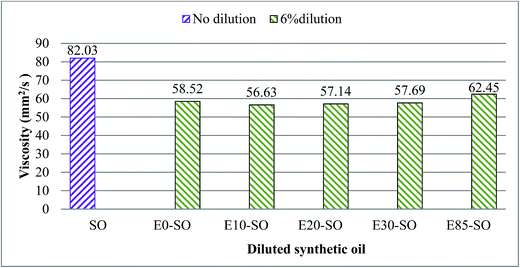 |
| Fig. 2 Variation of viscosity of synthetic oil diluted with bioethanol blends measured at 40 °C. | |
4.2. Acid number
The acid number is the quantity of the higher organic acid or acid-like derivatives in the lubricant and is the indicator of oil serviceability and higher susceptibility of the contact surface to corrosion.50 At higher temperature, fuel molecules and other organic acids inside lubricant are decomposed during operation and hence, increase the acidity. The acid number results are shown in Table 6. Since bioethanol is highly evaporative at high temperature, the change in the acid number of each fuel–oil sample was determined before conducting four-ball wear tests. Generally, the acid value of the new oil is not necessarily nil since oil additives can be acidic in nature. An increase in acid number indicates that lubricant is contaminated with bioethanol and when acid number increase, it makes the metal surface more susceptible to corrosion.4,50 It can be seen that synthetic oil samples diluted with 6% of bioethanol–gasoline fuels show higher acid number, compared to fresh SO. This, in turn, may provoke undesirable corrosive wear on engine components.16,51 Bioethanol is more chemically reactive than gasoline due to the presence of oxygen. This possibly interacts with lubricant base fluid and dissolved additives such as detergent and dispersants containing in the commercial engine oil, thus leading to oil degradation. The degradation of ethanol in the lubricant creates ethanoic (acetic) acid, which attacks soft metals and increases oxidation of the oil.52 As a consequence, it is possible that 6% dilution rate may cause serious effects such as oxidation and corrosion of engine oil, especially for synthetic oil diluted with fuel containing 85% v/v of bioethanol (E85–SO). Lubricant diluted with bioethanol–gasoline mixtures becomes degraded and produces higher wear compared to fresh engine oil.
4.3. Flash temperature parameter (FTP)
As mention in Section 3.6, flash temperature parameter (FTP) is a single number that is used to express the critical flash temperature at which an engine oil will fail under selected conditions. The FTP also refers to the possibility of lubricant breakdown.43 Generally, a greater FTP number indicates a better lubricating efficiency of the lubricant while a smaller FTP number indicates the reduction in lubricating film thickness.41 As seen in eqn (2), load and wear scar diameter (WSD) predict FTP number. Fig. 3 illustrates the variations of the FTP of fresh synthetic oil and diluted synthetic oils tested at both conditions. At the load of 40 kg, it can be seen that fresh synthetic oil (SO) had the highest FTP (178.19) indicating that fresh SO provided better lubricating performance and had less possibility of lubricating film breakdown, while all fuel diluted synthetic oils showed lower FTP numbers. The FTP values of E0–SO, E10–SO, E20–SO, E30–SO, and E85–SO were 155, 155.60, 156.74, 152.76 and 145.29 which meant that their lubricating performance is worse compared non-diluted oil. The least FTP was found in E85–SO which contained a higher amount of bioethanol inside the synthetic oil while E10–SO showed lower FTP value compared to other fuel diluted synthetic oils. The FTP number dropped significantly when the load was increased to 80 kg, which can be explained that at higher load and temperature the degradation of tested lubricating oil may occur and thus push the rubbing surface close to each other, which eventually increase the friction.53 The FTP values of lubricating oils were slightly different. SO, E0–SO, E10–SO, E20–SO, E30–SO, and E85–SO had FTP values of 34, 33.10, 29.38, 31.57, 30.74 and 28.41, respectively. The fresh synthetic oil still had higher FTP compared to the fuel diluted oils which can be explained that it may have better lubricating performance during high load, compared to fuel–oil mixtures. However, fuel contaminant particularly bioethanol inside synthetic oil deteriorated lubricating properties and may cause lubricating film breakdown at high load.
 |
| Fig. 3 Flash temperature parameter of diluted synthetic oil at different loads. | |
4.4. Effect of bioethanol fuels on friction
Fig. 4 describes the variation of FC of synthetic oil (SO) contaminated with bioethanol blended fuels at the load of 40 kg. There were slight differences of FC between synthetic oil and bioethanol diluted synthetic oil samples. It was due to the evaporation of bioethanol at high temperature or their slight differences in viscosity which caused almost identical friction behaviors. The average FC values of fresh oil and fuel-diluted oils tested at 40 kg and 80 kg are shown in Fig. 5. At 40 kg, the friction coefficients yielded by diluted synthetic oils were slightly higher than that yielded by the fresh synthetic oil. Based on the data averaged from the three tests, it is shown that E0–SO, E10–SO, E20–SO, E30–SO, and E85–SO had FC values of 0.111, 0.108, 0.106, 0.109 and 0.112, respectively. It can be seen that the average FC of SO was not the lowest value (0.108) while E20–SO had the lowest FC value (0.106). However, synthetic oil had the lowest frictional behavior as shown in Fig. 4. At first 30 min, SO performed higher friction compared to E10–SO, E20–SO, and E30–SO while its friction decreased to the minimum value during the remaining time. It might be due to the fact that oil additive has been activated to create lubricating film after half an hour. All fuel diluted oils had increasing or stable frictional characteristics, compared to fresh synthetic oil. It is observed that all bioethanol diluted oils yielded lower friction at first 30 min, which was probably because of the presence of oxygen and polarity of bioethanol that could enhance the lubricity of the oils.54 Moreover, there might be a stronger physical adsorption on surfaces of the rotating balls which reduce interference between asperities and increase contact resistance, thus stabilizing the friction behavior in the lubricant wear test.55 However, the synthetic oil contains base fluid and oil additive concentrations which possibly react with bioethanol under the presence of oxygen, causing oil degradation and oxidation, thus increasing friction.4 The more fuel dilutes into the lubricant, the more interactive effects on lubricant additive occurs after a particular operating time; as a result, no lubricating film may generate. E85–SO yielded lower friction compared to SO, but after 15 min it yielded the highest friction value due to the higher amount of bioethanol. It shall be notified that gasoline would result in higher friction compared to intermediate-level bioethanol fuels because gasoline (the mixture of hydrocarbon) has less lubricity than a polar molecule such as bioethanol.54 The variation of FC of diluted synthetic oils tested under the load of 80 kg is shown in Fig. 6. At this high load, it can be seen that all fuel diluted synthetic oils showed higher FC, compared to fresh synthetic oil. The average FC values of E0–SO, E10–SO, E20–SO, E30–SO, and E85–SO were 0.129, 0.113, 0.132, 0.115 and 0.118, respectively while SO had FC value of 0.111 which was slightly lower (Fig. 5). Synthetic oil performed the lowest frictional characteristics and had the minimum value during the whole testing time. As mentioned earlier, when synthetic oil was not diluted by a mixture of gasoline and bioethanol, it has better lubricating efficiency compared to fuel-diluted oils because the oil additive plays a role in reducing friction by creating the lubricating film. It can be seen that E0–SO and E20–SO had significantly higher FC values, respectively due to the increase of load (80 kg). At high load, the thermal vibration of fuel–oil molecules may occur due to additional frictional heating, causing a more decrease in the viscosity of these tested oils mixtures. The combination of lower oil viscosity and high loading, the film thickness is very low, resulting in boundary lubrication.56 Therefore, the high contact stress on surfaces caused plastic deformation of the contact surface, thus reducing the stress in the contact region and increasing the contact area with a higher friction coefficient.55 However, at this 80 kg load, the high bioethanol containing blends such as E85–SO and E30–SO yielded lower frictions than E0–SO and E20–SO samples. During high load test, the frictional heat can be induced, so the test temperature may exceed the boiling point of the bioethanol (78 °C) and cause bioethanol to become more chemically reactive and enhance corrosive reactions. The high bioethanol containing oils also have higher acidity which can cause more corrosion to the contact surface, especially at elevated test temperature. This eventually reduces the friction because the corroded surface is plowed with low friction force, whereas the amount of wear increases due to corrosive wear resulting from the high amount of bioethanol.57 Overall, it can be stated that a small amount of ethanol in lubricant may help increase the lubricity of the lubricant temporarily, but increase friction when it interacts with lubricant and oil additives. There might be also oxidation between oxygenated component (bioethanol) and lubricant (SO) additives, which can destroy the oil lubricating performance.
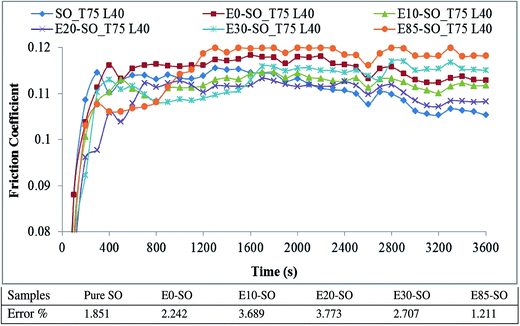 |
| Fig. 4 Friction coefficient of synthetic oil contaminated with different blended fuels at 6% dilution at 75 °C and L40 kg. | |
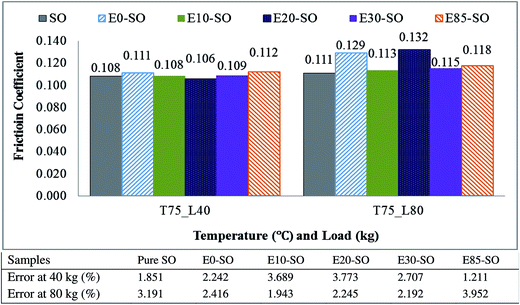 |
| Fig. 5 Average friction coefficient of each fuel diluted synthetic oil sample. | |
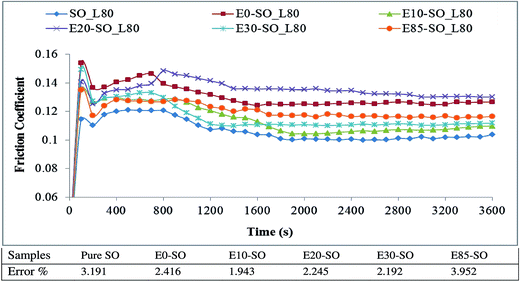 |
| Fig. 6 Friction coefficient of synthetic oil contaminated with different blended fuels at 6% dilution at 75 °C and load 80 kg. | |
4.5. Effect of bioethanol fuels on wear behavior
Fig. 7 illustrates the variation of wear scar diameter (WSD) of the ball tested with synthetic oil diluted with various gasoline–bioethanol fuels at 40 kg and 80 kg. Although gasoline–bioethanol fuel dilution has a little effect on frictional behavior, it is observed that the WSD seems to increase significantly with an increase in bioethanol amount inside engine oil. The WSD of the three stationary balls were measured and averaged for graph plotting. Generally, when the scar is large, it indicates a severe deterioration of material on the contact surfaces. At 40 kg load, it can be seen that all fuel diluted synthetic oils had higher wear scar diameter, compared to their fresh oil (SO). The WSD of E0–SO, E10–SO, E20–SO, E30–SO, and E85–SO were 0.380 mm, 0.379 mm, 0.377 mm, 0.384 mm and 0.398 mm, respectively while synthetic oil yielded WSD of 0.344 mm. The increase in the values of WSD for fuel diluted oils is due to the fact that fuel dilution reduces wear performance of the engine oil. The amount of bioethanol fuel dilution degrades the engine oil and reduce the performance of the oil, especially in the case when SO is contaminated with higher percentages of gasoline–bioethanol mixtures. The addition of bioethanol is known to reduce the film thickness of the lubricating oil.37 Fresh synthetic oil which was not contaminated with fuel mixture had a better lubricating performance due to its oil additive concentration which can generate the lubricating film. However, in the case of fuel dilution of engine oil, it affects lubricating properties (weakening the lubricant detergency, forming acid and corrosion, and reducing of the oil film causing metal asperities to contact each other promoting engine wear), thus increasing in friction and wear losses. Moreover, fuel dilution reduces oil viscosity, and the concentration of engine oil additives, potentially compromising lubricant's performance.58 Inadequate oil viscosity affects oil film formation and load-bearing capacity. This can potentially lead to excessive wear of bearing, journals and other moving components.58 The effect of load on wear scar is very significant. The large amount of WSD could cause more wear. At 80 kg load, the contact area is very big; as a result, the WSD of each test lubricant is extremely large, compared to the ball tested at 40 kg. All fuel diluted oil samples namely E0–SO, E10–SO, E20–SO, E30–SO and E85–SO had WSD of 1.879 mm, 2.045 mm, 1.943 mm, 1.980 mm and 2.095 mm respectively while the WSD of SO was 1.844 mm. Upon increasing of operation time, the oil additive will be depleted gradually and bioethanol may react with base oil and lubricant additive under the presence of oxygen, and causes oil oxidation of some of the lubricant constituents and subsequent formation of carboxylic acid and eventually destroys lubricating performance.59 E0–SO, E20–SO, E30–SO, and E85–SO had higher WSD, respectively with an increase in the amount of ethanol. These fuel-diluted oil samples also caused additional corrosive wear due to their higher acidity, compared to fresh synthetic oil. These samples are more chemically reactive and yield corrosive wear when there is elevated test temperature from frictional heat at this higher load (80 kg).57 E10–SO had higher WSD compared to other oils except this E85–SO while still remaining low friction. The increase in WSD of this oil was probably due to the interaction between asperities and contact surface which increase the contact area, thus increasing the amount of wear. It might be also due to the chemical attack on the surface of the ball by bioethanol presented in this oil.55 It shall be notified that the less effect on WSD is due to a large evaporated amount of bioethanol from the lubricant. It is believed that the WSD will be more severe when 6% of bioethanol fuel diluted into the oil sump in the vehicle engine where fuel is always diluted during engine operation, suggesting that ethanol-resistant engine oil should be produced in order to avoid serious wear from bioethanol, which can lead to engine catastrophic failure.
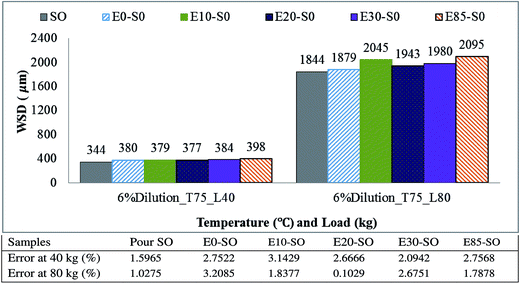 |
| Fig. 7 Wear scar diameter of synthetic oil diluted with 6% gasoline–bioethanol blends. | |
4.6. SEM analysis of worn surfaces
Fig. 8 shows the worn surface morphologies of stationary balls tested in SO, E0–SO, E10–SO, E20–SO, E30–SO and E85–SO at 40 kg. For fresh synthetic oil, the ball surface indicated mild abrasive wear. The micro grooves along the sliding direction were presented on the worn surface (Fig. 8a). It shall be notified that the adhesive wear is indicated on the worn surface when it is greater than 20 μm.60 In Fig. 8b–f, more severe surface deteriorations were found on the balls tested with lubricant diluted with gasoline–bioethanol mixtures. The balls tested with gasoline oil (E0–SO) showed moderate wear mechanism including abrasive wear as shown in Fig. 8b. The grooves along the surface were slightly bigger, compared to the fresh SO. The ball surface of bioethanol oils looked smoother, compared to that of gasoline oil because bioethanol is a polar molecule which has a better lubricity compared to gasoline (the mixture of hydrocarbon). However, the worn surface of the balls tested with bioethanol-diluted synthetic oils indicated more surface deterioration from abrasive wear and chemical wears (Fig. 8c–f). E10–SO shows fracture and delamination on the surface. E20–SO and E30–SO indicate light abrasive wear as small grooves and pits can be observed on the surfaces. The surface of E85–SO shows smoother surface and almost no groove indicated. This may be due to higher acid in this oil which corrodes the surface and the corroded surface is eventually plowed away by its counterpart. These fuel-diluted oils seem to undergo chemical reaction between lubricant (base fluid and oil additives), and bioethanol which may cause chemical wears such as oxidative and corrosive wear, thus increasing wear losses. Oxidative wear occurs due to the higher oxygen content of bioethanol inside the oil, which enhances the oxidation of fuel–oil mixture (Fig. 8c–f). This wear caused material removal by oxidative chemical reaction of the metal surface. Corrosive wear is the gradual deterioration of unprotected metal surfaces, caused by atmosphere and acid, and then creates pits and small cracks. This corrosive wear may also dissolve metal parts and cause plowing on the surfaces. It can also be seen that there were presences of the corrosive product of black colors due to the fact that oxidization and corrosion occurred and corrosive acids enhanced corrosive wear.61,62 Moreover, during the test at elevated temperature, the oxidation of fuel–oil mixture may generate peroxide in the present of oxygen, and this peroxide will undergo further reaction to form carboxylic acid, ketones, aldehydes, and alcohols; as a result, it may increase the acidity of the lubricant63,64 (Fig. 8d–f). From this SEM, it can be deduced that bioethanol blend inside synthetic oil resulting from fuel dilution is possible to degrade lubricating performance and also affect the friction and wear behaviors of lubricant.
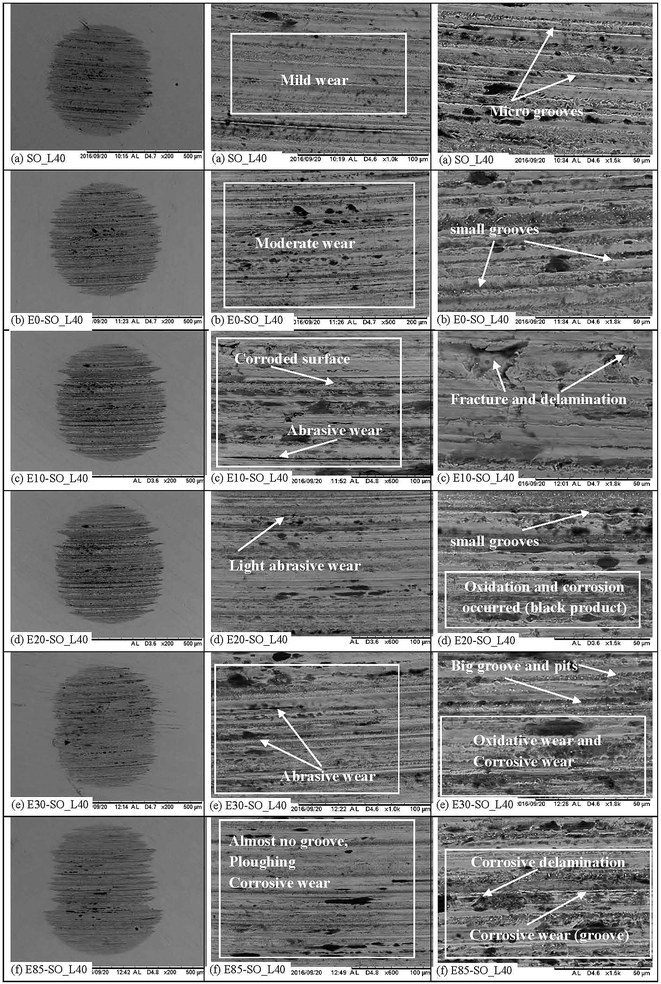 |
| Fig. 8 Worn surface of steel balls tested with fresh and diluted synthetic oils (test condition: load = 40 kg; speed = 1200 rpm; duration = 1 h; temperature = 75 °C). | |
5. Conclusion
This experimental study was conducted to assess the impact of bioethanol–gasoline blends, on the tribological performance of engine oil under selected conditions using four-ball wear tester. The results of the degradation of properties, friction, and wear losses of the contaminated engine oil have been thoroughly discussed. The conclusions can be drawn as the followings:
• The 6% addition of all bioethanol–gasoline blends to fully-synthetic oil significantly decreased the viscosity of the fresh oil to about 30%, compared to fresh synthetic oil. The decrease in viscosity from ethanol dilution may result in the thinner boundary film and higher wear because viscosity reduction causes more contact between asperities of the surface.
• Bioethanol–gasoline diluted oils show slightly higher the acid number, compared to fresh synthetic oil. The engine oil needs to be more alkaline to prevent the metal surface from corrosion. However, bioethanol is more chemically reactive compared to gasoline, which enhances the degradation of the fuel–oil mixture. Therefore, the fuel diluted lubricants contain more acidity making the surface more susceptible to corrosion, thus increasing wear losses.
• The addition of ethanol–gasoline fuels to synthetic oil shows that there is no clear trend or conclusive indication of each fuel–oil mixture is worse for friction at both loads, but during the test, it was shown that the ethanol rapidly and fully evaporated from the lubricant at the temperature of 75 °C. This minimal effect on the friction behavior is also due to the fact that the tests were conducted under boundary lubrication regime.
• Although bioethanol fuels have slight impact on the frictional characteristic of the oil, it has significant differences in the amount of wear. At both 40 kg and 80 kg loads, fuel–oil mixtures increase wear losses more or less with the increased amount of bioethanol, compared to their fresh oil. E30–SO and E85–SO have higher wear losses but low friction because acid corrodes the surface and causes additional corrosive wear. From the SEM of the worn surface of tested ball, it can be seen that the worn surface of the fuel–oil samples has more surface deterioration than fresh oil. Additional damage and chemical wears (oxidative wear and corrosive wear) can be investigated on the surface of the ball tested with these oils.
Overall, it can be concluded that 6% of bioethanol fuel dilution can degrade properties performance (viscosity and alkalinity) and tribological behaviors of the synthetic oil. Ethanol fuel dilution may reduce friction, compared to pure gasoline due to its polarity and acidity. However, high acid in the oil causes high wear due to its corrosion on the surface, suggesting that ethanol-resistant engine oil should be produced in order to avoid serious wear from bioethanol.
Appendix A and B
Tables 7–13
Table 7 Uncertainty analysis for FTP at 40 kg
Samples |
FTP 1 |
FTP 2 |
FTP 3 |
Min. |
Max. |
Accuracy |
Avg. |
Uncertainty% |
0.01 |
−0.01 |
+ |
− |
Pure SO |
180.39 |
181.88 |
173.93 |
173.93 |
181.88 |
181.888 |
173.916 |
177.902 |
2.234 |
−2.234 |
E0–SO |
156.74 |
160.30 |
148.41 |
148.41 |
160.30 |
160.311 |
148.398 |
154.354 |
3.852 |
−3.852 |
E10–SO |
162.75 |
155.01 |
148.94 |
148.94 |
162.75 |
162.762 |
148.930 |
155.846 |
4.431 |
−4.431 |
E20–SO |
155.01 |
164.00 |
152.20 |
152.20 |
155.01 |
155.021 |
152.190 |
153.605 |
0.915 |
−0.915 |
E30–SO |
149.48 |
162.75 |
151.65 |
149.48 |
162.75 |
162.762 |
149.465 |
156.113 |
4.252 |
−4.252 |
E85–SO |
148.41 |
139.37 |
145.29 |
139.37 |
148.41 |
148.418 |
139.357 |
143.888 |
3.141 |
−3.141 |
Uncertainty level |
|
|
|
|
|
|
|
|
3.137 |
−3.137 |
Table 8 Uncertainty analysis for FTP at 80 kg
Samples |
FTP 1 |
FTP 2 |
FTP 3 |
Min. |
Max. |
Accuracy |
Avg. |
Uncertainty% |
0.01 |
−0.01 |
+ |
− |
Pure SO |
33.36 |
34.33 |
34.22 |
33.36 |
34.33 |
34.338 |
33.345 |
33.841 |
1.438 |
−1.438 |
E0–SO |
31.86 |
34.86 |
32.62 |
31.86 |
34.86 |
34.871 |
31.85 |
33.362 |
4.490 |
−4.490 |
E10–SO |
29.22 |
28.73 |
30.25 |
28.73 |
30.25 |
30.260 |
28.72 |
29.492 |
2.572 |
−2.572 |
E20–SO |
31.54 |
31.63 |
31.54 |
31.54 |
31.63 |
31.644 |
31.53 |
31.589 |
0.144 |
−0.144 |
E30–SO |
30.00 |
30.00 |
32.33 |
30.00 |
32.33 |
32.342 |
29.99 |
31.165 |
3.744 |
−3.744 |
E85–SO |
28.50 |
27.67 |
29.09 |
27.67 |
29.09 |
29.095 |
27.66 |
28.375 |
2.502 |
−2.502 |
Uncertainty level |
|
|
|
|
|
|
|
|
2.482 |
−2.482 |
Table 9 Uncertainty analysis for FC at 40 kg
Samples |
FC 1 |
FC 2 |
FC 3 |
Min. |
Max. |
Accuracy |
Avg. |
Uncertainty% |
0.01 |
−0.01 |
+ |
− |
Pure SO |
0.109 |
0.110 |
0.115 |
0.109 |
0.115 |
0.125 |
0.0994 |
0.1122 |
2.49919 |
−2.49919 |
E0–SO |
0.114 |
0.114 |
0.109 |
0.109 |
0.114 |
0.124 |
0.0990 |
0.1115 |
2.24215 |
−2.24215 |
E10–SO |
0.103 |
0.111 |
0.103 |
0.103 |
0.111 |
0.1208 |
0.0931 |
0.1070 |
3.5998 |
−3.5998 |
E20–SO |
0.112 |
0.109 |
0.109 |
0.109 |
0.112 |
0.122 |
0.0987 |
0.1104 |
1.49524 |
−1.49524 |
E30–SO |
0.106 |
0.112 |
0.103 |
0.103 |
0.112 |
0.1219 |
0.0927 |
0.1073 |
4.28704 |
−4.28704 |
E85–SO |
0.110 |
0.113 |
0.1125 |
0.110 |
0.113 |
0.1228 |
0.1001 |
0.1115 |
1.21130 |
−1.21130 |
Uncertainty level |
|
|
|
|
|
|
|
|
2.55579 |
−2.55579 |
Table 10 Uncertainty analysis for FC at 80 kg
Samples |
FC 1 |
FC 2 |
FC 3 |
Min. |
Max. |
Accuracy |
Avg. |
Uncertainty% |
0.01 |
−0.01 |
+ |
− |
Pure SO |
0.1093 |
0.1148 |
0.1077 |
0.1077 |
0.1148 |
0.1248 |
0.0977 |
0.1112 |
3.19101 |
−3.19101 |
E0–SO |
0.1314 |
0.1252 |
0.1292 |
0.1252 |
0.1314 |
0.1414 |
0.1152 |
0.1283 |
2.41621 |
−2.41621 |
E10–SO |
0.1110 |
0.1154 |
0.1125 |
0.1110 |
0.1154 |
0.1254 |
0.1010 |
0.1132 |
1.94346 |
−1.94346 |
E20–SO |
0.1323 |
0.1284 |
0.1343 |
0.1284 |
0.1343 |
0.1443 |
0.1184 |
0.1313 |
2.24590 |
−2.24590 |
E30–SO |
0.1115 |
0.1165 |
0.1157 |
0.1115 |
0.1165 |
0.1265 |
0.1015 |
0.1140 |
2.19298 |
−2.19298 |
E85–SO |
0.1176 |
0.1223 |
0.1130 |
0.1130 |
0.1223 |
0.1323 |
0.1030 |
0.1176 |
3.95240 |
−3.95240 |
Uncertainty level |
|
|
|
|
|
|
|
|
2.65699 |
−2.65699 |
Table 11 Uncertainty analysis for WSD at 40 kg
Samples |
WSD 1 |
WSD 2 |
WSD 3 |
Min. |
Max. |
Accuracy |
Avg. |
Uncertainty% |
0.01 |
−0.01 |
+ |
− |
Pure SO |
341 |
339 |
350 |
339 |
350 |
350.01 |
338.99 |
344.5 |
1.59651 |
−1.59651 |
E0–SO |
377 |
371 |
392 |
371 |
392 |
392.01 |
370.99 |
381.5 |
2.75229 |
−2.75229 |
E10–SO |
367 |
380 |
391 |
367 |
391 |
390.53 |
366.71 |
378.62 |
3.14299 |
−3.14299 |
E20–SO |
380 |
365 |
385 |
365 |
385 |
385.01 |
364.99 |
375 |
2.66666 |
−2.66666 |
E30–SO |
390 |
3674 |
386 |
374 |
390 |
390.01 |
373.99 |
382 |
2.09424 |
−2.09424 |
E85–SO |
388 |
410 |
398 |
388 |
410 |
410.01 |
387.99 |
399 |
2.75689 |
−2.75689 |
Uncertainty level |
|
|
|
|
|
|
|
|
2.50160 |
−2.50160 |
Table 12 Uncertainty analysis for WSD at 80 kg
Samples |
WSD 1 |
WSD 2 |
WSD 3 |
Min. |
Max. |
Accuracy |
Avg. |
Uncertainty% |
0.01 |
−0.01 |
+ |
− |
Pure SO |
1868 |
1830 |
1834 |
1830 |
1868 |
1868.01 |
1829.99 |
1849 |
1.02758 |
−1.02758 |
E0–SO |
1930 |
1810 |
1898 |
1810 |
1930 |
1930.01 |
1809.99 |
1870 |
3.20855 |
−3.20855 |
E10–SO |
2053 |
2078 |
2003 |
2003 |
2078 |
2078.01 |
2002.99 |
2040.5 |
1.83778 |
−1.83778 |
E20–SO |
1944 |
1940 |
1944 |
1940 |
1944 |
1944.01 |
1939.99 |
1942 |
0.10298 |
−0.10298 |
E30–SO |
2015 |
2015 |
1910 |
1910 |
2015 |
2015.01 |
1909.99 |
1962.5 |
2.67515 |
−2.67515 |
E85–SO |
2090 |
2135 |
2060 |
2060 |
2135 |
2135.01 |
2059.99 |
2097.5 |
1.78784 |
−1.78784 |
Uncertainty level |
|
|
|
|
|
|
|
|
1.773318 |
−1.77331 |
Table 13 The summary of relative uncertainty and accuracy of this experiment
Parameters |
Accuracy |
Uncertainty |
Friction coefficient (FC) |
±0.05 |
At load 40 kg: ±2.501 |
At load 80 kg: ±1.773 |
Wear scar diameter (WSD) |
±0.01 |
At load 40 kg: ±2.556 |
At load 80 kg: ±2.6569 |
Acknowledgements
The first author is greatly indebted to the Japan International Cooperation Agency (JICA) and AUN/Seed-Net for funding the author's postgraduate study as well as providing the research grant under the ‘Collaborative Research’ programme. The authors amiably thank the Ministry of Higher Education Malaysia and University of Malaya, Malaysia for funding this study through the Grand Challenge Project (GC001-14AET), UMRG Grant (RP016-2012B), and FRGS Grant (FP063-2015A). Authors also appreciate International Scientific Partnership Program (Ref no. ISPP#0092) at Kind Saud University (KSU), Saudi Arabia for funding this research work.
References
- S. Shafiee and E. Topal, Energy Policy, 2009, 37, 181–189 CrossRef.
- E. De Cian, F. Sferra and M. Tavoni, Clim. Change, 2016, 136, 39–55 CrossRef.
- E. Martinot and J. Sawin, Renewables 2005: Global status report, Worldwatch Institute, Washington, DC, 2005 Search PubMed.
- L. Khuong, N. Zulkifli, H. Masjuki, E. N. Mohamad, A. Arslan, M. Mosarof and A. Azham, RSC Adv., 2016, 6, 66847–66869 RSC.
- T. Wallner and S. A. Miers, Combustion behavior of gasoline and gasoline/ethanol blends in a modern direct-injection 4-cylinder engine, 2008, Report 0148-7191, SAE Technical Paper Search PubMed.
- D. D. Dutcher, M. R. Stolzenburg, S. L. Thompson, J. M. Medrano, D. S. Gross, D. B. Kittelson and P. H. McMurry, Atmosphere, 2011, 2, 182–200 CrossRef CAS.
- M. Cavalcanti, A. Szklo and G. Machado, Renewable Energy, 2012, 43, 423–433 CrossRef.
- R. Linde and J. Frode, Emission and experience with E85 converted cars in the BEST project, Sweden, Vaxjo, 2010 Search PubMed.
- L. Pelkmans, G. Lenaers, J. Bruyninx, K. Scheepers and I. De Vlieger, Proc. Inst. Mech. Eng., Part D, 2011, 225, 1204–1220 CrossRef CAS.
- R. Magnusson, C. Nilsson and B. Andersson, Environ. Sci. Technol., 2002, 36, 1656–1664 CrossRef CAS PubMed.
- G. Chui and R. Baker, Lubrication behavior in ethanol-fueled engines, 1980 Search PubMed.
- M. Boons, R. Van den Bulk and T. King, The impact of E85 use on lubricant performance, 2008, Report 0148-7191, SAE Technical Paper Search PubMed.
- T. Tsunooka, Y. Hosokawa, S. Utsumi, T. Kawai and Y. Sonoda, High concentration ethanol effect on SI engine cold startability, 2007, Report 0148-7191, SAE Technical Paper Search PubMed.
- S. Shanta, G. Molina and V. Soloiu, Adv. Tribol., 2011 DOI:10.1155/2011/820795.
- P. De Silva, M. Priest, P. Lee, R. Coy and R. Taylor, Tribol. Lett., 2011, 43, 107–120 CrossRef.
- C. Besser, C. Schneidhofer, N. Dörr, F. Novotny-Farkas and G. Allmaier, Tribol. Int., 2012, 46, 174–182 CrossRef CAS.
- H. L. Costa and H. A. Spikes, Tribol. Int., 2016, 93, 364–376 CrossRef CAS.
- R. A. Mufti and M. Priest, J. Tribol., 2009, 131, 041101 CrossRef.
- M. Quazi, M. Fazal, A. Haseeb, F. Yusof, H. Masjuki and A. Arslan, Lasers in Manufacturing and Materials Processing, 2016, 3, 67–99 CrossRef.
- M. Quazi, M. Fazal, A. Haseeb, F. Yusof, H. Masjuki and A. Arslan, Crit. Rev. Solid State Mater. Sci., 2016, 41, 106–131 CrossRef CAS.
- A. Arslan, H. H. Masjuki, M. Varman, M. A. Kalam, M. M. Quazi and M. H. Mosarof, J. Mater. Res., 2016, 1–11 Search PubMed.
- A. Arslan, H. Masjuki, M. Kalam, M. Varman, R. Mufti, M. Mosarof, L. Khuong and M. Quazi, Crit. Rev. Solid State Mater. Sci., 2016, 1–35 Search PubMed.
- A. Arslan, H. Masjuki, M. Varman, M. Kalam, M. Quazi, K. Al Mahmud, M. Gulzar and M. Habibullah, Appl. Surf. Sci., 2015, 356, 1135–1149 CrossRef CAS.
- M. Mosarof, M. A. Kalam, H. Masjuki, A. Arslan, M. M. Islam, A. M. Ruhul, S. S. Ali and S. K. Leang, RSC Adv., 2016, 6, 81414–81425 RSC.
- P. Nagendramma and S. Kaul, Renewable Sustainable Energy Rev., 2012, 16, 764–774 CrossRef CAS.
- R. M. Mortier, S. T. Orszulik and M. F. Fox, in Chemistry and technology of lubricants, Springer, 2010, vol. 107115, ch. 2 Search PubMed.
- A. Jackson, Synthetic versus mineral fluids in lubrication, International Tribology Conference 1987, Melbourne, 2–4 December 1987, Preprints of Papers, Institution of Engineers, Australia, 1987.
- M. Lapuerta, O. Armas and J. M. Herreros, Fuel, 2008, 87, 25–31 CrossRef CAS.
- M. Wattrus, SAE Int. J. Fuels Lubr., 2013, 6, 794–806 CrossRef CAS.
- G. Chui and D. Millard, Development and testing of crankcase lubricants for alcohol fueled engines, 1981, Report 0148-7191, SAE Technical Paper Search PubMed.
- T. Hu, H. Teng, X. Luo and B. Chen, SAE International Journal of Engines, 2015, 8, 1107–1116 Search PubMed.
- N. Tippayawong and P. Sooksarn, Maejo Int. J. Sci. Technol., 2010, 4, 201–209 CAS.
- O. O. Ajayi, C. Lorenzo-Martin, G. Fenske, J. Corlett, C. Murphy and S. Przesmitzki, J. Tribol., 2016, 138, 021603 CrossRef.
- T. Cousseau, J. S. R. Acero and A. Sinatora, Tribol. Int., 2015, 100, 60–69 CrossRef.
- M. Abu-Qudais, O. Haddad and M. Qudaisat, Energy Convers. Manage., 2000, 41, 389–399 CrossRef CAS.
- P. De Silva, M. Priest, P. Lee, R. Coy and R. Taylor, Proc. Inst. Mech. Eng., Part J, 2011, 225, 347–358 CrossRef CAS.
- H. L. Costa and H. Spikes, Tribol. Trans., 2015, 58, 158–168 CrossRef CAS.
- V. W. Wong and S. C. Tung, Friction, 2016, 4, 1–28 CrossRef CAS.
- K. Al Mahmud, M. Varman, M. Kalam, H. Masjuki, H. Mobarak and N. Zulkifli, Surf. Coat. Technol., 2014, 245, 133–147 CrossRef CAS.
- M. Söderfjäll, A. Almqvist and R. Larsson, Tribol. Int., 2016, 104, 57–63 CrossRef.
- M. H. Mosarof, M. A. Kalam, H. H. Masjuki, A. Alabdulkarem, M. Habibullah, A. Arslan and I. M. Monirul, Ind. Crops Prod., 2016, 83, 470–483 CrossRef CAS.
- R. Rastogi, M. Yadav and A. Bhattacharya, Wear, 2002, 252, 686–692 CrossRef CAS.
- H. Masjuki and M. Maleque, Wear, 1997, 206, 179–186 CrossRef CAS.
- H. Masjuki, M. Saifullah, M. Husnawan, M. Faizul and M. Shaaban, in World Tribology Congress III, ASME Proceedings, 2005, vol. 1, pp. 451–452 Search PubMed.
- T. C. Ing, A. Mohammed Rafiq, Y. Azli and S. Syahrullail, Tribol. Trans., 2012, 55, 539–548 CrossRef CAS.
- H. H. Masjuki and M. A. Maleque, Wear, 1997, 206, 179–186 CrossRef CAS.
- M. Mofijur, H. Masjuki, M. Kalam, A. Atabani, I. R. Fattah and H. Mobarak, Ind. Crops Prod., 2014, 53, 78–84 CrossRef CAS.
- D. Ljubas, H. Krpan and I. Matanović, Nafta, 2010, 61, 73–79 CAS.
- A. K. Agarwal and J. G. Gupta, in Novel Combustion Concepts for Sustainable Energy Development, Springer, 2014, pp. 75–87 Search PubMed.
- S. Arumugam and G. Sriram, Aust. J. Mech. Eng., 2012, 10, 119–128 Search PubMed.
- J. Jakóbiec and A. Mazanek, Teka Komisji Motoryzacyjnej i Energetyki Rolnictwa, 2009, 9, 99–113 Search PubMed.
- K. Howard, Towards Zero Carbon Transportation, in Alternative Fuels and Advanced Vehicle Technologies for Improved Environmental Performance, ed. R. Folkson, Elsevier, UK, 2014, ch. 6, DOI:10.1533/9780857097422.1.138.
- M. Shahabuddin, H. Masjuki, M. Kalam, M. Bhuiya and H. Mehat, Ind. Crops Prod., 2013, 47, 323–330 CrossRef CAS.
- J. Agudelo, Á. Delgado and P. Benjumea, Rev. Fac. Ing., Univ. Antioquia, 2011, 9–16 CAS.
- Y.-C. Lin, T.-H. Kan, J.-N. Chen, J.-C. Tsai, Y.-Y. Ku and K.-W. Lin, Tribol. Trans., 2013, 56, 997–1010 CrossRef CAS.
- V. W. Wong, in Encyclopedia of automotive engineering, ed. D. Crolla, John Wiley & Sons, 2015, ch. 22 Search PubMed.
- Tribology Series, ed. G. W. Stachowiak and A. W. Batchelor, Elsevier, 1993, vol. 24, pp. 637–656 Search PubMed.
- A. K. Agarwal, A. Pandey, A. K. Gupta, S. K. Aggarwal and A. Kushari, Novel combustion concepts for sustainable energy development, Springer, 2014 Search PubMed.
- B. Rahimi, A. Semnani, A. Nezamzadeh-Ejhieh, H. Shakoori Langeroodi and M. Hakim Davood, J. Anal. Methods Chem., 2012, 2012, 819524, DOI:10.1155/2012/819524.
- X. Lu, J. Cotter and D. Eadie, Wear, 2005, 259, 1262–1269 CrossRef CAS.
- B. Briscoe, P. Thomas and D. Williams, Wear, 1992, 153, 263–275 CrossRef CAS.
- N. W. M. Zulkifli, M. A. Kalam, H. H. Masjuki, M. Shahabuddin and R. Yunus, Energy, 2013, 54, 167–173 CrossRef CAS.
- Y. Wu, W. Li, M. Zhang and X. Wang, Tribol. Int., 2013, 64, 16–23 CrossRef CAS.
- S. Q. A. Rizvi, in Lubricant additives: chemistry and applications, ed. L. R. Rudnick, CRC press, 2009, ch. 5 Search PubMed.
|
This journal is © The Royal Society of Chemistry 2017 |