DOI:
10.1039/C7RA00277G
(Paper)
RSC Adv., 2017,
7, 23714-23726
Four-stage biofilm anaerobic–anoxic–oxic–oxic system for strengthening the biological treatment of coking wastewater: COD removal behaviors and biokinetic modeling
Received
10th January 2017
, Accepted 17th April 2017
First published on 3rd May 2017
Abstract
High-strength coking wastewater with a high chemical oxygen demand (COD) was efficiently treated by a novel pilot-scale four-stage biofilm anaerobic–anoxic–oxic–oxic (FB-A2/O2) system. The results demonstrated that the system played an important role in obtaining an overall COD removal efficiency at a hydraulic detention time (HRT) of 116 h and over 60% of COD was removed in A2 and O1 reactors. Three different mathematical models, including the Grau second-order model, modified Stover–Kincannon model, and the Monod-biological contact oxidation model (Monod-BCO model) were applicable to describe the COD removal efficiency of the FB-A2/O2 system. Based on a kinetics study and model evaluation, the Monod-BCO model was demonstrated to be more applicable to COD removal from the system with an average determination coefficient of 0.9661. According to the Monod-BCO model, the COD maximum utilization rates for a unit area of carrier for the A1, A2, O1, and O2 units were 0.1685 g (m2 d)−1, 0.8384 g (m2 d)−1, 0.4654 g (m2 d)−1, and 0.2689 g (m2 d)−1, respectively. The refractory organics in each reactor were further evaluated by the Monod-BCO model. The results proved that the system had great potential for treating high-COD coking wastewater. Step-feeding after the A1 reactor was strongly suggested to further optimize the feeding pattern of the system, depending on the model evaluation.
1. Introduction
Coking wastewater generated from coking, coal gas purification, liquefaction, and the refining of coal by-products at coke plants1 usually contains a large amount of complicated organic compositions, thus endangering natural water bodies and threatening ecological environment and even impacting human health.2 For biodegradable organic contaminants, the conventional activated sludge (CAS) process is considered to be an environmental friendly and low-cost option.3,4 However, compositions of coking wastewater are ordinarily complicated and can involve many diverse bio-inhibitory organic compounds, such as long-chain alkanes, nitrogen heterocyclics (NHCs), and polycyclic aromatic hydrocarbons (PAHs),1,5,6 which are major obstacles for removing the oxygen-consuming substances responsible for the chemical oxygen demand (COD) by the CAS process. Moreover, toxic inhibition from these bio-refractory organic substances frequently leads to poor bioactivity, the difficulty of sludge settling, and weak resistance to loading shocks of the CAS system.7–10 Therefore, the effluent COD of coking wastewater can easily fail to meet the Emission Standard of Pollutants for the Coking Chemical Industry (GB 16171-2012).11
The above problems can be efficiently solved by biofilm systems since attached bio-reactors provide a favorable living environment by offering a higher volume loading rate, longer cell retention time, and more biomass than suspended sludge.12–15 Recently, a lot of attached-growth bio-reactors have been identified as desirable and steady systems suitable for treating refractory organic coking wastewater.12,16–21 However, anaerobic and anoxic stages are indispensable for degrading organic matters combined with aerobic degradation. Therefore, we successfully developed a novel pilot-scale four-stage anaerobic–anoxic–oxic–oxic (FB-A2/O2) system for treating coking wastewater based on the principle of the multi-stage mixed degradation of organics.22 Unlike other biological treatments, the total system consists of four separate biofilm compartments, which are arranged in sequence to favorably treat coking wastewater due to the combination of pre-anaerobic, anoxic and two aerobic processes for organic mineralization. The final effluent COD was improved to a value of less than 100 mg L−1, thus meeting the strict national coking wastewater discharge standards of China.22
Kinetic modeling is considered to be a feasible and convenient approach to deeply understand the removal performance, predict effluent concentration, and to optimize the biological process.23,24 Various models have been reported in previous studies for evaluating the biodegradation kinetics in different kinds of treatments of various wastewaters. The Grau second-order substrate removal model involving only the influents, effluent concentration, and the hydraulic detention time (HRT) was applied to evaluate COD removal from poultry slaughterhouse wastewater25 and cassava starch wastewater.26 The Monod model was used to describe the microbial growth at first.27 Afterward, it could be described well for assessing the kinetic characteristics of pollutant biodegradation.28–32 The Monod-biological contact oxidation model (Monod-BCO model) was modified by the Monod model.33 Besides describing the biodegradation kinetics, the total area of carriers and the concentration of refractory organics were also introduced as variables in the model. The Stover–Kincannon model originates from describing biomass in a rotating biological contactor34 and according to previous reports it is capable of predicting substrate removal for different wastewaters treated by a biofilm reactor.35–41 Different from the Monod model, the modified Stover–Kincannon model introduces the COD loading rate (QSi/V) to the model and simplifies it by not requiring other hard-to-measure parameters. Until now, however, a dynamics model for COD removal of coking wastewater in the aspect of microbial kinetics was still missing.23,42 Apart from this, the treatability and biodegradation potential of COD removal in each unit of the multi-stage process needs to be more deeply understood.
In the present study, the effects of the organic loading rate (OLR) OLR on overall COD removal and its removal proportion in each reactor of the total system were investigated. Also, in contrast to other studies analyzing the kinetics of coking wastewater using a single model,23,42 three different mathematical models, including the Grau second-order model, the modified Stover–Kincannon model, and the Monod-biological contact oxidation model (Monod-BCO model), were applied to establish a more simplified and practical mathematical model for assessing the kinetics of COD removal in our four-stage FB-A2/O2 system for treating coking wastewater. Finally, optimizing suggestions for process improvement were further put forward based on biokinetic analysis and model comparisons.
2. Materials and methods
2.1 COD removal in each reactor
The schematic diagram of the pilot-scale A2/O2 biofilm system was presented in our previous study.22 The characteristics of raw coking wastewater were shown in Table 1. The configuration of the A2/O2 system used for COD mass balance is also presented in Fig. 1. The amount of COD removal in each unit was calculated in accordance with eqn (1)–(4): |
COD removal in A1 reactor = Q(CA1 − Cin)
| (1) |
|
COD removal in A2 reactor = Q[(CA1 − CA2) + R(CO2 − CA2)]
| (2) |
|
COD removal in O1 reactor = Q(1 + R)(CO1 − CA2)
| (3) |
|
COD removal in O2 reactor = Q(1 + R)(CO2 − CO1)
| (4) |
Table 1 Wastewater characterization of raw coking wastewater
Parameters |
Values |
pH |
7.5–8.5 |
COD (mg L−1) |
900–1800 |
BOD5 (mg L−1) |
200–370 |
Ammonium nitrogen (mg L−1) |
150–330 |
BOD5/COD |
0.20–0.28 |
 |
| Fig. 1 Schematic diagram of the pilot-scale A2/O2 biofilm system (A1: anaerobic reactor; A2: anoxic reactor; O1: first oxic reactor; O2: second oxic reactor; Q: flow rate of the influent; R: nitrifying recirculation ratio). | |
2.2 Substrate removal kinetics
2.2.1 Grau second-order substrate removal model. The second-order model could be expressed via eqn (5):43 |
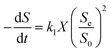 | (5) |
Eqn (6) was obtained as follows by integrating and linearizing from eqn (5):
|
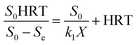 | (6) |
where
k1 is a constant of the second-order model (d
−1) and
X means the biomass concentration in the reactor (g L
−1). When the term

is expressed as a constant
a, the following equations of
eqn (7) and
(8) can be derived:
|
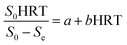 | (7) |
|
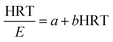 | (8) |
where the values of the constant
b and
a =
S0/(
k1X)
44 are obtained from plotting HRT/
E against HRT, while
E acts as the ammonium nitrogen removal efficiency (%).
2.2.2 Modified Stover–Kincannon model. The modified Stover–Kincannon model has been successfully used to determine the kinetic parameters in various attached-growth reactors for treating different kinds of wastewater.36,38–41 The maximal COD removal rate in the FB-A2/O2 system can be predicated from the modified Stover–Kincannon model at steady state, using the formula illustrated below: |
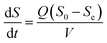 | (9) |
Furthermore, the modified Stover–Kincannon model can also be represented as:45
|
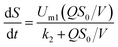 | (10) |
So, it can be converted to the following equation:
|
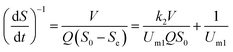 | (11) |
where
Um1 is the constant maximal substrate removal rate (kg (m
3 d)
−1) and
k2 means the saturation rate constant (kg (m
3 d)
−1). The means of the other parameters are represented the same as before.
2.2.3 Monod-biological contact oxidation model. A dynamic model of bio-contact oxidation, based on Monod's equation, can be used to provide a prediction for the degradation of COD in this system. According to Hu et al.,33 the substrate consumption in the reactor is satisfied by the following equation according to the material balance: |
 | (12) |
where (−dS/dt)S and (−dS/dt)A are the removal rates of suspended cells and attached cells (kg (m3 d)−1), respectively, VA is the volume of attached biofilm (m3), and VS is the liquid volume in the reactor (m3).In this study, the kinetic study of two-step biofilm aerobic systems was at the period of steady state. The suspended cells accounted for in the biofilm reactors were much lower than the attached ones and the suspended cells could be ignored. Thus, eqn (12) could be written as:
|
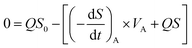 | (13) |
Furthermore, the substrate removal rate by the attached cells could be calculated by the biofilm cells' absolute growth rate (dx/dt)A and its theoretical yield coefficient YA:
|
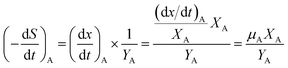 | (14) |
Then:
|
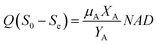 | (15) |
where
N means the volume of carriers in the fixed biofilm reactor (m
3) and
A is the surface area of the carriers (m
2 m
−3).
Furthermore, as one of the empirical models for simulating microbial growth, the Monod model27 has been proven to be applicable for bio-contact oxidation:33
|
 | (16) |
Eqn (16) can be deduced as below:
|
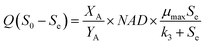 | (17) |
The removal rate in a unit area of carrier is (g m−2 d−1):
|
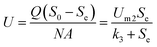 | (18) |
|
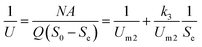 | (19) |
When Se ≪ k3, eqn (18) could be transformed as:
|
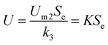 | (20) |
where
μA and
μmax are recognized as the specific growth rate of the attached biofilm and the maximum specific growth rate (d
−1), respectively.

, while
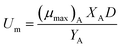
is the maximum substrate removal rate per area of carrier (g m
−2 d
−1), and
k3 is the half-saturation concentration (mg L
−1).
When non-biodegradable harmful compounds exist in the wastewater, the concentration of decomposition bio-refractory substances (Sn) should be reduced. The equation considering the refractory substance could be converted by eqn (18) and (19):
|
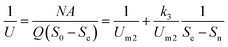 | (22) |
2.3 Analytical methods
The temperature, pH, dissolved oxygen (DO), and alkalinity in the influent and effluent samples in each reactor were analyzed on a daily basis, while COD, NH4+–N, and NO3−–N were measured weekly. The measurements of COD, NH4+–N, NO3–N, and alkalinity were determined according to the standard methods.46 Temperature and pH were monitored by a pH meter (WTW Multi340i). The value of DO was monitored via a portable DO meter (YSI-500).
3. Results and discussion
3.1 Effect of OLR on overall COD removal
The impact of the COD loading rate (QS0/V) (OLR) from the pilot-scale A2/O2 biofilm system along with the effluent COD concentration and its removal efficiency are depicted in Fig. 2. As the figure shows, the general trend of COD removal efficiency increased as the COD load increased from 0.1 kg (m3 d)−1 to 0.25 kg (m3 d)−1, but then obviously decreased with the increasing OLR. The value of effluent COD decreased to 98 mg L−1, with a maximum COD removal efficiency of 92.3% at an optimized OLR of 0.25 kg m−3 d−1. The effluent COD was able to meet the requirements of the Emission Standard of Pollutants for Coking Chemical Industry (GB 16171-2012) and the final effluent COD concentration in this study was lower than that in other related studies (Table 2).
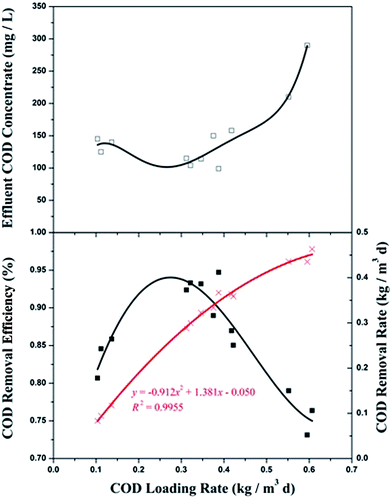 |
| Fig. 2 Variation of effluent COD concentration, COD removal efficiency, and COD removal rate at different OLRs. (□) Effluent COD concentration; (■) COD removal efficiency; (×) COD removal rate. | |
Table 2 Comparison of COD removal in coking wastewater by various processes
Process |
HRT (h) |
NLR kg COD per (m3 d) |
COD effluent concentration (mg L−1) |
Efficiency (average %) |
Reference |
Stripping-SBR |
115 |
0.271 |
206 |
84.1 |
47 |
A–O biofilms |
60 |
0.721 |
200 |
91 |
14 |
UBF-BAF |
46.7 |
0.514 |
185.3 |
81.5 |
20 |
A1–A2–O-MBBR |
103.75 |
0.248 |
122 |
88.6 |
48 |
A1–A2–O-MBBR |
144 |
0.973 |
262.8 |
95.5 |
21 |
FB-A2/O2 |
116 |
0.247 |
92.02 |
92.3 |
This study |
For a high level of OLR (over 0.38 kg m−3 d−1), the COD removal efficiency decreased dramatically from 90% to 73.1%, while the effluent COD concentration accordingly increased considerably. The lower COD removal efficiency indicated that the attached microorganisms could not flourish at a higher level of OLR. One possible reason for this was that the biofilm could have become detached by the strong hydraulic shear at a high level of OLR. On the other hand, the bioactivity of the microorganisms was restrained by some refractory organics accumulated at lower HRT.
The results of the trends of the COD removal rate in the different OLRs indicated that the COD removal rate rapidly increased with the increase in COD loading rate. A high correlation coefficient (R2 = 0.9955) was obtained between the COD loading rate and the COD removal rate, fitting well for the quadratic function. This implied that the total system had a distinguished capability for organics removal. As a high OLR was obtained, the tendency of the COD removal rate gradually became flat, implying that the COD removal rate of the system had reached its threshold. The optimum COD loading rate seemed within the range of 0.175 kg (m3 d)−1 to 0.380 kg (m3 d)−1 at a HRT of 116 h.
3.2 Effect of OLR on the COD removal in each reactor
In order to investigate the COD removal behavior within the whole system, the contribution ratio of each bioreactor in the overall COD removal efficiency at various organic loadings was determined and the results are shown in Fig. 3. As indicated in the figure, the OLR also had a significant impact on COD removal efficiency, either on the overall removal efficiency or the COD removal distribution in each reactor. In the A1 reactor, the average COD removal proportion of the total COD removal was close to 20%. An excessively high or low OLR increases the COD removal efficiency in the anaerobic reactor. Yet, as a pretreatment unit, instead of removing biodegradable COD, the main purpose of the anaerobic reactor was to enhance the biodegradability of the wastewater for partial rupturing and transforming some macromolecular structures, such as PAHs and heterocyclic compounds into the alkyl, acetic acid, and other long-chain organics and low molecular fatty acids.9,20,49 Thus, a moderate range of OLR was maintained to enhance the COD removal efficiency in the subsequent anoxic and two aerobic stages.
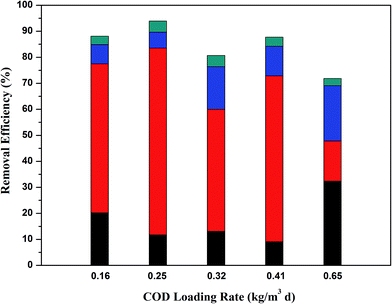 |
| Fig. 3 COD removal ratio of FB-A2/O2 systems at different OLRs ( COD removal in the A1 reactor; COD removal in the A2 reactor; COD removal in the O1 reactor; COD removal in the O2 reactor). | |
For the A2 reactor, the anoxic unit contributed greatly to the total COD removal efficiency at lower OLR, especially within the range of 0.25 kg m−3 d−1 to 0.41 kg m−3 d−1, in which the removal efficiency of COD could reach over 60%. Such a higher removal efficiency of COD was also reported by Zhang et al.9 and Sahariah et al.21 An excessively high COD loading rate would not be suitable for the anoxic degradation of COD due to an incomplete HRT.
For the two-step aerobic reactors, the COD removal efficiency was always higher in the O1 reactor than in the O2 reactor. The probable reason for this was that the molecular products converted by the anaerobic and anoxic stages were preferentially utilized and degraded throughout the O1 reactor. Thus, the remaining COD compositions entering the O2 reactor were biorefractory organics, which led to its lower COD removal efficiency. Furthermore, the calculated data showed that the COD removal efficiency in the two aerobic units at a COD loading rate of 0.65 kg (m3 d)−1 was higher than at the lower COD loading rate due to the relatively adequate degradation of organic compounds under longer HRT.
3.3 Kinetic analyses of COD removal in the A1 reactor
3.3.1 Grau second-order substrate removal model. The coefficients of the Grau second-order substrate removal model in the A1 reactor were determined by plotting eqn (8). According to eqn (8), HRT/E is the slope and HRT is the intercept for the model. As shown in Fig. 4A, a and b were calculated as 329.7721 per day and 35.1395 per day, respectively. The determination coefficient (R2) of Grau second-order substrate removal in the A1 reactor was 0.8835, revealing the unsuitability of the model. Such a lower R2 may be due to the complexity of the raw coking wastewater, which has significant effects on the biomass concentration in the A1 reactor because of its variable quality and toxic and harmful ingredients. Thus,
cannot be considered as an absolute constant during the operation and HRT fails to present a fine linear relationship with the HRT/E.
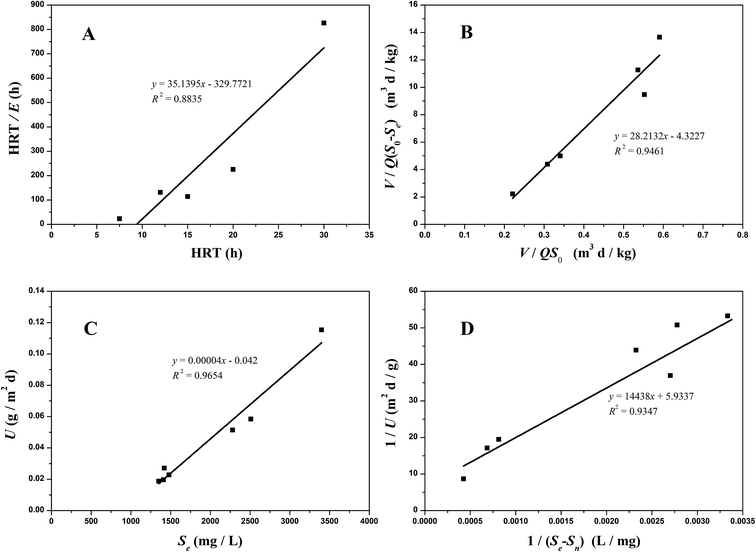 |
| Fig. 4 Substrate removal model plots for COD removal in the A1 reactor ((A) the Grau second-order model; (B) the modified Stover–Kincannon model; (C) Sn calculated; (D) Um and k calculated). | |
3.3.2 Modified Stover–Kincannon model. By applying the modified Stover–Kincannon model in the A1 reactor, the values of k2-A1 and Um1-A1 were obtained as 6.5257 kg (m3 d)−1 and 0.2313 kg (m3 d)−1, respectively, as shown in Fig. 4B. A correlation coefficient of 0.9461 was obtained, confirming a closer fit for predicting COD removal of the A1 unit in this study. For the use of the modified Stover–Kincannon model in the A1 reactor, the expression developed to assume the effluent COD concentrations was as follows: |
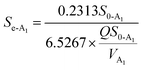 | (23) |
3.3.3 Monod-biological contact oxidation model. The Monod-BCO model was used to describe the COD removal rate of the unit area of carrier in the A1 reactor. At first, Sn needed to be evaluated by the X-intercept before calculating the kinetic constants due to the refractory compounds in the raw wastewater. Thus, a value of Sn-A1 of 1050 mg L−1 was obtained from Fig. 4C. Furthermore, the kinetic constant for COD removal in the A1 reactor was determined from the slope line of 1/U and 1/(Se − S0) using eqn (22). Here, Um2-A1 and k3-A1 were determined as 0.1685 g (m2 d)−1 and 2433.2204 mg L−1 from Fig. 4D, and a correlation coefficient of 0.9347 was achieved. Compared to the Monod-BCO model applied in other reactors, the relatively lower value of R2 may be due to the presence of refractory organics. The effluent COD concentration in the effluent was predicted by this model using eqn (24): |
 | (24) |
3.4 Kinetic studies in the A2 reactor
3.4.1 Grau second-order substrate removal model. As shown in Fig. 5A, the parameters of the Grau second-order model were calculated by plotting HRT/E versus HRT. It was observed that R2 was 0.9859, demonstrating that it was much more suitable for describing the COD performance in the A2 reactor than in the A1 reactor. Moreover, a and b were 7.4102 per day and 3.1057 per day, respectively.
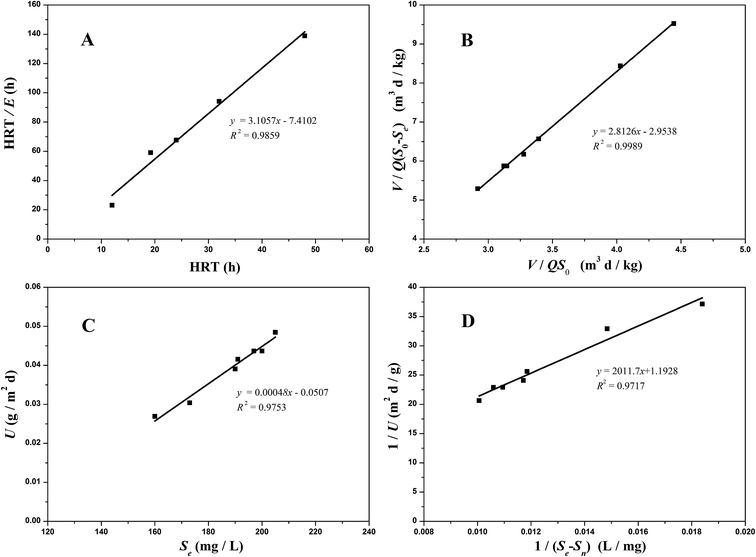 |
| Fig. 5 Substrate removal model plots for COD removal in the A2 reactor ((A) the Grau second-order model; (B) the modified Stover–Kincannon model; (C) Sn calculated; (D) Um and k calculated). | |
3.4.2 Modified Stover–Kincannon model. Fig. 5B illustrates that the R2 correlation coefficients for the modified Stover–Kincannon model in the A2 reactor was 0.9989, which demonstrated that the most favorable linear relationship was between
and
among the four reactors. It was probable that the refractory compounds were obviously reduced or converted through hydrolysis in the A1 reactor. Accordingly, sufficient and steady amounts of biodegradable COD were utilized by the denitrifying bacteria. The crucial parameters for the modified Stover–Kincannon model were confirmed, depending on eqn (11). The saturation value constant (k2-A2) and maximum utilization rate (Um1-A2) were calculated as 0.9522 kg (m3 d)−1 and 0.3385 kg (m3 d)−1, respectively. The equation for predicting the effluent COD concentrations of the A2 reactor obtained from the modified Stover–Kincannon model was as follows: |
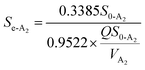 | (25) |
3.4.3 Monod-biological contact oxidation model. Fig. 5C was used to determine the refractory organics Sn-A2, which was calculated to be 105.6 mg L−1. Moreover, plots of 1/U vs. 1/(Se − Sn) are shown in Fig. 5D. The maximum rate of substrate utilization Um2-A2 and the saturation concentration (k3-A2) of the Monod-BCO model were 0.8384 g (m2 d)−1 and 1686.5360 mg L−1, respectively. Compared with the values obtained by the Monod-BCO model in the A1 reactor, it can be seen that the maximum COD utilization rate in the A2 reactor was about 5 times higher than in the A1 reactor. This may be attributed to the substantial reduction of refractory substances Sn, from 1050 mg L−1 to 105.6 mg L−1 in the A1 reactor. On the other hand, along with the accumulation of NOx–N during the steady-state period in the anoxic stage, aromatics compounds with bicyclics and polycyclics were mineralized by the high concentration of denitrifying bacteria, utilizing NOx–N as substrates.50 However, the actual maximum substrate removal rate per area of carrier was 0.1044 g (m2 d)−1, which was only 12.45% of the predicted Um2-A2, implying that the A2 reactor possessed better COD removal potential. |
 | (26) |
3.5 Kinetic studies in the O1 and O2 reactors
3.5.1 Grau second-order substrate removal model. The Grau second-order substrate removal model was also applied to perform the COD removal in the two aerobic reactors (Fig. 6A and 7A). The values for the constant a in the O1 and O2 reactors were 15.7352 per day and 47.4110 per day, respectively, while the values for constant b were 3.4153 per day and 2.7484 per day, respectively. The higher correlation coefficient (R2 = 0.9527 − 0.9701) achieved highlighted the validity of this model in both the O1 and O2 reactors.
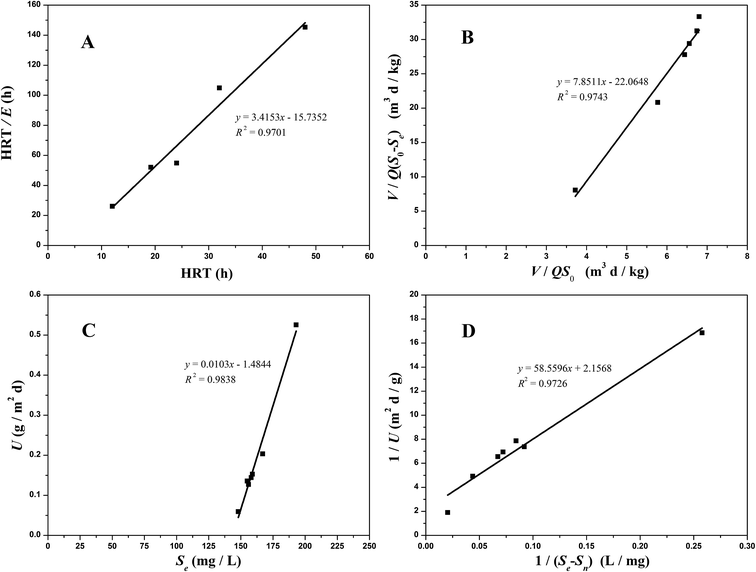 |
| Fig. 6 Substrate removal model plots for COD removal in the O1 reactor ((A) the Grau second-order model; (B) the modified Stover–Kincannon model; (C) Sn calculated; (D) Um and k calculated). | |
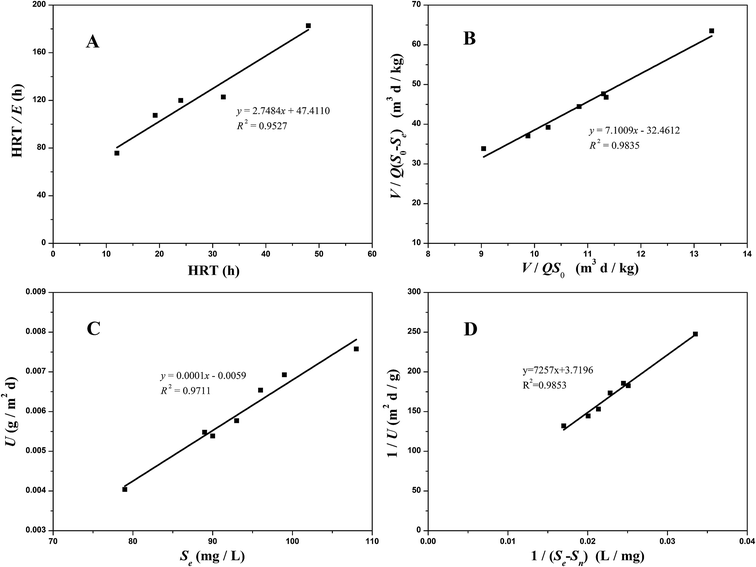 |
| Fig. 7 Substrate removal model plots for COD removal in the O2 reactor ((A) the Grau second-order model; (B) the modified Stover–Kincannon model; (C) Sn calculated; (D) Um and k calculated). | |
3.5.2 Modified Stover–Kincannon model. When the COD removal kinetics of the O1 and O2 reactors was plotted by the modified Stover–Kincannon model (Fig. 6B and 7B), best-fit lines of 0.9743 and 0.9835 were obtained, respectively.For the O1 reactor, from the slope and intercept, the kinetic parameters for COD removal were calculated as the maximum utilization rate Um1-O1 of 0.0454 kg (m3 d)−1 and the saturation value constant k2-O1 of 0.3561 kg (m3 d)−1. For the O2 reactor, the model parameters evaluated were Um1-O2 of 0.0308 kg (m3 d)−1 and k2-O2 of 0.2188 kg (m3 d)−1, respectively. Instead of the A2 reactor with the maximum COD removal rate of 0.3385 kg (m3 d)−1, the COD removal efficiency of the O1 and O2 kinetic indexes decreased obviously, suggesting that the anoxic reactor significantly contributed to COD removal of the whole system. Based on the curves in Fig. 6B and 7B, the COD concentration in the effluents from the O1 and O2 reactors by this model were simulated via utilizing eqn (27) and (28):
|
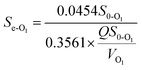 | (27) |
|
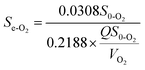 | (28) |
3.5.3 Monod-BCO model. The Monod-BCO model coefficients in the O1 reactor were obtained from Fig. 6C and D. The value of Sn-O1 taken from Fig. 6C was 144.12 mg L−1 based on eqn (21), while the numerical computation of k3-O1 and Um2-O1 were estimated as 27.337 mg L−1 and 0.464 g (m2 d)−1, respectively. The diagram displayed good co-linearity as the R2 was 0.9728. Compared to the estimated value of Sn-A2 obtained in the A2 reactor, the concentration of Sn-O1 increased slightly. One of the probable explanations for this was that some chemical bonds belonging to macromolecular organic substances were not broken entirely while other organics were transformed at the same time. Zhang et al.9 also reported that some refractory substances concentration in coking wastewater increased after the anoxic reactor. In the O2 reactor, the corresponding values of Sn-O2, k3-O2, and Um2-O2 were 49.167 mg L−1, 1951.0162 mg L−1, and 0.2689 g (m2 d)−1, respectively (Fig. 7C and D). The correlation of determination was 0.9853, thus proving the good availability of the application of the Monod-BCO model for the removal rate of COD in the O2 unit. The predicted effluent concentration of COD in the O1 and O2 reactors could be given by eqn (29) and (30), respectively: |
 | (29) |
|
 | (30) |
From the values of the maximum COD utilization rate obtained by the Monod-BCO model and the modified Stover–Kincannon model, it could be seen that in contrast with the O1 reactor, Um in the O2 reactor was relatively low, which may be due to the presence of autotrophic nitrifying bacteria, such as nitrobacter, nitrococcus, nitrosomonas, and nitrosococcus, holding predominance in the microbial community structure in the second aerobic reactor.51 On the other hand, the inhibitory effects of the refractory organics on microorganisms were largely reduced in the O2 reactor owing to preferential removal of the macromolecules in the first aerobic reactor.
3.6 Model verifications and kinetic comparisons
As discussed above, three kinetic models were implemented to develop a suitable model for COD removal efficiency of a multi-step biofilm system. The crucial parameters obtained from the models are summarized in Table 3. It could be observed that the modified Stover–Kincannon model and the Monod-BCO model held more advantages than the Grau second-order substrate model in predicting organics removal in the whole system. Although the Grau second-order substrate removal model was proven to have affinities with the modified Stover–Kincannon model and they converted to each other when Um was equal to k,52 it did not perform well in the A1 reactor. The modified Stover–Kincannon model has been widely used in various reactors treating different kinds of wastewater.53,54 In this study, it also was used as a fitting precision model to describe COD removal of each unit in this system, partially owing to the addition of COD loading rate (QSi/V) to the model. Hence, it is capable of predicting substrate removal under any loading conditions. Furthermore, the Stover–Kincannon model did not need to consider additional hard-to-measure parameters for substrate removal prediction.53
Table 3 Summary of kinetic constants in the different reactors
Reactors |
Models |
Kinetic constants |
Unit |
Values |
R2 |
Average error between the measured and predicted values |
A1 |
Grau second-order substrate removal model |
a |
d−1 |
329.7721 |
0.8835 |
— |
b |
d−1 |
35.1395 |
Modified Stover–Kincannon model |
Um1 |
kg (m3 d)−1 |
0.2313 |
0.9461 |
45.736 |
k2 |
kg (m3 d)−1 |
6.5257 |
Monod-BCO model |
Um2 |
g (m2 d)−1 |
0.1685 |
0.9347 |
10.302 |
k3 |
mg L−1 |
2433.2204 |
A2 |
Grau second-order substrate removal model |
a |
d−1 |
7.4102 |
0.9859 |
— |
b |
d−1 |
3.1057 |
Modified Stover–Kincannon model |
Um1 |
kg (m3 d)−1 |
0.3385 |
0.9989 |
96.387 |
k2 |
kg (m3 d)−1 |
0.9522 |
Monod-BCO model |
Um2 |
g (m2 d)−1 |
0.8384 |
0.9717 |
0.2202 |
k3 |
mg L−1 |
1686.5360 |
O1 |
Grau second-order substrate removal model |
a |
d−1 |
15.7352 |
0.9701 |
— |
b |
d−1 |
3.4153 |
Modified Stover–Kincannon model |
Um1 |
kg (m3 d)−1 |
0.0454 |
0.9743 |
1.523 |
k2 |
kg (m3 d)−1 |
0.3561 |
Monod-BCO model |
Um2 |
g (m2 d)−1 |
0.4654 |
0.9726 |
41.488 |
k3 |
mg L−1 |
27.3370 |
O2 |
Grau second-order substrate removal model |
a |
d−1 |
47.4110 |
0.9527 |
— |
b |
d−1 |
2.7484 |
Modified Stover–Kincannon model |
Um1 |
kg (m3 d)−1 |
0.0308 |
0.9835 |
15.997 |
k2 |
kg (m3 d)−1 |
0.2188 |
Monod-BCO model |
Um2 |
g (m2 d)−1 |
0.2689 |
0.9853 |
1.009 |
k3 |
mg L−1 |
1951.0162 |
Table 4 gives a comparison of the kinetics results between this study and other related studies by two models. In the modified Stover–Kincannon model, from the table, it can be seen that the maximum COD removal rate (Umax) and the saturation constant (KB) in this study were much lower than in other studies due to the presence of recalcitrant organics in refractory coking wastewater, similar with the value reported by Sponza et al. However, similar kinetic parameters were obtained with other related studies when utilizing the Monod model, which was the most applicable model in our study.
Table 4 Kinetic comparisons of this study and other related studies
Model |
Wastewater |
Process |
COD mg L−1 |
OLR kg m−3 d−1 |
Umax kg m−3 d−1 |
KB kg m−3 d−1 |
References |
Modified Stover–Kincannon |
Distillery spent wash |
Anaerobic fixed film bioreactor |
11 000–19 000 |
5–20 |
2 |
1.69 |
55 |
2,4-DCP wastewater |
UASB |
3000 |
3.6–36 |
0.008 |
0.0346 |
56 |
Weak industrial wastewater |
UASB |
704.55 ± 54.33 |
0.8052–0.049 |
1.502 |
2.924 |
57 |
Coking wastewater |
Anaerobic |
900–1800 |
0.16–0.65 |
0.2313 |
6.5257 |
This study |
Anoxic |
0.3385 |
0.9522 |
Oxic-1 |
0.0454 |
0.3561 |
Oxic-2 |
0.0308 |
0.2188 |
Model |
Wastewater |
Process |
COD mg L−1 |
OLR kg m−3 d−1 |
Umax kg m−3 d−1 |
KB mg L−1 |
References |
Monod |
2,4-DCP wastewater |
UASB |
3000 |
6–34 |
2.73 |
560 |
56 |
Tobacco-waste leachate |
SBR |
500–3000 |
0.25–1.50 |
3.26 |
5450 |
58 |
Coking wastewater |
Anaerobic |
900–1800 |
0.16–0.65 |
0.6571 |
2433.2204 |
This study |
Anoxic |
3.2698 |
1686.536 |
Oxic-1 |
0.1098 |
27.337 |
Oxic-2 |
1.0487 |
1951.0162 |
3.7 Process optimization via kinetic modeling
Although both the modified Stover–Kincannon model and the Monod-BCO model gave high correlation coefficients (R2 > 0.95), the Monod-BCO model was shown to be a bit more appropriate for the description of COD removal under actual conditions as the Monod-BCO model not only describes the biodegradation kinetics53 but also contains the total area of carriers and refractory compounds in its calculations. Also, the obtained Monod-BCO model could be utilized to optimize the process of the FB-A2/O2 system treating coking wastewater. The value of the half-saturation constant (k) could represent the substrate affinity, which means a higher COD removal efficiency when higher k values are obtained. The k values obtained by the Monod-BCO model of a biofilm for the A1, A2, O1, and O2 reactors were 2433.2204 mg L−1, 1686.5360 mg L−1, 27.3370 mg L−1, and 1951.0162 mg L−1, respectively. Compared with the previously reported values for coking wastewater treatment,23,59 our system had significantly higher substrate affinity in this study. The average k value obtained in this study was 1524.527 mg L−1, which was higher than the COD concentration in raw coke plant wastewater. It was certificated that the pilot-scale A2/O2 biofilm system had a maximum potential of COD removal efficiency; that is, the effluent COD concentration set by the system could be much lower than the presented result. Moreover, the actual average COD removal rate per area of the carrier could not completely reach the predicted Um value in the system (only about 19.18% of the Um). This may partially be attributed to an undesirable substrate distribution, especially in the O2 reactor. Uneven organics provision led to a decreased utilized rate of the carbon source. Moreover, many studies have reported that some non-biodegradable organic compounds, like NHCs, in anaerobic or aerobic units tend to be easily mineralized under anoxic conditions. From this perspective, the potentials of the A2, O1, and O2 reactors' degradation for COD might not be made full use of in single-feeding systems, since all raw wastewater only directly flows into the anaerobic tank. To ensure the combined biodegradation of organics under different redox conditions to enhance the process for COD removal, a step-feeding process is expected to be an alternative option. However, studies on the flow distributed ratio within each reactor also should be further optimized in any step-feeding process in the future.
4. Conclusion
In this study, the COD removal characteristics and biokinetics models of this were investigated utilizing a pilot-scale FB-A2/O2 system effectively treating high-strength coking wastewater. Some conclusions were drawn, as listed below:
(1) The COD removal rate increases and then flattens with the increase in the influent COD loading rate. The maximum COD removal efficiency achieved was 92.3% with an effluent COD concentration of 98 mg L−1 at an OLR of 0.25 kg (m3 d)−1. The results indicated that the anoxic and first oxic units played significant roles in removing the COD from coking wastewater.
(2) The modified Stover–Kincannon model and Monod-biological contact oxidation model were proven to be applicable to estimate the COD removal performance in each unit. Model validation demonstrated that the Monod-BCO model fitted better for COD removal, even though the modified Stover–Kincannon model had a higher correlation coefficient. According to the Monod-BCO model, The theoretical COD maximum removal rates (Um) of the A1, A2, O1, and O2 units were 0.1685 g (m2 d)−1, 0.8384 g (m2 d)−1, 0.4654 g (m2 d)−1, and 0.2689 g (m2 d)−1, respectively, and a large amount of refractory substance (Sn) could be decreased through utilizing the system.
(3) The system also showed great substrate affinity, whereby the corresponding k values of each rector were 2433.2204 mg L−1, 1686.5360 mg L−1, 27.3370 mg L−1, and 1951.0162 mg L−1, respectively.
(4) Step-feeding after the A1 reactor was suggested to optimize the system on the basis of the Monod-BCO kinetic model in this study.
Acknowledgements
This work was supported by National Natural Science Foundation of Youth in China (No. 21607111), China Postdoctoral Science Foundation (No. 2015M571287), Open project in State Key Laboratory of Urban Water Resources and Environment, Harbin Institute of Technology (No. QA201620) and Graduate Students Joint Training Base for Talents Training Project in Shanxi (No. 2016JD16). The authors also thank Dr David Howard for English language editing of this manuscript.
References
- M. Han, G. Li, N. Sang and Y. Dong, Investigating the bio-toxicity of coking wastewater using Zea mays L. assay, Ecotoxicol. Environ. Saf., 2011, 74, 1050–1056 CrossRef CAS PubMed.
- S. Zhou, H. Watanabe, C. Wei, D. Wang, J. Zhou, N. Tatarazako, S. Masunaga and Y. Zhang, Reduction in toxicity of coking wastewater to aquatic organisms by vertical tubular biological reactor, Ecotoxicol. Environ. Saf., 2015, 115, 217–222 CrossRef CAS PubMed.
- E. I. Garcia-Pena, P. Zarate-Segura, P. Guerra-Blanco, T. Poznyak and I. Chairez, Enhanced Phenol and Chlorinated Phenols Removal by Combining Ozonation and Biodegradation, Water, Air, Soil Pollut., 2012, 223, 4047–4064 CrossRef CAS.
- G. Moussavi and M. Heidarizad, The performance of SBR, SCR, and MSCR for simultaneous biodegradation of high concentrations of formaldehyde and ammonia, Sep. Purif. Technol., 2011, 77, 187–195 CrossRef CAS.
- P. Xu, H. Han, H. Zhuang, B. Hou, S. Jia, D. Wang, K. Li and Q. Zhao, Anoxic degradation of nitrogenous heterocyclic compounds by activated sludge and their active sites, J. Environ. Sci., 2015, 31, 221–225 CrossRef PubMed.
- W. Zhang, C. Wei, B. Yan, C. Feng, G. Zhao, C. Lin, M. Yuan, C. Wu, Y. Ren and Y. Hu, Identification and removal of polycyclic aromatic hydrocarbons in wastewater treatment processes from coke production plants, Environ. Sci. Pollut. Res. Int., 2013, 20, 6418–6432 CrossRef CAS PubMed.
- X. Yu, C. Wei, H. Wu, Z. Jiang and R. Xu, Improvement of biodegradability for coking wastewater by selective adsorption of hydrophobic organic pollutants, Sep. Purif. Technol., 2015, 151, 23–30 CrossRef CAS.
- P. Lai, H. Z. Zhao, M. Zeng and J. R. Ni, Study on treatment of coking wastewater by biofilm reactors combined with zero-valent iron process, J. Hazard. Mater., 2009, 162, 1423–1429 CrossRef CAS PubMed.
- M. Zhang, J. H. Tay, Y. Qian and X. S. Gu, Coke plant wastewater treatment by fixed biofilm system for COD and NH3–N removal, Water Res., 1998, 32, 519–527 CrossRef CAS.
- H.-q. Li, H.-j. Han and M.-a. Du, Effect of nitrate concentration on performance of pre-denitrification moving bed biofilm reactor system in treating coal gasification wastewater, Desalin. Water Treat., 2013, 51, 5996–6002 CrossRef CAS.
- X. Yuan, H. Sun and D. Guo, The removal of COD from coking wastewater using extraction replacement–biodegradation coupling, Desalination, 2012, 289, 45–50 CrossRef CAS.
- Q. Gu, T. Sun, G. Wu, M. Li and W. Qiu, Influence of carrier filling ratio on the performance of moving bed biofilm reactor in treating coking wastewater, Bioresour. Technol., 2014, 166, 72–78 CrossRef CAS PubMed.
- J. Y. Jing, J. Feng, W. Y. Li and Y. Xu, Removal of COD from coking-plant wastewater in the moving-bed biofilm sequencing batch reactor, Korean J. Chem. Eng., 2009, 26, 564–568 CrossRef CAS.
- P. Lai, H. Zhao, Z. Ye and J. Ni, Assessing the effectiveness of treating coking effluents using anaerobic and aerobic biofilms, Process Biochem., 2008, 43, 229–237 CrossRef CAS.
- L. Rodríguez-Hernández, A. L. Esteban-García and I. Tejero, Comparison between a fixed bed hybrid membrane bioreactor and a conventional membrane bioreactor for municipal wastewater treatment: A pilot-scale study, Bioresour. Technol., 2014, 152, 212–219 CrossRef PubMed.
- Z. Yu, Treatment of coke plant wastewater by A/O fixed biofilm system, Sci. China, Ser. B: Chem., 2005, 48, 489–496 CrossRef CAS.
- S. Shi, Y. Qu, Q. Ma, X. Zhang, J. Zhou and F. Ma, Performance and microbial community dynamics in bioaugmented aerated filter reactor treating with coking wastewater, Bioresour. Technol., 2015, 190, 159–166 CrossRef CAS PubMed.
- P. M. Sutton and M. Hoeksema, Biological fluidized-bed treatment of wastewater from byproduct coking operations: Full-scale case history, Water Environ. Res., 1999, 71, 5–9 CrossRef CAS.
- H. S. Ou, C. H. Wei, H. Z. Wu, C. H. Mo and B. Y. He, Sequential dynamic artificial neural network modeling of a full-scale coking wastewater treatment plant with fluidized bed reactors, Environ. Sci. Pollut. Res. Int., 2015, 22, 15910–15919 CrossRef CAS PubMed.
- S. Zhu and J. Ni, Treatment of coking wastewater by a UBF-BAF combined process, J. Chem. Technol. Biotechnol., 2008, 83, 317–324 CrossRef CAS.
- B. P. Sahariah, J. Anandkumar and S. Chakraborty, Treatment of coke oven wastewater in an anaerobic–anoxic–aerobic moving bed bioreactor system, Desalin. Water Treat., 2015, 57, 14396–14402 CrossRef.
- X. Zhou, Y. Li, Y. Zhao and X. Yue, Pilot-scale anaerobic/anoxic/oxic/oxic biofilm process treating coking wastewater, J. Chem. Technol. Biotechnol., 2013, 88, 305–310 CrossRef CAS.
- X. Wu, Y. Yang, G. Wu, J. Mao and T. Zhou, Simulation and optimization of a coking wastewater biological treatment process by activated sludge models (ASM), J. Environ. Manage., 2016, 165, 235–242 CrossRef CAS PubMed.
- M. K. Sharma and A. A. Kazmi, Substrate Removal Kinetics of Domestic Wastewater Treatment in a Two-Stage Anaerobic System, Sep. Sci. Technol., 2015, 50, 2752–2758 CAS.
- E. Debik and T. Coskun, Use of the Static Granular Bed Reactor (SGBR) with anaerobic sludge to treat poultry slaughterhouse wastewater and kinetic modeling, Bioresour. Technol., 2009, 100, 2777–2782 CrossRef CAS PubMed.
- L. Sun, S. Wan, Z. Yu, Y. Wang and S. Wang, Anaerobic biological treatment of high strength cassava starch wastewater in a new type up-flow multistage anaerobic reactor, Bioresour. Technol., 2012, 104, 280–288 CrossRef CAS PubMed.
- J. Monod, The Growth of Bacterial Cultures, Annu. Rev. Microbiol., 1949, 3, 371–394 CrossRef CAS.
- T. Chen, P. Zheng, L. Shen, S. Ding and Q. Mahmood, Kinetic characteristics and microbial community of Anammox-EGSB reactor, J. Hazard. Mater., 2011, 190, 28–35 CrossRef CAS PubMed.
- C. T. Goudar and T. G. Ellis, Explicit oxygen concentration expression for estimating extant biodegradation kinetics from respirometric experiments, Biotechnol. Bioeng., 2001, 75, 74–81 CrossRef CAS PubMed.
- A. O. Ibeje, Mathematical Modelling of Cassava Wastewater Treatment Using Anaerobic Baffled Reactor, Am. J. Eng. Res., 2013, 2, 128–134 Search PubMed.
- S. Macé, J. Dosta, A. Galí and J. Mata-Alvarez, Optimization of Biological Nitrogen Removal via Nitrite in a SBR Treating Supernatant from the Anaerobic Digestion of Municipal Solid Wastes, Ind. Eng. Chem. Res., 2006, 45, 2787–2792 CrossRef.
- Z. Y. Pan, S. Q. Yu and H. F. Huang, Treatment of high concent ration pesticide wastewater by pressurized biochemical process, The 10th Asia Pacific Confederation of Chemical Engineering Congress, Japan, 2004, pp. 128–135 Search PubMed.
- J. C. Hu and X. S. Gu, Research on the kinetic model of wastewater treatment by using the bio-contact oxidation, China Water Wastewater, 1987, 5–9 Search PubMed.
- E. L. Stover and D. F. Kincannon, Presented in Part at the Proceedings of the 1st International Conference on Fixed Film Biological Processes, Ohio, 1982 Search PubMed.
- S. Sandhya and K. Swaminathan, Kinetic analysis of treatment of textile wastewater in hybrid column upflow anaerobic fixed bed reactor, Chem. Eng. J., 2006, 122, 87–92 CrossRef CAS.
- A. Noroozi, M. Farhadian and A. Solaimanynazar, Kinetic coefficients for the domestic wastewater treatment using hybrid activated sludge process, Desalin. Water Treat., 2014, 57, 4439–4446 Search PubMed.
- J. Wang, J. Yan and W. Xu, Treatment of dyeing wastewater by MIC anaerobic reactor, Biochem. Eng. J., 2015, 101, 179–184 CrossRef CAS.
- S. A. Kordkandi and L. Berardi, Comparing new perspective of hybrid approach and conventional kinetic modelling techniques of a submerged biofilm reactor performance, Biochem. Eng. J., 2015, 103, 170–176 CrossRef CAS.
- A. Akhbari, A. A. L. Zinatizadeh, P. Mohammadi, Y. Mansouri, M. Irandoust and M. H. Isa, Kinetic modeling of carbon and nutrients removal in an integrated rotating biological contactor-activated sludge system, Int. J. Environ. Sci. Technol., 2012, 9, 371–378 CrossRef CAS.
- J. H. Ahn and C. F. Forster, Kinetic analyses of the operation of mesophilic and thermophilic anaerobic filters treating a simulated starch wastewater, Process Biochem., 2000, 36, 19–23 CrossRef CAS.
- M. Faridnasr, B. Ghanbari and A. Sassani, Optimization of the moving-bed biofilm sequencing batch reactor (MBSBR) to control aeration time by kinetic computational modeling: Simulated sugar-industry wastewater treatment, Bioresour. Technol., 2016, 208, 149–160 CrossRef CAS PubMed.
- W. Y. Li, P. M. Xu, Y. Xu and J. Y. Jing, Study on Kinetic Model of Immobilized Dominant Bacteria for Treatment of COD in Coke Plant Wastewater, Energy Sources, Part A, 2009, 31, 1654–1659 CrossRef CAS.
- P. Grau, M. Dohányos and J. Chudoba, Kinetics of multicomponent substrate removal by activated sludge, Water Res., 1975, 9, 637–642 CrossRef.
- S. M. Borghei, M. Sharbatmaleki, P. Pourrezaie and G. Borghei, Kinetics of organic removal in fixed-bed aerobic biological reactor, Bioresour. Technol., 2008, 99, 1118–1124 CrossRef CAS PubMed.
- H. Yu, F. Wilson and J.-H. Tay, Kinetic analysis of an anaerobic filter treating soybean wastewater, Water Res., 1998, 32, 3341–3352 CrossRef CAS.
- Standard Monitoring & Analytical Methods for the Examination of Water and Wastewater, ed. E. P. A. Chinese, China Environmental Science Press, Beijing, 2002 Search PubMed.
- E. Maranon, I. Vazquez, J. Rodriguez, L. Castrillon, Y. Fernandez and H. Lopez, Treatment of coke wastewater in a sequential batch reactor (SBR) at pilot plant scale, Bioresour. Technol., 2008, 99, 4192–4198 CrossRef CAS PubMed.
- X. L. Shi, X. B. Hu, Z. Wang, L. L. Ding and H. Q. Ren, Effect of reflux ratio on COD and nitrogen removals from coke plant wastewaters, Water Sci. Technol., 2010, 61, 3017–3025 CrossRef CAS PubMed.
- X. X. Wei, Z. Y. Zhang, Q. L. Fan, X. Y. Yuan and D. S. Guo, The effect of treatment stages on the coking wastewater hazardous compounds and their toxicity, J. Hazard. Mater., 2012, 239–240, 135–141 CrossRef CAS PubMed.
- K. J. Rockne and S. E. Strand, Anaerobic biodegradation of naphthalene, phenanthrene, and biphenyl by a denitrifying enrichment culture, Water Res., 2001, 35, 291–299 CrossRef CAS PubMed.
- X. Zhou, Y. X. Li and Y. Zhao, Removal characteristics of organics and nitrogen in a novel four-stage biofilm integrated system for enhanced treatment of coking wastewater under different HRTs, RSC Adv., 2014, 4, 15620–15629 RSC.
- M. Işik and D. T. Sponza, Substrate removal kinetics in an upflow anaerobic sludge blanket reactor decolorising simulated textile wastewater, Process Biochem., 2005, 40, 1189–1198 CrossRef.
- S. Q. Ni, S. Sung, Q. Y. Yue and B. Y. Gao, Substrate removal evaluation of granular anammox process in a pilot-scale upflow anaerobic sludge blanket reactor, Ecol. Eng., 2012, 38, 30–36 CrossRef.
- X. W. Huang, Q. Y. Wei, K. Urata, Y. Tomoshige, X. H. Zhang and Y. Kawagoshi, Kinetic study on nitrogen removal performance in marine anammox bacterial culture, J. Biosci. Bioeng., 2014, 117, 285–291 CrossRef CAS PubMed.
- B. K. Acharya, H. Pathak, S. Mohana, Y. Shouche, V. Singh and D. Madamwar, Kinetic modelling and microbial community assessment of anaerobic biphasic fixed film bioreactor treating distillery spent wash, Water Res., 2011, 45, 4248–4259 CrossRef CAS PubMed.
- D. T. Sponza and A. Ulukoy, Kinetic of carbonaceous substrate in an upflow anaerobic sludge sludge blanket (UASB) reactor treating 2,4-dichlorophenol (2,4-DCP), J. Environ. Manage., 2008, 86, 121–131 CrossRef CAS PubMed.
- S. M. Abtahi, M. M. Amin, R. Nateghi and A. Vosoogh, Prediction of effluent COD
concentration of UASB reactor using kinetic models of Monod, Contois, second-order Grau and modified Stover–Kincannon, Int. J. Environ. Health Eng., 2013, 2, 12 CrossRef.
- M. Vuković, I. Ćosić and D. Kučić, Biodegradation kinetics of tobacco waste leachate by activated sludge in SBR, Chem. Biochem. Eng. Q., 2012, 26, 191–198 Search PubMed.
- C. J. Dong and Q. Y. Pan, Kinetic Analysis for COD Removal in Actual Coking Wastewater through an Micro-Aerobic EGSB Reactor, Appl. Mech. Mater., 2014, 700, 455–459 CrossRef.
|
This journal is © The Royal Society of Chemistry 2017 |