DOI:
10.1039/C7RA00191F
(Paper)
RSC Adv., 2017,
7, 12856-12870
Cerium-doped mesoporous-assembled SiO2/P25 nanocomposites with innovative visible-light sensitivity for the photocatalytic degradation of organic dyes
Received
5th January 2017
, Accepted 10th February 2017
First published on 24th February 2017
Abstract
SiO2/P25 (SP) composites doped with different contents of cerium were prepared by a hydrothermal process at a relatively low temperature in this experiment. The resulting Ce–SiO2/P25 (CSP) composites were successfully characterized by X-ray diffraction (XRD), X-ray photoelectron spectroscopy (XPS), scanning electron microscopy (SEM), high resolution transmission electron microscopy (HRTEM), N2-physisorption, UV-vis diffuse reflectance spectroscopy (DRS), and photoluminescence spectroscopy (PL). The photocatalytic activities of the as-obtained catalysts were evaluated for the degradation of organic dyes, including Methylene Blue (MB) and Reactive Red 4 (RR4), under visible-light irradiation. The best results were obtained for a cerium loading of 5 mM. The CSP-5 nanoparticle showed the 91.8% decomposition of MB and 90.2% decomposition of RR4 in the liquid phase at room temperature under visible-light irradiation in a photoreactor, and the corresponding hydrogen evolution rate was 2.315 mmol g−1, which was more efficient than that of pristine P25. Remarkably enhanced activities towards the photodecomposition of several organic compounds were observed depending on the synergistic effect between silicon and cerium. The Ce–SiO2/P25 structure leads to efficient light harvesting into the visible-light region by forming new energy levels inside the nanoparticles and then electron–hole recombination could be effectively inhibited. The BET surface area measurement was used to provide an insight into the enhanced photocatalytic activity of the CSP composites, which was also understood to be because of the relative enhancement of the adsorption of organic molecules on the photocatalyst surface. In addition, the effect of the initial pH values on the photocatalytic degradation of different dyes using CSP-5 was investigated. Finally, a good recyclability of CSP-5 was demonstrated compared to that of pristine P25. Herein, the photocatalytic mechanism has been concretely explained.
1. Introduction
The incomplete removal of organic dyes and other emerging pollutants in conventional wastewater treatment plants has been identified as the main route by which anthropogenic pollutants reach aqueous environments.1 Consequently, the presence of dyes has become ubiquitous in natural waters, even to the extent of entering drinking water facilities.2 Since Fujishima reported the generation of oxygen and hydrogen using titania electrodes under the irradiation of light in 1972,3 photocatalysis has been regarded as one of the most effective and economical ways to remove organic pollutants from wastewater. Titanium dioxide (TiO2) is the most used semiconductor for the photocatalytic degradation of organic compounds due to its interesting characteristics such as low-cost, non-toxicity, high stability, and high photocatalytic activity.4 TiO2 is widely used in photocatalysis,5,6 self-cleaning coatings,7 dye-sensitized solar cells,8 nanoparticles-coated facemasks,9 antibacterial coatings on medical devices,10 and in optoelectronic and energy storage devices.11 Commercial photocatalytic TiO2 is usually available in the form of powder (Evonik Degussa Aeroxide P25) and has excellent activity. Its activity is based on the absorption of a photon of appropriate energy (about 3.2 eV) and the creation of charge carriers (holes-h+ and electrons-e−) that can oxidize organic pollutants into CO2 and H2O.12–14 Despite such excellent properties of TiO2, there are certain problems associated with TiO2 that hinder its commercialization, including agglomeration of the nanoparticles,15 which could result in a decrease in surface area upon thermal treatment,16 the recombination of photogenerated electron–hole pairs,17 the lack of visible-light photoactivity due to its wide band gap (Eg = 3.2 eV),18 and difficulty in recovery of the photocatalysts from aqueous suspension.
To solve most of these problems, titania and other functional metal oxide nanoparticles can be doped with thermally stable, low-cost, and high surface area materials, such as SiO2, ZrO2, MoO3, and Fe2O3.8,19 Silica is one of the best materials for preparing cerium-doped SiO2/TiO2 materials due to its surface chemistry and adsorption capacity,20 easy and controllable preparation by the Stöber method,21 optical transparency in the TiO2 absorption region, and high thermal and mechanical stability.22 Its surface silicon hydroxyl groups are beneficial for the adsorption of pollutants and the incorporation of an amorphous phase is effective to minimize the agglomeration of TiO2 particles.23,24 For example, some literature studies have reported SiO2–TiO2 heterogeneous systems exhibiting better photocatalytic activity than pristine TiO2 probably since the adsorption of organic compounds was improved and the aggregation of TiO2 nanoparticles was minimized, thus leaving a higher exposed surface area. They also reported that the photocatalytic activity and thermal stability of SiO2-coated TiO2 nanoparticles can be enhanced more efficiently than pure TiO2 powder.25–30
It is recognized that the impurities induced by metal ions doping into the TiO2 could efficiently narrow its band gap and extend the absorption edge into the visible-light range, thus improving the photocatalytic activity of TiO2 under visible-light irradiation.31–34 Among these, TiO2 doped with Ce ions has stimulated extensive research attention due to its special 4f electron orbital structure, which can increase the separation rate of photogenerated charges.35–37 Cerium is the most favored dopant among the lanthanide ions due to its low cost and ability to shift between CeO2 and Ce2O3 in oxidizing and reducing conditions.38 Previous studies have confirmed that the variable valences of Ce, such as Ce4+ and Ce3+, make TiO2 possess excellent characteristics for transferring electrons and enhancing the visible-light-absorption capability.39 In addition, cerium doping increases the formation of oxygen vacancies with a relatively high transfer capacity of oxygen species.40 Electronically, the effect of cerium on the band gap of TiO2 has been explained as being due to an n-type impurity band at the interface between the titanium and cerium oxides.41
In this study, first, visible-light-activated cerium-doped SiO2/P25 (CSP) nanocomposites were synthesized by the common hydrothermal method which has been extensively employed. The synthesized cerium-doped SiO2/TiO2 materials were thoroughly characterized by XRD, N2 adsorption–desorption, SEM-EDS, TEM, XPS, UV-vis diffuse reflectance spectroscopy, and PL to determine their morphology and structural and optical properties. Second, both Methylene Blue (MB) and Reactive Red 4 (RR4) were selected as target contaminants to evaluate the photocatalytic activity of the cerium-doped SiO2/P25 under visible-light irradiation. Organic dyes frequently occur globally in water sources and can severely affect the health of humans and animals when consumed in toxic water or when exposed to them. In particular, either cationic MB or anionic RR4 is one of the most commonly found organic dyes in surface waters that serve as sources of drinking water supply. In this study, the application of the cerium-doped SiO2/P25 for the treatment of water contaminated with MB and RR4 was evaluated.
2. Experimental
2.1. Materials
All the reagents and solvents used for the synthesis and analysis of the catalysts were commercially available and used as received without further purification. Commercially available TiO2 in powder form P25 was from Evonik (Germany). Reactive Red 4 dye (RR4, chemical formula: C32H23ClN8Na4O14S4) and Methylene Blue (MB, chemical formula: C16H18ClN3S) simulated as the organic pollutants were provided by Aladdin. Cerium nitrate hexahydrate (Ce(NO3)3·6H2O, 99.99%) and tetraorthosilicate (TEOS, 99.99%) were purchased from Sigma-Aldrich and used as the cerium and silicon source, respectively. Absolute ethanol and deionized water were used for the preparation of the catalysts. Furthermore, hydrochloric acid and sodium hydroxide solution were used to adjust the initial pH values of the organic dye solution in the photocatalytic experiments. Table 1 provides a summary of the organic pollutants models used in this work.
Table 1 Summary of the organic pollutants in this study
Organic pollutants |
Chemical structure |
Chemical formula |
Molar mass (g mol−1) |
λmax (nm) |
Methylene Blue |
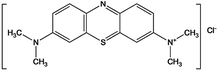 |
C16H18ClN3S |
319.85 |
664 |
Reactive Red 4 |
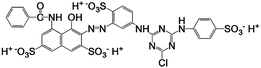 |
C32H23ClN8Na4O14S4 |
995.23 |
517 |
2.2. Methods
2.2.1. Preparation of the Ce–SiO2/P25 (CSP) composites. The modified catalysts were prepared by the following steps. 2.0 g of P25 nanoparticle was first suspended in 50 mL of deionized water with sodium hexametaphosphate serving as the dispersant by ultrasonic dispersion for 30 min. The solution was under continuous stirring while 2.5 mL TEOS and an appropriate amount of Ce(NO3)3 solution was added dropwise at a slow speed. The pH of the solution was adjusted to 7. Then, the suspension was transferred to a Teflon-lined stainless steel autoclave, followed by a hydrothermal treatment at 130 °C for 12 h. Finally, the CSP composites were washed with deionized water and ethanol repeatedly and dried under vacuum at 80 °C for 12 h. For short, hereafter CSP-1, CSP-3, CSP-5, and CSP-7 denote the samples prepared in 1, 3, 5, and 7 mM Ce(NO3)3 solution, respectively. For comparison, SiO2/P25 (SP) was prepared as well following the same route, with and without the addition of the corresponding dopant.
2.2.2 Characterization techniques. The crystal phase of the materials was characterized by X-ray diffraction (XRD) and patterned as a collection in a 2θ range from 10° to 80° using a RIGAKU Ru-200B diffractometer equipped with Cu Kα irradiation at a scan rate of 0.5° s−1. The average crystallite size was determined from the broadening of the diffraction peak using the Scherrer formula (d = Kλ/β
cos
θ). The particles morphology and size distribution were examined by high resolution transmission electron microscopy (HRTEM, Tecnai G2 F20) and field-emission scanning electron microscopy (FE-SEM, Hitachi-S4800). The BET surface area was determined by a multipoint method on a Micromeritics ASAP 2020 using the adsorption data in the relative pressure range (P/P0) of 0.02–0.45. Prior to the analysis, the samples were degassed for 6 h at 150 °C under vacuum (10−5 mbar) in the degas port of the adsorption analyzer, and the specific surface area was calculated by the Brunauer–Emmett–Teller (BET) method. X-ray photoelectron spectroscopy (XPS) was obtained on a dual anode VG Microtech X-ray source and a Thermo Scientific ESCALAB 250Xi analyzer. A Cary 5000 UV-vis diffuse spectrophotometer was used to acquire the solid-state absorption and diffuse reflectance spectra (DRS) of the materials within the 200–800 nm range. The band gap values were evaluated using the Tauc Plot method by plotting (αhν)1/2 versus hν (photon energy, eV), where α is the absorption coefficient, considering that all the solids are indirect semiconductors, like TiO2.42 The photoluminescence emission spectra (PL, FLS-980) were tested to investigate the recombination of photogenerated electron–hole pairs. The samples were excited at 280 nm.
2.2.3. Photocatalytic degradation. The photocatalytic activity of the different photocatalysts were evaluated by monitoring the degradation of cationic MB and anionic RR4 dye, respectively. The experiments were carried out at room temperature in a photoreactor (XPA-7) with a 500 W xenon lamp as the irradiation source, which was surrounded by all the cylindrical containers and placed 10 cm away from these containers. 100 mg of all the prepared catalysts were suspended in 100 mL aqueous solutions of the organic dyes with the concentration of 25 mg L−1 in a beaker. Prior to irradiation, the system was placed in a total dark environment under magnetic stirring for 45 min to investigate the adsorption behaviors of the photocatalysts. Following this, the photocatalytic reaction was started by exposure to visible light. During the reaction process, vigorous agitation was performed in each container to guarantee the uniform irradiation of the photocatalysts. A certain solution of organic dye was taken out at regular intervals of 15 min and analyzed after centrifugation followed by filtration to remove the catalyst particles. The degradation efficiency of the pollutants was measured by detecting the absorbance at their maximum absorption wavelength (λmax) using the UV-vis spectrophotometer. The photometric analyses of all the photocatalysts could be carried out by measuring the degradation efficiency of dye (D%), which is defined by the following expression: |
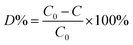 | (1) |
where C0 is the initial concentration of organic dye once the adsorption equilibrium was reached before lighting the lamp and C is the concentration of them after irradiation of the samples in the desired time interval. All the samples were tested under the same experimental conditions.
2.2.4. Stability tests of the photocatalysts. To evaluate the stability of the photocatalysts, a recycled usage experiment was carried out. Here, 100 mg of CSP-5 photocatalyst was suspended in 100 mL of a 25 mg L−1 solution of the dye and irradiated under a xenon lamp for 90 min. The sample was then centrifuged and the residues were collected, washed by distilled water and ethanol, then dried in the oven at 80 °C. Finally, this photocatalyst was reused again for the second cycle of degradation with a fresh dye solution. This process was repeated up to 5 times of application.
2.2.5. Photoelectrochemical experiments. To determine the photoelectrochemical characteristic of all the catalysts, experiments involving photocatalytic water splitting to generate hydrogen were carried out at ambient temperature and atmospheric pressure. Various photocatalyst powders were fabricated via the procedure reported by Ito et al.43 to produce electrodes. The experiment was processed in a quartz photoreactor with a three-electrode configuration using the P25 and the prepared photocatalysts as the working electrode, a Pt wire as the counter electrode, and Ag/AgCl as the reference electrode, which were immersed in the electrolyte containing 100 mL of a 20 vol% ethanol and distilled water mixed solution. Prior to irradiation, the suspension of the catalysts was dispersed by an ultrasonic clean for 10 min and then the system was evacuated for 30 min to ensure the reactor was in an anaerobic condition. A xenon lamp located above the reactor was used as the light source. The generated gas was collected by a probe in 30 min intervals and analyzed using gas chromatography (GC-14C) with N2 as the carrier flowing at 20 mL L−1. The photocatalytic activities of the different samples were evaluated by the H2 evolution rate in the same concentration range.
3. Results and discussion
3.1. XRD analysis
To investigate the crystal structure of the prepared catalysts, XRD measurements were performed. Fig. 1 shows the typical XRD patterns of the prepared samples. All the samples had similar patterns, which were dominated by a mixture of the anatase and rutile phases of TiO2. The diffraction peaks of all the samples at 2θ values of 25.3°, 37.8°, 48.0°, 53.9°, 55.1°, 62.7°, 68.9°, 70.3°, and 75.1° could be perfectly indexed to the (101), (004), (200), (105), (211), (204), (116), (220), and (215) crystal planes of anatase TiO2. Moreover, the patterns also clearly showed peaks of rutile TiO2, namely, the planes (110), (101), and (111) at 2θ values of ca. 27.4°, 36.1°, and 41.2° respectively. There was also no phase transformation for all the as-obtained samples. According to Zhang et al.,44 they observed the occurrence of phase transformation of TiO2 only when the temperature was at 900 °C. The particle sizes were calculated from the Scherrer equation for all the samples from the most intense diffraction peak (101) corresponding to the peak at 2θ 25.31°, and coincident with the information specified from the producers. The equation used was as follows: |
 | (2) |
where d is the average thickness of the crystal in a direction normal to the diffracting plane (hkl), β is the crystallite size contribution to the peak width at half maximum intensity in radians, and K is the Scherrer constant. The crystallite sizes were 35.4, 31.3, 26.4, 22.6, 20.3, and 19.8 nm for P25, SP, CSP-1, CSP-3, CSP-5, and CSP-7, respectively. P25 showed good crystallinity at all major 2θ positions for the anatase phase. A strong reduction in the diffraction line intensity of anatase could be seen in the SP and CPS samples. This does not reflect the degradation/transformation of the anatase phase but is rather a result of the X-ray diffractions of an additional guest phase of amorphous silica, thus reducing the TiO2 concentration in the mixture.45 On the other hand, it should be noted that the ionic radius of Ce4+ (0.101 nm) is much bigger than that of Ti4+ (0.068 nm), and therefore it is difficult for Ce4+ to replace Ti4+ in the crystal lattice. Also, no crystalline phase related to cerium oxides could be found, confirming that it is unlikely for Ce4+ to enter the TiO2 structure. In light of the ionic radii, as lanthanide ions are much larger than Ti4+, these cerium ions tended to bond to oxygen anion on the surface of titania. Therefore, the decrease in the crystal size might be attributed to the presence of Ce–O–Ti bonds on the surface of the doped samples, which inhibit the growth of crystal grains.46,47
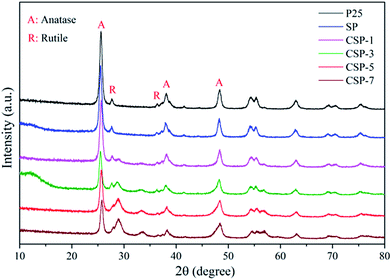 |
| Fig. 1 XRD patterns of all the catalysts. | |
3.2. N2 adsorption–desorption analysis
Gas physisorption is extensively used in the characterization of porous solids, particularly for evaluation of their specific surface area and total pore volume as well as their surface properties. The shape of the isotherm obtained from these adsorption measurements is very important as it can reveal the kind of porosity (micro-, meso-, or macroporosity) present in the sample. The differences in isotherms types and hysteresis loops can clearly be seen in Fig. 2. All the samples exhibit type IV sorption isotherms, while P25 shows an isotherm type II.48 Also, it can be clearly observed that all the modified samples led to a marked change in the shape of the hysteresis loop in comparison to P25, showing a partial collapse of the ordered pore arrangement into a disordered pore arrangement, which finds some supporting evidence also in the SEM, TEM, and XRD analyses. These samples exhibited sorption isotherms with a relatively narrow hysteresis loop of an H2 type, which is often attributed to porous inorganic oxides,49 which indicates that the powders had a mesoporous structure with a narrow slit-like shape,50 while P25 was reflected by the H3 hysteresis. The structural parameters determined on the basis of these isotherms are listed in Table 2. The results indicated that the P25 sample had the lowest surface area (84.67 m2 g−1), but with the introduction of Si and Ce in the sample, an increase in the surface area was observed. Among all the photocatalysts, the surface area of CSP-5 was found to be the highest at 226.50 m2 g−1. Also, the surface area of the CSP samples exceeded the sum of the SiO2/P25 and pristine P25 samples, hence this enhanced the dispersity of the active component on the surface of the catalysts, which is beneficial for photocatalytic activity. At a higher dopant concentration, the well-dispersed CeO2 species obstructed the pores and the active sites of TiO2, which thus decreased the specific surface area.
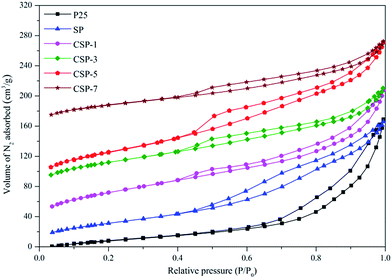 |
| Fig. 2 N2 adsorption–desorption isotherms of the P25, SP, and CSPs catalysts. | |
Table 2 Structural parameters of different catalysts
Samples |
Crystal size (nm) |
SBET (m2 g−1) |
Vtot (nm) |
P25 |
35.4 |
84.67 |
0.201 |
SP |
31.3 |
147.59 |
0.241 |
CSP-1 |
26.4 |
162.81 |
0.314 |
CSP-3 |
22.6 |
205.38 |
0.363 |
CSP-5 |
20.3 |
226.50 |
0.412 |
CSP-7 |
19.8 |
219.55 |
0.387 |
3.3. XPS analysis
X-ray photoelectron spectroscopic analysis was employed as an important characteristic method to detect the chemical states of the different species in the CSP samples. The XPS survey spectrum of the CSP-5 catalyst shown in Fig. 3a indicated that five elements of Ti, O, C, Si, and Ce existed on the surface of the CSP-5 sample. The carbon residue in the catalyst might be attributed to the carbon not being burned off completely during the heat treatment. The high resolution XPS spectrum with scanning over the area corresponding to the binding energies for the Ti 2p region is analyzed in Fig. 3b. The representative Ti 2p XPS spectrum indicates two peaks located at 458.9 eV and 464.5 eV, assigned to Ti 2p3/2 and Ti 2p1/2, which agree with the values of Ti4+ in a tetragonal structure.51,52 The O 1s spectra of the CSP-5 sample is illustrated in Fig. 3c. The spectrum shows two types of peaks for the oxygen in the sample. The dominant peaks located at 529.8 eV agree with the O 1s electron binding energy of the lattice oxygen for TiO2.53 And the fitting data at 533.1 eV should be assigned to a Si–O bond. The Si 2p core region is shown in Fig. 3d, which verifies the formation of an interfacial Ti–O–Si bond between TiO2 and SiO2. The Si 2p line of CSP-5 can be best fitted as two doublets. The main peak at the binding energy (BE) of 103.7 eV corresponds to a Si–O–Si bond,54 while the extra component that occurs at a 1.6 eV lower BE may be assigned to the Si–O–Ti bond. It turned out that a shift of electron density from the Ti to Si atom through an intermediate O atom led to a decrease in the BE of the Si atom, which confirmed the formation of an interfacial Si–O–Ti bond.22 The XPS spectrum for Ce 3d is revealed in Fig. 3e. The Ce 3d spectra can be split into four pairs of spin-orbital bands (3d5/2 and 3d3/2 denoted as v and u, respectively): v/u, v′/u′, v′′/u′′, and v′′′/u′′′, where the v/u, v′′/u′′, and v′′′/u′′′ peaks are associated with the characteristic chemical states of Ce4+, while v′/u′ corresponds to that of Ce3+.55 The Ce species present in both forms of Ce3+ and Ce4+, which shows that the surface of the catalyst was not fully oxidized, suggests the existence of oxygen vacancies. The Ce species are beneficial for the shift of the absorption toward the visible region and leads to a charge imbalance, thereby yielding oxygen vacancies and unsaturated chemical bonds. Thus, additional adsorbed oxygen species were generated on the surface of CSP-5, thus improving the photocatalytic degradation activity.56
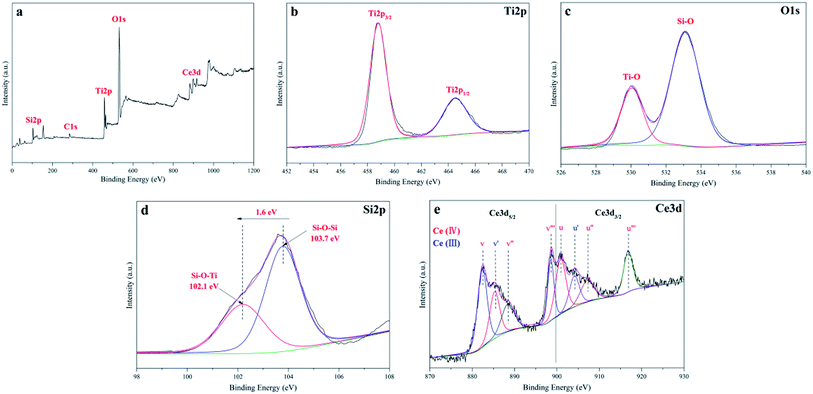 |
| Fig. 3 (a) XPS survey spectra; high resolution XPS spectra of the: (b) Ti 2p, (c) O 1s, (d) Si 2p, and (e) Ce 3d regions of the CSP-5 catalyst. | |
3.4. Morphological and structural properties
The morphologies of P25 and CSP-5 were examined by FE-SEM and HRTEM, with the corresponding images shown in Fig. 4 and 5. The representative FE-SEM images show that the pure P25 had smooth spherical particles (Fig. 4a), while the surface of CSPs was rougher and decorated with small SiO2 nanoparticles (Fig. 4b–e). The images of different concentrations of the Ce-doped samples are shown in Fig. 4b–e and indicate that the spherical aggregates of particles exhibited varying sizes, which was affected by the presence of silicon and cerium. In addition, Fig. 4 shows other FE-SEM images of P25 and CSP-5 together with the EDX spectrum of the Ti, O, Si, and Ce elements. From Fig. 4g, it can be seen that the elements of Ti, O, Si, and Ce were detected with random selection, confirming the presence of Si and Ce on the TiO2 nanoparticles. The EDX analysis results give the atomic percentages of Ti, O, Si, and Ce as 43.9%, 32.5%, 15.6%, and 5.1%, respectively. To further obtain the microscopic structural information, HRTEM analysis of different samples was carried out, and the results are displayed in Fig. 5. The particle size of P25 was about 37.2 nm and serious aggregation can be observed clearly (Fig. 5a). However, after Si and Ce doping it was found that the Ce–SiO2/TiO2 sample exhibited the morphology of a loose and homogeneous sphere and had a smaller particle size of 21.8 nm (Fig. 5b), which was in accordance with XRD results that the doped Ce could prevent TiO2 nanocrystals from aggregating. Clear lattice fringes of the P25 and CSP-5 samples in the magnified HRTEM images can be seen in Fig. 5c and d, in which the interplanar spacing of 0.362 nm and 0.325 nm correspond to the (101) lattice plane of anatase TiO2 and the (111) lattice plane of CeO2, respectively.
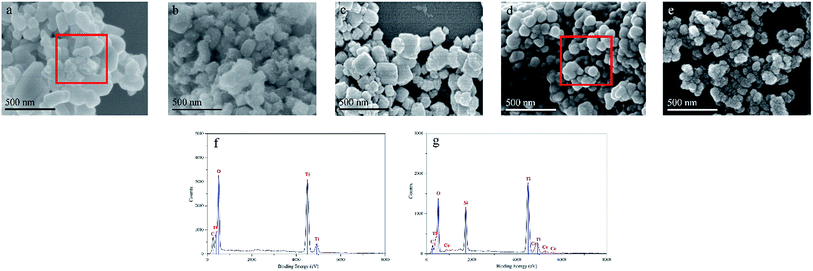 |
| Fig. 4 FE-SEM images of the: (a) P25, (b) CSP-1, (c) CSP-3, (d) CSP-5, and (e) CSP-7 catalysts; EDX of the (f) P25 and (g) CSP-5 catalysts. | |
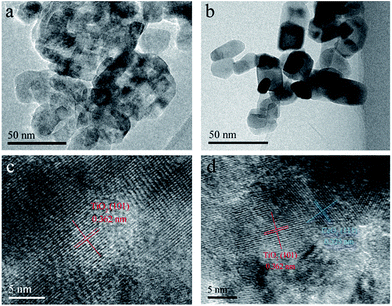 |
| Fig. 5 HRTEM images of (a) P25 and (b) CSP-5; magnified image of the (c) P25 and (d) CSP-5 catalysts. | |
3.5. Optical properties
3.5.1. UV-vis DRS. UV-vis DRS was performed and the resulting spectra are shown in Fig. 6, which indicate a decrease in the band gap energy of titania in the case of SiO2/P25 impregnation with Ce. From Fig. 6a, the onset of the absorption spectrum of P25 appears in the UV area, which matches well with the intrinsic band gap of TiO2. Also, it can be observed that the absorption edge is extended toward higher wavelength for the Ce-doped samples, and it increases with the increasing Ce doping concentration. Among all the samples, the largest red-shift can be found with the Ce-doped SiO2/P25 sample with 5 mM Ce. The Tauc plot from the Kubelka–Munk function was used to identify the band gap energy, as shown in Fig. 6b. The absorption coefficient α and the indirect band gap (Eg) are related through the following equation: |
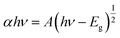 | (3) |
where α represents the absorption coefficient, h is Planck's constant, ν is the radiation frequency, and A is a constant. From the equation, it is possible to obtain a linear plot (αhν)1/2 versus hν, whose slope is the band gap value. Thus, the Eg of the as-prepared P25, SP, CSP-1, CSP-3, CSP-5, and CSP-7 samples were estimated to 3.02, 2.84, 2.31, 2.23, 2.01, and 2.16 eV respectively. A possible explanation for this phenomenon can be attributed to the presence of Ce3+, as observed from the XPS spectra. CeO2 has a band gap of about 2.92 eV, and the Ce 4f states are unoccupied. However, the existence of Ce3+ leads to an occupation of the 4f band, resulting in a new electronic state among the TiO2 band gap.57,58 The distance of charge transfer between the f electrons of the cerium and the conduction (CB) or valence band (VB) of TiO2 is thus narrowed, thereby allowing the generation of electron–hole pairs using visible light, in which the energy is lower than that required to excite the TiO2, thus yielding materials with lower band gap values.59 Hence, these Ce-doped SiO2/P25 composites show light absorption in the visible region and adequate band gap values to be used as visible-driven photocatalysts. The above information on the physical properties of the prepared catalysts is useful in the following study of photocatalysis.
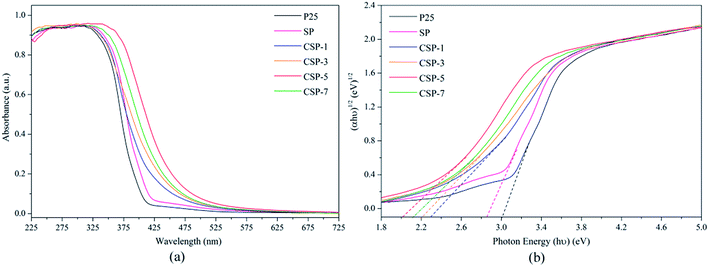 |
| Fig. 6 (a) UV-vis diffuse reflectance spectroscopy and (b) Tauc plot of the as-synthesized catalysts. | |
3.5.2. PL emission. The photoluminescence (PL) emission spectra of all the catalysts are shown in Fig. 7. It is well known that the PL spectra can be used to investigate the efficiency of charge transfer and electron–hole pairs' separation. PL emission mainly depends on the recombination of electron–hole pairs. A lower emission intensity reveals a decrease in the recombination rate, which is beneficial for improving the photocatalytic activity of catalysts. As can be seen in Fig. 7, the peak positions of the different catalysts were basically the same, showing that the individual loading of silicon and cerium has not induced new photoluminescence. Whereas, the peak intensity of Ce-doped SiO2/P25 were significantly weaker than that of pure P25, which indicated that the recombination of the photogenerated charge carriers were effectively inhibited. Further inspection of the PL spectra reveal that the CSP-5 sample has the lowest PL intensity compared to the other samples, which suggests that the recombination of photogenerated carriers was highly suppressed due to the cooperative effect of silicon and cerium. This decrease in intensity because of the decreased rate of electron–hole recombination facilitates the production of a large number of ·OH radicals, which enhances the photocatalytic activity for the degradation of pollutants.60 Nevertheless, it has been reported that excessive metal doping of TiO2 can adversely cause the acceleration of electron–hole recombination just as much as the CSP-7 material, thus overloading of a metal may not improve the photocatalytic activity.
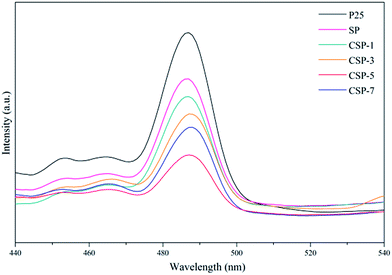 |
| Fig. 7 Photoluminescence emission spectra of all the catalysts. | |
3.6. Photocatalytic activity
3.6.1. Degradation of organic dyes. The nanocomposites prepared with and without cerium were tested as photocatalysts for the degradation of Methylene Blue (MB) and Reactive Red 4 (RR4) under visible-light irradiation. As indicated, before the photocatalytic reaction, the samples were mixed with the solutions under stirring in the dark for 45 min to reach the adsorption equilibrium. Fig. 8 shows the evolution of MB and RR4 concentration versus irradiation time with the P25 and SP/CSP series. Direct photolysis in the absence of any photocatalyst was very small under the experimental conditions and as can be seen, the photocatalytic activity depended on the cerium content of the samples. With the increase in the cerium percentages (for the CSP-1, CSP-3, and CSP-5 samples), there was an improvement in the photocatalytic activity compared with that of the nanocomposite without cerium (SP). However, a further increase in the Ce content resulted in a lower activity (CSP-7). This effect may be due to the structural changes caused by the incorporation of Ce into the TiO2 lattice. At low cerium concentrations, the structure of the anatase is not modified and the visible absorption becomes obvious, thus the enhanced photocatalytic activity seems to be ascribed to cerium. However, a further increase in the cerium percentage produced a modification of the anatase structure, which reduced the photocatalytic activity. According to Yan et al.,61 the photocatalytic activity was enhanced as a consequence of a lower crystal size at lower cerium amounts, which is in accordance with that observed in our work. Also, the presence of the cerium could effectively capture the photo-induced electrons and holes, while the Ce 4f levels improve the formation of electron–hole pairs, which can eventually enable the Ce4+/Ce3+ pairs to interact directly with organic molecules.62 However, a further increase in the Ce content can also produce recombination of the electron–hole pairs, thus reducing the photocatalytic activity.63,64 The corresponding results of the photodegradation rate by Ce-doped SiO2/P25 with different Ce contents and pure P25 under visible light are presented in Fig. 9. The CSP-5 sample shows the best photocatalytic activity of all the samples. The photodegradation rate of this sample could reach over 90% for both MB and RR4 (91.8% for MB and 90.2% for RR4, respectively) after reaction for 90 min, which was more efficient than that of undoped P25 and SP. In order to compare the catalytic activity easily, the results of the photocatalytic activities for different photocatalysts are shown in Table 3. From the table, it can be clearly seen that the photocatalytic activity is in the order of CSP-5 > CSP-7 > CSP-3 > CSP-1 > SP > P25. According to these results, it can be noted that doping with cerium and silicon can be considered as an effective way to improve the photoactivity for preparing TiO2-based photocatalysts. In addition, the photocatalytic mechanism will be demonstrated concretely in the following section.
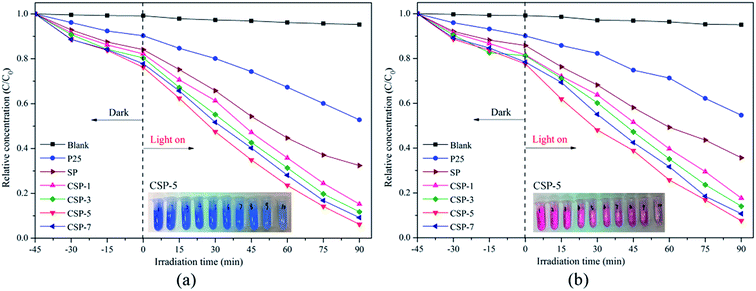 |
| Fig. 8 (a) Photodegradation of (a) MB and (b) RR4 versus irradiation time for all the photocatalysts. | |
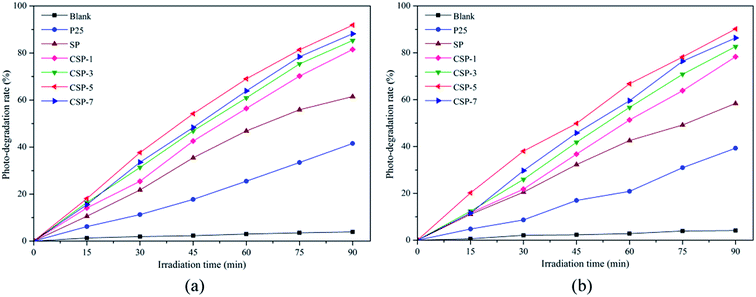 |
| Fig. 9 Degradation efficiency of (a) MB and (b) RR4 versus irradiation time for all the photocatalysts. | |
Table 3 Results of the photocatalytic activities for the different photocatalysts
Degradation of MB |
Degradation of RR4 |
Catalyst |
Relative concentration (C/C0) |
Degradation rate |
Catalyst |
Relative concentration (C/C0) |
Degradation rate |
Blank |
0.953 |
3.93% |
Blank |
0.951 |
4.13% |
P25 |
0.528 |
41.53% |
P25 |
0.547 |
39.29% |
SP |
0.324 |
61.47% |
SP |
0.357 |
58.39% |
CSP-1 |
0.152 |
81.51% |
CSP-1 |
0.177 |
78.31% |
CSP-3 |
0.118 |
85.32% |
CSP-3 |
0.141 |
82.66% |
CSP-5 |
0.062 |
91.86% |
CSP-5 |
0.087 |
88.79% |
CSP-7 |
0.092 |
88.17% |
CSP-7 |
0.107 |
86.35% |
The kinetics study of photocatalytic degradation for the different catalysts is discussed using the kinetic model proposed by Langmuir–Hinshelwood65,66 according to the equation:
|
 | (4) |
where
C0 is the initial concentration of organic dye once the adsorption equilibrium is reached before lighting the lamp,
C is the concentration of the dye after irradiation of the samples in the desired time interval,
k is a rate constant, and
t is the time. From
Fig. 10, a linear relationship between ln(
C/
C0) and the irradiation time could be observed, which suggested that the degradation of MB and RR4 followed first-order reaction kinetics. The kinetics of CSP-5 was almost five times faster than that of the P25 sample, which coincided nicely with higher surface area of the former sample. In accordance with this was the kinetics of the SP sample, which was also faster than the pure TiO
2 sources (
Table 4).
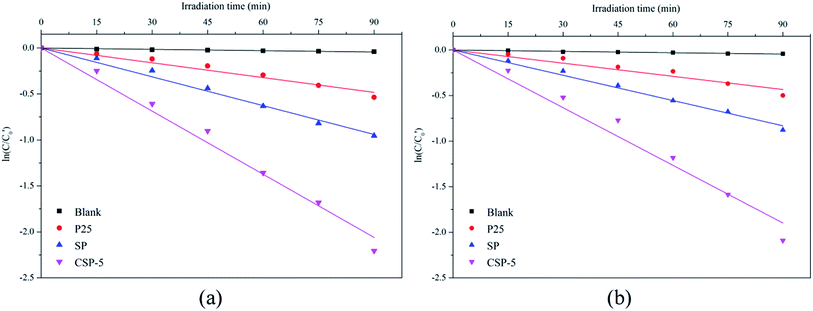 |
| Fig. 10 First-order kinetics for (a) MB and (b) RR4 photocatalytic degradation with various photocatalysts under visible-light irradiation. | |
Table 4 Kinetic features of the catalysts for the degradation of Methylene Blue and Reactive Red 4
Organic pollutants |
Catalysts |
Rate constant × 10−3 (min−1) |
R2 |
Methylene Blue |
Blank |
0.49 |
0.982 |
P25 |
5.36 |
0.985 |
SP |
10.45 |
0.995 |
CSP-5 |
22.88 |
0.994 |
Reactive Red 4 |
Blank |
0.51 |
0.987 |
P25 |
4.81 |
0.972 |
SP |
9.24 |
0.996 |
CSP-5 |
21.08 |
0.988 |
Generally, pH is not just an important factor influencing the degradation efficiency of organic compounds in the photocatalytic process but is also a vital variable in the actual treatment of wastewater. Hence, extra experiments were carried out to determine the effect of pH on the degradation of MB and RR4 at pH values in the range of 3–11. The strong influence of pH on MB and RR4 photodegradation are shown in Fig. 11. It can be observed from Fig. 11a that the photodegradation rate of MB increased with increasing pH. According to the previous research, the isoelectric point of SiO2/TiO2 is 3.00 pH units, resulting in the surface of the catalyst having a negative potential in the range of pH > 3.38 Since MB is a cationic dye, it is easier to adsorb onto the surface of the catalysts to promote reaction at higher pH. Another possible reason for the result is that there are more hydroxide ions (OH−) in the alkaline condition that can react with the photogenerated holes to favor the formation of more hydroxyl radicals (·OH), which could significantly enhance the photocatalytic degradation of the dye.67 On the contrary, it is well known that RR4 is an anionic dye. Based on the theory we mentioned above, the opposite results presented in the anionic RR4 experiment were as expected in Fig. 11b, where it can be seen that the photocatalytic performance of RR4 dropped when going from pH 3 to 11. Therefore, it can be concluded that the influence of pH on photocatalysis is mainly due to the amount of dye adsorbed on the catalysts, and that the degradation takes place at or near the catalyst surface, rather than in the bulk solution.
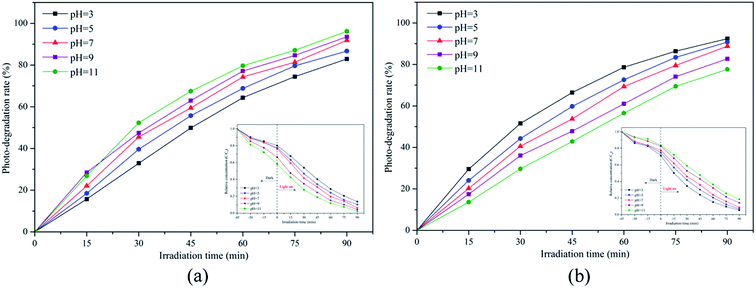 |
| Fig. 11 Effects of the initial pH on the photodegradation of (a) MB and (b) RR4 by the CSP-5 catalyst. | |
3.6.2. Stability of the photocatalysts. One of the most important practical aspects of photocatalysis is the recovery of the used nanocatalyst from suspension and its reuse several times in repeated photocatalytic cycles.68 The recovery of the nanocatalyst (such as TiO2) from aqueous suspension becomes easy when such small particles are supported on relatively large size support particles (such as SiO2).Fig. 12 shows the results of the recycling experiments where the same photocatalyst powder was repeatedly used for up to five times for the photodegradation of MB and RR4. The P25 and CSP-5 catalysts from the first cycle were recovered by centrifugation and reused for the second cycle, and so on. As shown in Fig. 12, the degradation efficiency for both MB and RR4 decreased gradually for both samples after several recycles because active sites on the surface of the samples may be covered by organic substances. Also, some small particles of the catalyst are lost after washing and filtration in each cycle. However, it is clear that the recycled CSPs do not lose their photocatalytic activity upon reuse for up to five times (91.3–87.6% for MB, 88.6–83.9% for RR4) in comparison to pure P25, whereas this difference was higher (41.5–26.9% for MB, 39.3–21.9% for RR4) in the P25 sample. Undoubtedly, we can say that the Ce–SiO2/P25 sample was a robust and stable catalyst. The CSPs thus offers the advantages of easy recovery by centrifugation and good recyclability due to persistence of the photoactivity of the recycled photocatalyst.
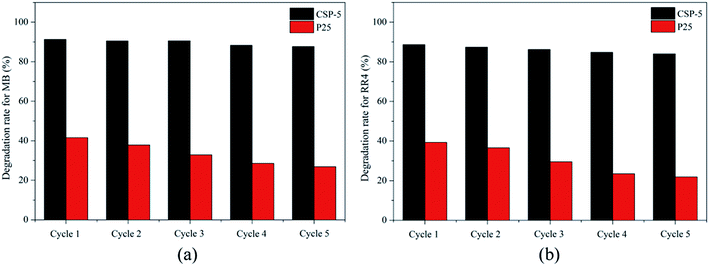 |
| Fig. 12 Photodegradation of (a) MB and (b) RR4 by the recycled P25 and CSP-5 photocatalysts during repeated photocatalytic cycles. | |
3.6.3. Photoelectrochemical experiments. Since the as-prepared photocatalysts showed the perfect performance in the degradation of the tested dyes, hydrogen evolution experiments were further carried out to investigate the accurate photoelectrochemical properties of the as-prepared samples. Before the actual photoelectrochemical experiments were started, pure ethanol solution was used for the control experiment in the absence of either photocatalyst or irradiation. There was no hydrogen production observed in this experiment. The results therefore excluded other influences of hydrogen generation except from the photocatalytic activities of the as-prepared catalysts. Fig. 13 shows the H2 evolution of all the catalysts as a function of irradiation time. As the time went on, the generation of hydrogen of all the samples ascended steadily. P25 presented the lowest photocatalytic efficiency in water splitting among all the catalysts, which was only 0.287 mmol g−1 after 5 h under visible-light irradiation. Besides, SP performed much better than pure P25, which was 1.294 mmol g−1. As expected, the H2 evolution rate of the CSPs samples were up to 1.573, 2.019, 2.216, and 2.315 mmol g−1, respectively, higher than SP. This was due to the synergistic effects of silicon and cerium, which could significantly improve the photocatalytic activity of TiO2. The redox coupling of Ce4+/Ce3+ was able to form labile oxygen vacancies with a relatively high mobility of bulk oxygen species, so Ce ions were able to effectively trap conductive band electrons, leading to a high photocatalytic activity. Moreover, Si4+ with a higher electronegativity could replace the Ti4+ in the TiO2–SiO2 composite system, leading to the formation of Si–O–Ti bonds. With the formation of Si–O–Ti bonds, more surface defects may appear. These defects can not only capture the photogenerated electrons or holes but can also increase the reaction activity of hydroxyls, both of which increase the photocatalytic activity of TiO2.69,70 It was found that CSP-5 sample had the best photoactivity under the same conditions. However, cerium adversely worked as photogenerated electrons recombination centers when overloaded, as we mentioned in the section covering the photoluminescence emission spectrum. Therefore, it may be suggested that photogenerated electrons recombination should be considered when increasing the visible-light absorption of TiO2.
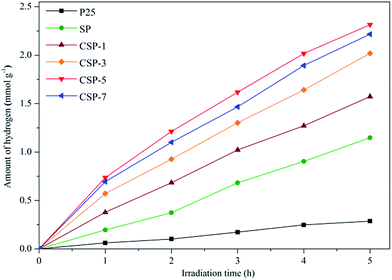 |
| Fig. 13 Visible-light-driven photocatalytic H2 evolution over all the photocatalysts. | |
3.7. Photocatalytic mechanism
The plausible photocatalytic mechanism is proposed in this section. When the photocatalyst is irradiated by visible light, electron–hole pairs could be generated, which then react with water and dioxygen absorbed on the photocatalyst surface, leading to more oxidized species to form hydroxyl radicals (·OH) and superoxide radical anions (O2−). These oxy radicals can attack organic compounds, including MB and RR4, and decompose them into CO2 and H2O. The specific mechanism of photocatalysis mainly includes the following reactions: |
·OH(O2−) + MB/RR4 → CO2 + H2O
| (10) |
However, from the results of the experiments presented in the preceding sections, it turns out that there are many factors that contribute to the enhanced photocatalytic activity of CSPs compared to pure P25, including the following.
3.7.1. Better dispersion of and improved adsorption of organic pollutants. An important difference between the P25 and CSPs samples may be the degree of dispersion of the nanoparticles in suspension. Better dispersion is expected in the case of silica-supported titania,71,72 while pure P25 very easily forms aggregates in aqueous dispersions.73 For the supported nanoparticles attached to the silica surface, aggregation is hindered, giving higher accessibility to adsorbates (MB and RR4 in this case). These materials allow an improved probability of photocatalytic reaction, which facilitates the attack by either free or trapped holes.74–76
3.7.2. Structural effects. As shown in Fig. 14, the incorporation of Ce dopants can result in a red-shift of the optical absorption edge, indicating that some energy levels are formed on the top of the valence band.77 From Fig. 14, when the system is irradiated under visible light (hν < 3.2 eV), the electrons are more excited from the Ce-doped energy level to arrive at the conduction band of TiO2. Owing to the enhancement of the visible-light adsorption, the visible-light-induced photocatalytic performance of Ce-doped P25 would also be improved compared to pure P25. As for the Ce3+/Ce4+ pairs, they can serve as electron acceptors and superoxide radicals (·O2−) producers by altering the electron–hole pairs recombination rate.78 Photogenerated electrons could be trapped by Ce4+, and the Ce4+ could reduce to Ce3+. Then, the Ce3+ could also be oxidized back to Ce4+ by the adsorbed oxygen in this system. Meanwhile, the chemisorbed oxygen could trap the electron of Ce3+, and then produce ·O2−,79 which can effectively oxidate organic pollutants into CO2 and H2O. Ce3+/Ce4+ electronic pairs can also capture protons, generating peroxide radicals, or can be further oxidized yielding hydroxide radicals (·OH), whose presence improves the efficiency of the photocatalyst by reacting with the organic pollutants.80 Therefore, the presence of the Ce3+/Ce4+ pairs can not only efficiently separate the electrons and holes but can also result in the generation of ·O2−, thus enhancing the occurrence of ·OH for the degradation of the organic molecules. However, excess Ce species might cover the active sites or act as a recombination center of TiO2, which can result in the decrease the photocatalytic activity and in the separation efficiency of the charge carriers, as discussed in Section 3.5.2.
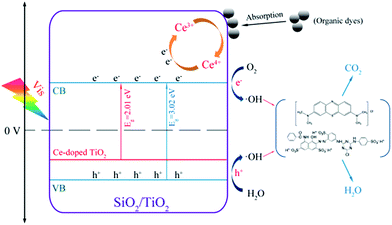 |
| Fig. 14 Photocatalytic mechanism for the photodegradation of organic dyes over Ce–SiO2/P25 catalyst under visible light. | |
In summary, the enhanced photoactivity of CSPs compared to pristine P25 may thus be attributed to a combined effect involving a better dispersion of the supported TiO2, a good adsorption of organic molecules, and the formation of new energy levels on the CSPs.
4. Conclusions
Low-cost Ce–SiO2/P25 nanoparticles (denoted as CSPs in this article) were prepared using an environmentally friendly approach involving precipitation of a colloidal solution of Degussa P25 nanoparticles with TEOS and peptization with Ce(NO3)3, at low temperature and at ambient pressure. The formed CSPs had a high BET surface area, small crystallite size, and large pore volume, which was beneficial for enhancing the adsorption of organic pollutants on the catalyst surface before their degradation. The CSPs were evaluated as a kind of Ce–SiO2/TiO2 composite in the form of powders for the total decomposition of Methylene Blue (MB) and Reactive Red 4 (RR4) as model organic pollutants. In particular, the CSP-5 nanoparticle was the most effective photocatalyst for the degradation of organic dyes under visible-light irradiation, and showed 91.8% decomposition of MB and 90.2% decomposition of RR4 in the liquid phase, at room temperature. The results indicated that the increase in photocatalytic activity of the CSP-5 sample resulted from the combination of a quantum size effect with the reduced size of the nanocrystals, new energy levels, and enhanced adsorption of the pollutants due to the SiO2 support. Another operative condition was studied for further practical application. The optimal solution pH for RR4 and MB was found to be equal to 3 and 11, respectively. Since real wastewater is a mixture of many pollutants, it is important to design a photocatalyst that addresses as many of them in the highest decomposition efficiency possible. The two contaminants used in this study (MB and RR4) are considered as surrogates for two major classes (cationic and anionic dyes) of water contaminants, which represent a good choice for testing the efficiency of newly synthesized photocatalysts. We expect our work could give a new way of thinking about the exploration of TiO2 photocatalysts.
Acknowledgements
The author would like to thank Beijing Jiaotong University and the State Key Laboratory of Solid Wastes Resource Utilization and Energy Saving Building Materials in Beijing Building Materials Academy of Science Research. This work was financially supported by Beijing Jiaotong University Foundation (2012RC002), the National Science Foundation of China (Grant no. 11475017), the State Key Laboratory of Solid Wastes Resource Utilization and Energy Saving Building Materials (SWR-2013-009) and the Fundamental Research Funds for the Central Universities (2014JBZ010). Yan Jiang would also like to express his gratitude to all colleagues who provided assistance.
References
- K. Kümmerer, in Pharmaceuticals in the Environment: Sources, Fate, Effects and Risk, ed. K. Kümmerer, Springer, New York, 2004, pp. 3–11 Search PubMed.
- D. Maycock and C. Watts, in Encyclopedia of Environmental Health, ed. J. Nriagu, Elsevier, Burlington, 2011, pp. 472–484 Search PubMed.
- A. Fujishima and K. Honda, Electrochemical photocatalysis of water at semiconductor electrode, Nature, 1972, 238, 37–38 CrossRef CAS PubMed.
- X. Chen and A. Selloni, Introduction: titanium dioxide (TiO2) nanomaterials, Chem. Rev., 2014, 114, 9281–9282 CrossRef CAS PubMed.
- A. Linsebigler, G. Lu and J. Yates, Photocatalysis on TiO2 surfaces: principles, mechanisms, and selected results, Chem. Rev., 1995, 95, 735–758 CrossRef CAS.
- A. Fujishima, X. Zhang and D. Tryk, TiO2 photocatalysis and related surface phenomena, Surf. Sci. Rep., 2008, 63, 515–582 CrossRef CAS.
- E. Pakdel and W. Daoud, Self-cleaning cotton functionalized with TiO2/SiO2: focus on the role of silica, J. Colloid Interface Sci., 2013, 401, 1–7 CrossRef CAS PubMed.
- S. Son, S. Hwang, C. Kim, J. Yun and J. Jang, Designed synthesis of SiO2/TiO2 core/shell structure as light scattering material for highly efficient dye-sensitized solar cells, ACS Appl. Mater. Interfaces, 2013, 5, 4815–4820 CAS.
- Y. Li, P. Leung, L. Yao, Q. Song and E. Newton, Antimicrobial effect of surgical masks coated with nanoparticles, J. Hosp. Infect., 2006, 62, 58–63 CrossRef CAS PubMed.
- D. Sheel and P. Evans, Photoactive and antibacterial TiO2 thin films on stainless steel, Surf. Coat. Technol., 2007, 201, 9319–9324 CrossRef.
- K. Saravanan, K. Ananthanarayanan and P. Balaya, Mesoporous TiO2 with high packing density for superior lithium storage, Energy Environ. Sci., 2010, 3, 939–948 CAS.
- J. Schneider, M. Matsuoka, M. Takeuchi, J. Zhang, Y. Horiuchi, M. Anpo and D. W. Bahnemann, Understanding TiO2 photocatalysis: mechanisms and materials, Chem. Rev., 2014, 114, 9919–9986 CrossRef CAS PubMed.
- M. Dahl, Y. Liu and Y. Yin, Composite titanium dioxide nanomaterials, Chem. Rev., 2014, 114, 9853–9889 CrossRef CAS PubMed.
- Y. Wang, W. Duan, B. Liu, X. Chen, F. Yang and J. Guo, The effect of doping copper and mesoporous structure on photocatalytic properties of TiO2, J. Nanomater., 2014, 178152, 7 Search PubMed.
- N. Mandzy, E. Grulke and T. Druffel, Breakage of TiO2 agglomerates in electrostatically stabilized aqueous dispersions, Powder Technol., 2005, 160, 121–126 CrossRef CAS.
- D. Hanaor, M. Assadi, S. Li, A. Yu and C. Sorrell, Ab initio study of phase stability in doped TiO2, Comput. Mech., 2012, 50, 185–194 CrossRef.
- K. Raj and B. Viswanathan, Effect of surface area, pore volume and particle size of P25 titania on the phase transformation of anatase to rutile, Indian J. Chem., 2009, 48, 1378–1382 Search PubMed.
- J. Herrmann, Heterogeneous photocatalysis: fundamentals and applications to the removal of various types of aqueous pollutants, Catal. Today, 1999, 53, 115–129 CrossRef CAS.
- A. Hanprasopwattana, T. Rieker, A. Sault and A. Datye, Morphology of titania coatings on silica gel, Catal. Lett., 1997, 45, 165–175 CrossRef CAS.
- R. Iler, The chemistry of Silica, Wiley-Interscience, New York, 1978 Search PubMed.
- A. Fink, W. Stöber and E. Bohn, Controlled growth of monodisperse silica spheres in the micron size range, J. Colloid Interface Sci., 1968, 26, 62–69 CrossRef.
- S. Ullah, E. Ferreira-Neto, A. Pasa, C. Alcântara, J. Acuna, S. Bilmes, M. Ricci, R. Landers, T. Fermino and U. Rodrigues-Filho, Enhanced photocatalytic properties of core@shell SiO2@TiO2 nanoparticles, Appl. Catal., B, 2015, 179, 333–343 CrossRef CAS.
- G. Tian, H. Fu, L. Jing, B. Xin and K. Pan, Preparation and characterization of stable biphase TiO2 photocatalyst with high crystallinity, large surface area, and enhanced photoactivity, J. Phys. Chem. C, 2008, 112, 3083–3089 CAS.
- H. Zhu, Z. Ma, S. Overbury and S. Dai, Rational design of gold catalysts with enhanced thermal stability: post modification of Au/TiO2 by amorphous SiO2 decoration, Catal. Lett., 2007, 116, 128–135 CrossRef CAS.
- P. Periyat, K. Baiju, P. Mukundan, P. Pillai and K. Warrier, High temperature stable mesoporous anatase TiO2 photocatalyst achieved by silica addition, Appl. Catal., A, 2008, 349, 13–19 CrossRef CAS.
- S. Rasalingam, R. Peng and R. Koodali, Removal of hazardous pollutants from wastewaters: applications of TiO2–SiO2 mixed oxide materials, J. Nanomater., 2014, 617405, 42 Search PubMed.
- C. Anderson and A. Bard, An improved photocatalyst of TiO2/SiO2 prepared by a sol–gel synthesis, J. Phys. Chem., 1995, 99, 9882–9885 CrossRef CAS.
- P. Cheng, M. Zheng, Y. Jin, Q. Huang and M. Gu, Preparation and characterization of silica-doped titania photocatalyst through sol–gel method, Mater. Lett., 2003, 57, 2989–2994 CrossRef CAS.
- G. Calleja, D. Serrano, R. Sanz and P. Pizarro, Mesostructured SiO2-doped TiO2 with enhanced thermal stability prepared by a soft-templating sol–gel route, Microporous Mesoporous Mater., 2008, 111, 429–440 CrossRef CAS.
- J. Aguado, R. Grieken, M. López-Muñoz and J. Marugán, Comprehensive study of the synthesis, characterization and activity of TiO2 and mixed TiO2/SiO2, Appl. Catal., A, 2006, 312, 202–212 CrossRef CAS.
- Y. Cong, B. Tian and J. Zhang, Improving the thermal stability and photocatalytic activity of nanosized titanium dioxide via La3+ and N co-doping, Appl. Catal., B, 2011, 101, 376–381 CrossRef CAS.
- S. Sajjad, S. Leghari, F. Chen and J. Zhang, Bismuth-doped ordered mesoporous TiO2: visible-light catalyst for simultaneous degradation of phenol and chromium, Chem.–Eur. J., 2010, 16, 13795–13804 CrossRef CAS PubMed.
- W. Wang, J. Zhang, F. Chen, M. Anpo and D. He, Catalysis of redox reactions by Ag@TiO2 and Fe3+-doped Ag@TiO2 core–shell type nanoparticles, Res. Chem. Intermed., 2010, 36, 163–172 CrossRef CAS.
- X. Yuan, J. Zhang, M. Anpo and D. He, Synthesis of Fe3+ doped ordered mesoporous TiO2 with enhanced visible light photocatalytic activity and highly crystallized anatase wall, Res. Chem. Intermed., 2010, 36, 83–88 CrossRef CAS.
- M. Nasir, F. Zhang and B. Tian, Detailed study of Ce and C codoping on the visible light response of titanium dioxide, Res. Chem. Intermed., 2015, 41, 1607–1624 CrossRef CAS.
- M. Nasir, S. Bagwasi, Y. Jiao, F. Chen, B. Tian and J. Zhang, Characterization and activity of the Ce and N co-doped TiO2 prepared through hydrothermal method, Chem. Eng. J., 2014, 236, 388–397 CrossRef CAS.
- V. Stengl, S. Bakardjieva and N. Murafa, Preparation and photocatalytic activity of rare earth doped TiO2 nanoparticles, Mater. Chem. Phys., 2009, 114, 217–226 CrossRef CAS.
- Y. Liu, H. Yu, Z. Lv, S. Zhan, J. Yang, X. Peng, Y. Ren and X. Wu, Simulated-sunlight-activated photocatalysis of Methylene Blue using cerium-doped SiO2/TiO2 nanostructured fibers, J. Environ. Sci., 2012, 24, 1867–1875 CrossRef CAS.
- S. Pavasupree, Y. Suzuki, S. Pivsa-Art and S. Yoshikawa, Preparation and characterization of mesoporous TiO2–CeO2 nanopowders respond to visible wavelength, J. Solid State Chem., 2005, 178, 128–134 CrossRef CAS.
- B. Reddy, A. Khan, Y. Yamada, T. Kobayashi, S. Loridant and J. C. Volta, Surface characterization of CeO2/SiO2 and V2O5/CeO2/SiO2 catalysts by Raman, XPS, and other techniques, J. Phys. Chem. B, 2002, 106, 10964–10972 CrossRef CAS.
- F. Li, X. Li, M. Hou, K. Cheah and W. Choy, Enhanced photocatalytic activity of Ce3+–TiO2 for 2-mercaptobenzothiazole degradation in aqueous suspension for odour control, Appl. Catal., A, 2005, 285, 181–189 CrossRef CAS.
- M. Fernández-García, A. Martínez-Arias, J. Hanson and J. Rodríguez, Nanostructured oxides in chemistry: characterization and properties, Chem. Rev., 2004, 104, 4063–4104 CrossRef PubMed.
- S. Ito, P. Chen, P. Comte, M. Nazeeruddin, P. Liska and P. Pechy, Fabrication of screen printing pastes from TiO2 powders for dye-sensitised solar cells, Prog. Photovoltaics, 2007, 15, 603–612 CAS.
- J. Zhang, Z. Huang, Y. Xu and F. Kang, Carbon-coated TiO2 composites for the photocatalytic degradation of low concentration benzene, New Carbon Materials, 2011, 26, 63–70 CrossRef CAS.
- A. Suligoj, U. Stangara, A. Ristic, M. Mazaj, D. Verhovsek and N. Tusar, TiO2–SiO2 films from organic-free colloidal TiO2 anatase nanoparticles as photocatalyst for removal of volatile organic compounds from indoor air, Appl. Catal., A, 2016, 184, 119–131 CrossRef CAS.
- A. Xu, Y. Gao and H. Liu, The preparation, characterization, and their photocatalytic activities of rare-earth-doped TiO2 nanoparticles, J. Catal., 2002, 207, 151–157 CrossRef CAS.
- J. Xu, Y. Ao and D. Fu, A novel Ce,C-codoped TiO2 nanoparticles and its photocatalytic activity under visible light, Appl. Surf. Sci., 2009, 256, 884–888 CrossRef CAS.
- K. Sing, Reporting physisorption data for gas/solid systems with special reference to the determination of surface area and porosity, Pure Appl. Chem., 1982, 54, 2208–2218 CrossRef.
- M. Kruk and M. Jaroniec, Gas adsorption characterization of ordered organic–inorganic nanocomposite materials, Chem. Mater., 2001, 13, 3169–3183 CrossRef CAS.
- J. Yu, J. Yu, M. Leung, W. Ho, B. Cheng, X. Zhao and J. Zhao, Effects of acidic and basic hydrolysis catalysts on the photocatalytic activity and microstructures of bimodal mesoporous titania, J. Catal., 2003, 217, 69–78 CAS.
- Y. Cao, W. Yang, W. Zhang, G. Liu and P. Yue, Improved photocatalytic activity of Sn4+ doped TiO2 nanoparticulate films prepared by plasma-enhanced chemical vapor deposition, New J. Chem., 2004, 28, 218–222 RSC.
- B. Erdem, R. Hunsicker, G. Simmons, E. Sudol, V. Dimonie and M. El-Aasser, XPS and FTIR surface characterization of TiO2 particles used in polymer encapsulation, Langmuir, 2001, 17, 2664–2669 CrossRef CAS.
- M. Nasir, Z. Xi, M. Xing, J. Zhang, F. Chen, B. Tian and S. Bagwasi, Study of synergistic effect of Ce- and S-codoping on the enhancement of visible-light photocatalytic activity of TiO2, J. Phys. Chem. C, 2013, 117, 9520–9528 CAS.
- T. Gross, M. Ramm, H. Sonntag, W. Unger, H. Weijers and E. Adem, An XPS analysis of different SiO2 modifications employing a C1s as well as an Au4f7/2 static charge reference, Surf. Interface Anal., 1992, 18, 59–64 CrossRef CAS.
- E. Paparazzo, G. Ingo and N. Zacchetti, X-ray induced reduction effects at CeO2 surfaces:
an X-ray photoelectron spectroscopy study, J. Vac. Sci. Technol., A, 1991, 9, 1416–1420 CAS.
- S. Yang, W. Zhu, J. Wang and Z. Chen, The surface properties and the activities in catalytic wet air oxidation over CeO2–TiO2 catalysts, J. Hazard. Mater., 2008, 153, 1248–1253 CrossRef CAS PubMed.
- N. Skorodumova, R. Ahuja, S. Simak, I. Abrikosov, B. Johansson and B. Lundqvist, Electronic, bonding, and optical properties of CeO2 and Ce2O3 from first principles, Phys. Rev. B: Condens. Matter Mater. Phys., 2001, 64, 115108 CrossRef.
- D. Andersson, S. Simak, B. Johansson, I. Abrikosov and N. Skorodumova, Modeling of CeO2, Ce2O3, and CeO2−x in the LDA+U formalism, Phys. Rev. B: Condens. Matter Mater. Phys., 2007, 75, 035109 CrossRef.
- C. Belver, R. Bellod, S. Stewart, F. Requejo and M. Fernandez-Garcia, Nitrogen-containing TiO2 photocatalysts: part 2. Photocatalytic behavior under sunlight excitation, Appl. Catal., B, 2006, 65, 309–314 CrossRef CAS.
- Z. Jin, W. Duan, B. Liu, X. Chen, F. Yang and J. Guo, Fabrication of efficient visible light activated Cu–P25–graphene ternary composite for photocatalytic degradation of methyl blue, Appl. Surf. Sci., 2015, 356, 707–718 CrossRef CAS.
- N. Yan, Z. Zhu, J. Zhang, Z. Zhao and Q. Liu, Preparation and properties of Ce-doped TiO2 photocatalyst, Mater. Res. Bull., 2012, 47, 1869–1873 CrossRef CAS.
- Y. Xie and C. Yuan, Visible-light responsive cerium ion modified titania sol and nanocrystallites for X-3B dye photodegradation, Appl. Catal., B, 2003, 46, 251–259 CrossRef CAS.
- K. Nagaveni, M. Hegde and G. Madras, Structure and photocatalytic activity of Ti1−xMxO2 ± delta (M = W, V, Ce, Zr, Fe, and Cu) synthesized by solution combustion method, J. Phys. Chem. B, 2004, 108, 20204–20212 CrossRef CAS.
- S. Ikeda, N. Sugiyama, B. Pal, G. Marc, L. Palmisano, H. Noguchi, K. Uosakid and B. Ohtani, Photocatalytic activity of transition-metal-loaded titanium(IV) oxide powders suspended in aqueous solutions: correlation with electron hole recombination kinetics, Phys. Chem. Chem. Phys., 2001, 3, 267–273 RSC.
- Y. Chiang and C. Lin, Photocatalytic decolorization of methylene blue in aqueous solutions using coupled ZnO/SnO2 photocatalysts, Powder Technol., 2013, 246, 137–143 CrossRef CAS.
- S. Kansal, G. Kaur and S. Singh, Studies on the photocatalytic degradation of 2,3-dichlorophenol using different oxidants in aqueous solutions, React. Kinet. Catal. Lett., 2009, 98, 177–186 CrossRef CAS.
- C. Wang, C. Lee, M. Lyu and L. Juang, Photocatalytic degradation of C.I. basic violet 10 using TiO2 catalysts supported by Y zeolite: an investigation of the effects of operational parameters, Dyes Pigm., 2008, 76, 817–824 CrossRef CAS.
- X. Li, X. Wu, G. He, J. Sun, W. Xiao and Y. Tan, Microspheroidization treatment of macroporous TiO2 to enhance its recycling and prevent membrane fouling of photocatalysis-membrane system, Chem. Eng. J., 2014, 251, 58–68 CrossRef CAS.
- X. Gao and I. Wachs, Titania–silica as catalysts: molecular structural characteristics and physico-chemical properties, Catal. Today, 1999, 51, 233–254 CrossRef CAS.
- Y. Yao, N. Zhao, J. Feng, M. Yao and F. Li, Photocatalytic activities of Ce or Co doped nanocrystalline TiO2–SiO2 composite films, Ceram. Int., 2013, 39, 4735–4738 CrossRef CAS.
- A. Li, Y. Jin, D. Muggli, D. Pierce, H. Aranwela, G. Marasinghe, T. Knutson, G. Brockman and X. Zhao, Nanoscale effects of silica particle supports on the formation and properties of TiO2 nanocatalysts, Nanoscale, 2013, 5, 5854–5862 RSC.
- M. Hirano, K. Ota and H. Iwata, Formation of anatase (TiO2)/silica (SiO2) composite nanoparticles with high phase stability of 1300 °C from acidic solution by hydrolysis under hydrothermal condition, Chem. Mater., 2004, 16, 3725–3732 CrossRef CAS.
- R. Pagel and J. Dohtmann, Thermochemical study of photoinduced processes at TiO2 nanoparticles in aqueous suspension containing Br− or Cl−. Photodeaggregation and subsequent processes, J. Phys. Chem. C, 2007, 111, 4458–4464 CAS.
- C. Minero, F. Catozzo and E. Pelizzetti, Role of adsorption in photocatalyzed reactions of organic molecules in aqueous titania suspensions, Langmuir, 1992, 8, 481–486 CrossRef CAS.
- D. Friesen, L. Morello, J. Headley and C. Langford, Factors influencing relative efficiency in photo-oxidations of organic molecules by Cs3PW12O40 and TiO2 colloidal photocatalysts, J. Photochem. Photobiol., A, 2000, 133, 213–220 CrossRef CAS.
- Y. Xu and C. Langford, UV- or visible-light-induced degradation of X3B on TiO2 nanoparticles: the influence of adsorption, Langmuir, 2001, 17, 897–902 CrossRef CAS.
- S. Song, L. Xu, Z. He, J. Chen, X. Xiao and B. Yan, Mechanism of the photocatalytic degradation of CI Reactive Black 5 at pH 12.0 using SrTiO3/CeO2 as the catalyst, Environ. Sci. Technol., 2007, 41, 5846–5853 CrossRef CAS PubMed.
- Q. Chen, D. Jiang, W. Shi, D. Wu and Y. Xu, Visible-light-activated Ce–Si co-doped TiO2 photocatalyst, Appl. Surf. Sci., 2009, 255, 7918–7924 CrossRef CAS.
- E. Kozlova, T. Korobkina, A. Vorontsov and V. Parmon, Enhancement of the O2- or H2-photoproduction rate in a Ce3+/Ce4+–TiO2 system by the TiO2 surface and structure modification, Appl. Catal., A, 2009, 367, 130–137 CrossRef CAS.
- N. Yan, Z. Zhu, J. Zhang, Z. Zhao and Q. Liu, Preparation and properties of Ce-doped TiO2 photocatalyst, Mater. Res. Bull., 2012, 47, 1869–1873 CrossRef CAS.
|
This journal is © The Royal Society of Chemistry 2017 |
Click here to see how this site uses Cookies. View our privacy policy here.