DOI:
10.1039/C6RA28687A
(Paper)
RSC Adv., 2017,
7, 20716-20723
Biodegradation of aliphatic and aromatic hydrocarbons using the filamentous fungus Penicillium sp. CHY-2 and characterization of its manganese peroxidase activity
Received
26th December 2016
, Accepted 27th March 2017
First published on 11th April 2017
Abstract
The aim of this work was to study the potential of the non-lignolytic filamentous fungus Penicillium sp. CHY-2, isolated from Antarctic soil, for the biodegradation of eight different aliphatic and aromatic hydrocarbons such as octane, decane, dodecane, ethylbenzene, butylbenzene, naphthalene, acenaphthene, and benzo[a]pyrene. Among all the compounds, CHY-2 showed the highest level of degradation for decane (49.0%), followed by butylbenzene (42.0%) and dodecane (33.0%), and lower levels of degradation for naphthalene (15.0%), acenaphthene (10.0%), octane (8.0%), ethylbenzene (4.0%), and benzo[a]pyrene (2.0%) at 20 °C. The addition of carbon sources such as glucose (5 g L−1) and Tween-80 (5 g L−1) enhanced decane degradation by about 1.8-fold and 1.61-fold respectively at 20 °C. The metabolites produced during the degradation of decane were identified by gas chromatography-mass spectrometry (GC-MS). Furthermore, the enzyme manganese peroxidase (MnP) from CHY-2 was purified. MnP was found to consist of monomers with a molecular mass of 36 kDa. The purified MnP had an optimum pH of 5.0 and temperature of 30 °C. The Km and Vmax values of MnP towards Mn2+ were 1.31 μM and 185.19 μM min−1 respectively. These results indicated that the strain CHY-2 can be used for the degradation of hydrocarbons and could have promising applications in treatment of hydrocarbon contaminated sites.
Introduction
Hydrocarbons are one of the most common pollutants that are accumulated in the soil environment due to a variety of anthropogenic activities, and they potentially cause harmful effects on human health.1 The hydrophobic and tenacious nature of polycyclic aromatic hydrocarbons (PAHs) causes them to alter the biological and physicochemical properties of soil, such as the microbial population, soil enzyme activities, water holding capacity, and soil organic matter.2,3 Due to their negative effects on the environment and tendency to migrate into water bodies, there is an urgent desire to be able to remove PAHs from soil.4 Physico-chemical methods for the removal of PAHs are highly efficient but they change the soil structure, reduce the soil microbial population and lead to the depletion of nutrients that are essential for plant vegetation.5,6
Biodegradation is a biological process that utilizes natural microbial populations for the rapid elimination of PAHs from soil.7 The biodegradation of PAHs by microorganisms has been demonstrated as an efficient alternative to remove PAHs from contaminated soil.8 Several studies have been reported about PAH degradation using microorganisms.9–11 Among other microorganisms, fungi have certain advantages over bacteria with respect to biodegradation due to their resistance to PAHs.12
Filamentous fungi Penicillium sp. have received much attention as potential candidates for the degradation of a wide range of PAHs.13 The rapid growth rate, ability to grow under different environmental conditions, and ability to utilize a wide range of substrates as carbon and nitrogen sources enhanced the application of Penicillium sp. in biodegradation studies.14 Furthermore, numerous studies have reported the potential of white rot fungi for the biodegradation of PAHs and their extracellular manganese peroxidase activity.15,16 To date, most of the studies have reported about Penicillium sp. isolated from contaminated soil and water, while there is very little information available about Penicillium sp. isolated from Antarctic soil,17 a low temperature region of the Earth’s last pristine wilderness. The environmental characteristics of Antarctica allow the fungus to thrive under low temperature conditions with diverse biodegradation potential. However, there are no reports about the degradation of PAHs by filamentous fungi Penicillium sp., under low temperature conditions.
Our previous study reported the biodegradation of alkylphenols by Penicillium sp. CHY-2 isolated from Antarctic soil.18 Consequently, the present work demonstrates the ability of Penicillium sp. CHY-2 to degrade hydrocarbons and PAHs under low temperature conditions and suggests that Penicillium sp. CHY-2 may be a useful tool for the biodegradation of PAHs in contaminated environments under low temperature conditions. In addition, the degradation ability of Penicillium sp. CHY-2 towards 8 different toxic hydrocarbons, octane, decane, dodecane, ethylbenzene, butylbenzene, naphthalene, acenaphthene, and benzo[a]pyrene, was evaluated. The influence of different temperatures and pH levels on the biodegradation of PAHs by Penicillium sp. CHY-2 was also evaluated. Furthermore, the purification and characterization of MnP produced by Penicillium sp. CHY-2 were performed, and the PAH degradation potential of the purified MnP was evaluated under different temperature and pH conditions. In this study, the CHY-2 strain exhibited a great capability to degrade various hydrocarbons including decane under low and medium temperature conditions. In addition, MnP enzyme activities were observed during fungal growth on these compounds. These results suggest that the fungus CHY-2 has the ability to degrade aromatic and aliphatic hydrocarbons present in contaminated soils.
Materials and methods
Strain and culture conditions
Penicillium sp. CHY-2 (accession no: AB980801) was characterized in our previous study.18 Stock cultures of CHY-2 were maintained in potato dextrose agar (PDA) medium at 4 °C. Penicillium sp. CHY-2 was first grown in 100 mL of potato dextrose broth in a 250 mL Erlenmeyer flask at 28 °C for 5 days with a shaking speed of 200 rpm.
Chemicals
Octane, decane, dodecane, ethylbenzene, butylbenzene, naphthalene, acenaphthene, and benzo[a]pyrene were purchased from Tokyo chemicals (Tokyo, Japan) at a 97% purity level or higher. All chemicals used in this study were of analytical grade.
Biodegradation of different hydrocarbons and PAHs by Penicillium sp. CHY-2
Biodegradation experiments were performed in 100 mL serum bottles containing 50 mL of mineral glucose (MG) liquid medium. The MG medium contains the following composition: 1 g L−1 K2HPO4; 0.05 g L−1 NaCl; 0.2 g L−1 MgSO4·7H2O; 0.05 g L−1 CaCl2; 0.0083 g L−1 FeCl3·6H2O; 0.014 g L−1 MnCl2·4H2O; 0.017 g L−1 NaMoO4·2H2O; 0.001 g L−1 ZnCl2; and 5 g L−1 glucose. The medium pH was adjusted to 6 with 1 N HCl and sterilized in an autoclave for 20 min at 121 °C. Five disks (10 mm diameter) obtained with a cork borer from actively growing fungi on agar were placed into each bottle. The inoculated bottles were pre-cultivated for several days to obtain similar radial growths and minimize growth variation. Various toxic hydrocarbons and PAHs (at a final concentration of 100 mg L−1), such as octane, decane, dodecane, ethylbenzene, butylbenzene, naphthalene, acenaphthene, and benzo[a]pyrene, were injected into separate bottles, and the bottles were incubated in the dark with an agitation speed of 110 rpm under aerobic conditions. Hydrocarbons and PAHs in the liquid medium were extracted and measured by GC. The samples were shaken with equal volumes of hexane for 3 min and centrifuged at 8000g for 10 min. The organic layer was then extracted and analysed directly by GC-FID (flame ionization detection). The GC-MS analysis was conducted on a Shimadzu GC-MS system (GC-2014) with a DB-5MS capillary column (J & W scientific). All samples were performed in triplicate and the results were expressed as mean values and standard deviations.
Optimization studies for decane degradation
The influence of temperature and pH on the decane degradation capacity of the CHY-2 strain was investigated under aerobic conditions. Batch experiments were conducted in 100 mL serum bottles containing 50 mL of the MG medium with different initial pH conditions (pH 5–9). Three disks (10 mm diameter) of actively growing fungi on agar were placed into each serum bottle containing the MG liquid medium. 100 mg L−1 decane was added to each bottle and the bottles were incubated for up to 28 days at different temperatures (4, 10, and 20 °C) with an agitation speed of 110 rpm. Control experiments were performed without inoculation of the culture.
Effect of glucose
A monohyphal culture of CHY-2 was obtained by single spore isolation. The culture was maintained on 2% MG agar plates. Three different concentrations of glucose (1, 5, and 10 g L−1) were used. Uniform circular agar blocks containing a CHY-2 mycelial mat were cut from the 10 day old culture plate and transferred in to a sterilized 25 mL basal medium with 100 mg L−1 decane. The inoculated bottles were incubated at 4 and 20 °C in a shaking incubator at 110 rpm. After 10 days of incubation, the cultures were spread on Petri plates containing different concentrations of glucose (1, 5, and 10 g L−1).
Effect of Tween 80
Batch experiments were carried out in 50 mL serum bottles containing 20 mL MG medium with and without Tween 80 addition. Four different concentrations of Tween 80 (1, 2.5, 5, and 10 g L−1) were used. Briefly, three disks (10 mm diameter) of actively growing CHY-2 were placed into each serum bottle containing the liquid medium. 100 mg L−1 decane was added to each bottle and the bottles were incubated for 28 days at 4 and 20 °C with an agitation speed of 110 rpm. The effect of Tween 80 on decane degradation was observed according to Chang et al.18
Analysis of decane metabolites by GC-MS
The metabolites produced during decane degradation were determined by GC-MS. The samples were shaken with equal volumes of hexane for 3 min and centrifuged at 8000g for 10 min. The organic layer was then extracted and analyzed directly by GC-MS. Prior to GC-MS analysis the extract was dried under a nitrogen flow and derived by trimethylsilylation (TMS) using an N,O-bis(trimethylsilyl)trifluoroacetamide (BSTFA)/acetonitrile solution at 60 °C for 1 h. The GC-MS analysis was conducted on a Shimadzu GC-MS system (GCMS-QP2010) with an Rxi-5ms capillary column (30 m, 0.25 mm Id, 1.00 mm df; Restek, PA, USA).
Enzyme assay
The activity of MnP from the culture filtrates was measured according to the method described by Wariishi et al.19 In brief, the reaction mixture (1.0 mL) contained 50 mM malonic acid disodium salt monohydrate (pH 4.5), 1.0 mM manganese(II) sulfate, 0.1 mM hydrogen peroxide (H2O2) and the enzyme solution. The reaction was monitored for the formation of Mn3+ malonate complexes at 270 nm. One unit (U) of MnP activity was defined as the amount of enzyme required to produce 1 M Mn3+ from the oxidation of Mn2+ per minute.
Enzyme purification
A four day old culture of Penicillium sp. CHY-2 was centrifuged for 10 min at 13
000g. The supernatant was filtered through a 0.45 μm filter (Advantec, Dismic-25AS, Bedford, USA). The filtrate served as the crude enzyme and it was subjected to various purification steps.
Ion-exchange chromatography
The first purification step involved a High Q cartridge (BioRAD, USA) column (5 mL, 40 mm × 12.6 mm inner diameter), which had been equilibrated with 50 mM Tris–HCl buffer at pH 8.0. The flow rate was maintained at 0.5 mL min−1, and a total of 60 1.0 mL fractions were collected. The protein concentration was determined for each fraction from the absorbance at 595 nm using the Bradford method. The MnP activity was then estimated as mentioned above for each fraction at 270 nm. The fractions with high activity were collected and pooled.20
Gel filtration
The High Q cartridge fraction containing the MnP enzyme was concentrated to 1.0 mL by ultra-filtration using a 10
000 Da cut-off membrane at 4 °C. The enzyme solution was then loaded onto a HiPrep 16/60 Sephacryl S-300 (GE Healthcare Sweden) column (120 mL) which was pre-equilibrated with 50 mM PB (pH 7.2) and eluted with the same buffer. Fractions of 1.0 mL each were collected. All the fractions with high A270 and high MnP activity were pooled. The pooled samples were concentrated by ultra-filtration using a 10
000 Da cut-off membrane at 4 °C, and used for characterization.21
SDS-PAGE
The molecular mass of the purified enzyme was determined by sodium dodecyl sulfate-polyacrylamide gel electrophoresis (SDS-PAGE).22 SDS-PAGE was run on a 12.5% polyacrylamide gel.
Effect of pH and temperature on the activity of MnP
The effect of pH on the MnP activity was studied at different pH values (3–7). In brief, the MnP was pre-incubated in 50 mM malonic acid buffer (pH 2.0–5.5) and 50 mM potassium phosphate buffer (pH 6.0–7.0) of different pH values in the range of 3–8 for 24 h at 30 °C. After incubation, the residual enzyme activity was determined under standard assay conditions. Similarly, the effect of temperature on the MnP activity also determined at different temperatures between 4 and 40 °C. Untreated enzyme was used as a control.
Kinetic analysis of MnP
The kinetic parameters of MnP toward Mn2+ were determined according to Zeng et al.23 The rate of oxidation was determined by spectrophotometry using the reported molar extinction coefficient (ε) values at the maximal absorption wavelengths. The reaction was conducted in 50 mM malonic acid buffer and MnP (pH 5.5). The Km and Vmax values for the purified MnP from Penicillium sp. CHY-2 were calculated using the Lineweaver–Burk transformation of the Michaelis–Menten equation.24
Decane degradation by MnP
The decane degradation ability of purified MnP was determined according to Zeng et al.23 with minor modifications. Briefly, the purified MnP was incubated with decane for 48 h under the optimum pH (pH 5.5) and temperature (30 °C). After the desired incubation period, the degradation rate was assayed using GC-MS. Control experiments were performed without the inoculation of MnP.
Statistical analysis
All the results presented in this study were mean values of three replicates of experiments, and standard deviations were used to analyse the data. Statistical analysis was performed using Microsoft Excel 2013.
Results and discussion
Biodegradation of 8 different hydrocarbons and PAHs by Penicillium sp. CHY-2
The genus Penicillium is a group of fungi that exhibits a wide range of metabolic activities, including the degradation of various toxic compounds.18 Penicillium sp. has been demonstrated to as a potential fungus for the bioremediation of a wide range of hydrocarbons and PAHs.25 Singh26 reported that Penicillium sp. is capable of using hydrocarbons as carbon and energy sources. Therefore, in the present study, Penicillium sp. CHY-2 was selected for the degradation of different hydrocarbons and PAHs. Biodegradation of the eight different hydrocarbons and PAHs at two different concentrations (100 and 500 mg L−1) was investigated using the CHY-2 strain at 20 °C (Fig. 1). At 100 mg L−1 concentration, among all the compounds studied, CHY-2 showed the highest levels of degradation for decane (49.0%), followed by butylbenzene (42.0%) and dodecane (33.0%), and lower levels of degradation for naphthalene (15.0%), acenaphthene (10.0%), octane (8.0%), ethylbenzene (4.0%), and benzo[a]pyrene (2.0%). At 500 mg L−1 concentration, among all the compounds studied, CHY-2 showed the highest levels of degradation for decane (34.0%) followed by butylbenzene (25.0%), and lower levels of degradation for naphthalene (7.0%) and benzo[a]pyrene (1.0%). However, other compounds such as octane, dodecane, ethylbenzene, and acenaphthene were not degraded by the strain. The rate of degradation was measured on day 28 at 20 °C. Apart from the white rot fungi, the filamentous Penicillium sp. also has the capacity to degrade a wide variety of organo-pollutants, including PAHs, phenolic compounds and heavy metals.25 Boonchan et al.27 reported that Penicillium janthinellum can oxidize PAHs into hydrophilic or detoxified products. Launen et al.28 reported that filamentous Penicillium janthinellum was capable of utilizing pyrene as a sole carbon and energy source. Degradation experiments showed that Penicillium janthinellum could oxidize or detoxify PAHs, and the mineralization of these compounds was not detected. In this study, the CHY-2 strain could effectively degrade hydrocarbons and PAHs. A significant increment of the degradation rate was obtained at the concentration of 100 mg L−1. Among the eight different hydrocarbons and PAHs, decane showed the highest percentage of degradation at both 100 and 500 mg L−1 concentrations. Therefore further studies were carried out on the degradation of decane using the CHY-2 strain.
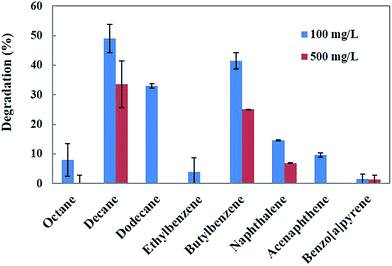 |
| Fig. 1 Biodegradation of different hydrocarbons and PAHs by Penicillium sp. CHY-2. | |
Optimization of decane degradation using CHY-2
To investigate the effect of temperature and pH on decane (at 100 mg L−1 concentration) degradation by Penicillium sp. CHY-2, batch experiments were carried out at different temperatures (4, 10, and 20 °C) and different pH conditions (5–9) in a MG liquid medium. The CHY-2 strain grown at 20 °C for 28 days showed the highest percentage of decane removal (75%), followed by that for 14 days (73%) and 7 days (72%) of incubation time (Fig. 2). However, moderate removal of decane was observed at 10 °C at 28 days (61%), 14 days (54%), and 7 days (40%). An interesting phenomenon was observed in this study, as the CHY-2 strain was able to degrade the decane even at 4 °C. At 28 days 37% of decane degradation was observed followed by 27% at 14 days, and 9% at 7 days at a temperature of 4 °C.
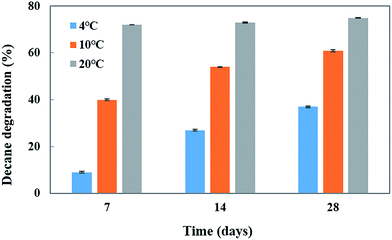 |
| Fig. 2 Effect of temperature on decane degradation using CHY-2. | |
pH is an important abiotic factor which affects the microbial metabolism. Consequently, its effect on decane degradation was studied. The initial pH significantly influenced the growth and degradation rate of the CHY-2 strain under acidic conditions (pH 4.0–6.0) (Fig. 3). Decane degradation was high at pH 6.0 (81%) followed by that at pH 8.0 (56%) and pH 7 (40%). The results indicate that the CHY-2 strain is able to degrade decane under acidic conditions rather than alkaline conditions.
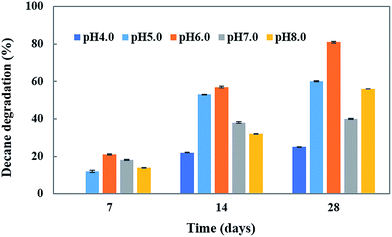 |
| Fig. 3 Effect of pH on decane degradation using CHY-2. | |
Effect of glucose on decane degradation
Generally, microorganisms require suitable growth conditions (e.g., carbon sources, nutrients, temperature, pH, redox potential and oxygen content) which strongly affect their growth. The carbon source of the growth medium is an important factor for the growth and metabolism of a microorganism. Selection of an ideal and inexpensive carbon source accelerates the growth of the fungus as well as the bioremediation process.29 Our previous study18 identified glucose as the best carbon source for the biodegradation of 4-tert-butylphenol (4-BP) by the CHY-2 strain. Thus, we selected glucose as the carbon source for decane degradation in this study (Fig. 4). The results showed that 1 and 5 g L−1 concentrations of glucose resulted in 85.3 and 88.3% decane degradation. However, 10 g L−1 of glucose resulted in a lower level of decane degradation (64.3%) than 5 g L−1 of glucose in the medium. Based on the results, it was concluded that 5 g L−1 of glucose resulted in the highest removal of decane. The medium without glucose served as the control in this experiment. Chang et al.18 reported that the cultures promoted the degradation activity of CHY-2 at 1 g L−1 glucose concentration. Ron and Rosenberg30 reported that adding glucose as the carbon source resulted in higher rates of n-eicosane degradation compared to the control. Furthermore, the addition of a carbon source accelerates the biodegradation of petroleum hydrocarbon pollutants.
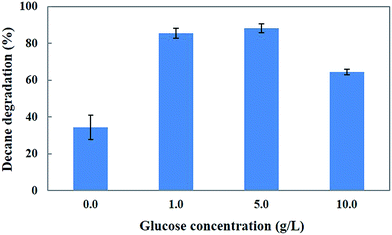 |
| Fig. 4 Effect of glucose on decane degradation using CHY-2. | |
Effect of Tween 80 on decane degradation
Tween 80 is a water soluble synthetic compound which enhances the biodegradation and bioremediation of toxic pollutants. Thus, the influence of Tween 80 at different concentrations (1–10.0 g L−1) and temperatures (4 and 20 °C) on decane degradation was investigated (Fig. 5). The results show that an increased concentration of Tween 80 (1, 2.5 and 5 g L−1) in the medium causes a gradual increase in decane degradation (55.0%, 74.5%, and 79.3%) at 20 °C. However, a 10 g L−1 concentration of Tween 80 caused a decrease in degradation (57.2%) compared to 5 g L−1 (79.3%) at 20 °C.
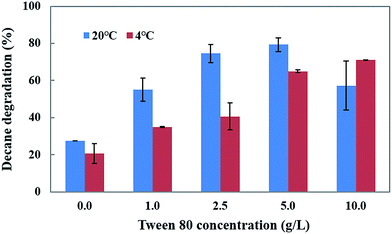 |
| Fig. 5 Effect of Tween-80 on decane degradation using CHY-2. | |
The addition of Tween 80 enhances the decane degradation activity of CHY-2 even at low temperature conditions. The increased concentrations of Tween 80 (1, 2.5, 5, and 10 g L−1) in the medium caused gradual increases in decane degradation (34.9%, 40.5%, 64.9% and 70.9%) at 4 °C. Based on these results, it was concluded that Tween 80 may play an important role in the degradation of toxic compounds in low temperature environments. Garon et al.31 reported that the addition of Tween 80 enhanced the biodegradation of fluorine by Doratomyces stemonitis (46–62%) and Penicillium chrysogenum (28–61%). Wang et al.32 reported that Tween 80 acts as a stimulant for benzene[a]pyrene degradation in contaminated soil. The positive effect of Tween 80 on pyrene biodegradation by PAH-degrading Burkholderia cepacia was observed by Chen et al.33 Budde et al.34 reported that a Ralstonia eutropha culture with Tween 80 showed considerably higher optical densities than the control (without Tween 80), and that Tween 80 can serve as an effective carbon source for R. eutropha. It was established that the Tween molecules contain fatty acid groups that are cleaved by esterase, allowing them to be utilized as a carbon source by many microorganisms.
Determination of decane metabolites
The peak area of decane seen at the retention time of 13 min decreased as the reaction progressed and the metabolites were observed. The peak (peak I) at the retention time of 13 min was identified as 1,6-hexanediol (Fig. 6). The mass spectrum of 1,6-hexanediol is shown in Fig. 6b. The largest molecular ion at m/z 262 was presumed to be a decane derivative of 1,6-hexanediol. The mass spectrum corresponds to that of a previous report of decane metabolites.35
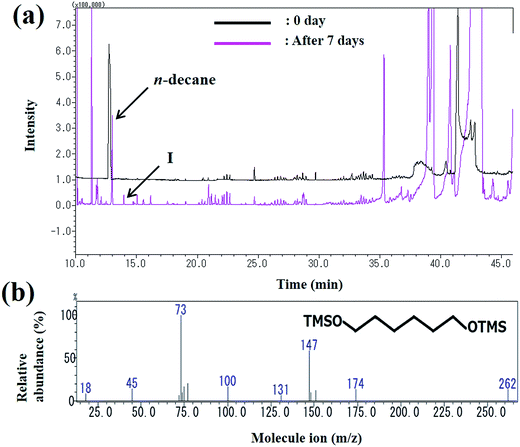 |
| Fig. 6 (a) Gas chromatogram of the intermediates produced during decane degradation; (b) mass spectrum of the metabolite (1,6-hexanediol) identified by GC-MS. | |
Purification of MnP from Penicillium sp. CHY-2
MnP secreted by Penicillium sp. CHY-2 was purified as described in Table 1. The crude enzyme obtained from strain CHY-2 contained 1.0492 U of MnP. The crude MnP was fractionated by ion exchange chromatography at pH 8.0. The fractions corresponding to MnP were collected and further purified by gel filtration chromatography (Fig. 7a and b). The elution pattern on the column showed 4 major peaks at 270 nm. Similar MnP peaks were observed by Zeng et al.23 The fractions with high MnP activity were pooled, concentrated and loaded onto a HiPrep 16/60 Sephacryl S-300 (GE Healthcare Sweden) column. The molecular weight of the purified MnP was analyzed by SDS-PAGE. The purified MnP from the CHY-2 strain revealed a single band in SDS-PAGE with an estimated molecular mass of 36.0 kDa (Fig. 7c). Molecular masses of fungal MnPs usually range from 32.0 to 75.0 kDa.36,37 Furthermore, gel filtration using a Sephacryl S-300 column was performed in order to determine the size and subunit composition of MnP. A single peak was observed for the native form of MnP with a relative molecular mass estimated to be 36 kDa (Fig. 7d). This result, correlated with the observations made through SDS-PAGE, indicates that the native form of MnP has a monomer conformation.21 MnP is a lignolytic enzyme produced by most white-rot fungi.38 However, some filamentous fungal species like Penicillium also have been reported as MnP producing fungi. Urek et al.39 reported the production of MnP by P. chrysosporium with a molecular mass of 45 kDa.
Table 1 Purification steps of MnP from Penicillium sp. CHY-2
Purification step |
Activity (U) |
Protein (mg mL−1) |
Specific activity (μmol min−1 mg−1 of protein) |
Crude enzyme |
1.0492 |
0.0175 |
59.95 |
Ion-exchange |
0.2278 |
0.0009 |
253.00 |
Gel filtration |
0.0414 |
0.0001 |
414.00 |
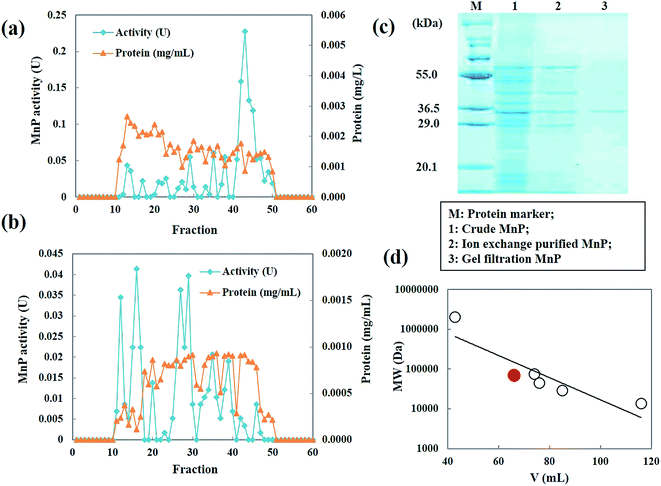 |
| Fig. 7 Elution profile of the MnP from Penicillium sp. CHY-2. (A) Ion-exchange chromatography; (B) gel filtration chromatography; (C) SDS-PAGE of purified MnP; (D) molecular mass of MnP. | |
Effect of pH and temperature on the activity of MnP
Both pH and temperature play an important role in determining the activity and stability of enzymes.40 The effect of pH on the activity profiles of MnP was investigated at 30 °C with different pH values (pH 3.0–7.0) in 50 mM malonic acid (pH 2.0–5.5), and 50 mM potassium phosphate (pH 6.0–7.0) buffers. As shown in Fig. 8a the optimum pH value was estimated to be 5.0. The pH value appeared to be critical for MnP activity. The maximum MnP activity is within a close range of the previously reported MnP produced by Phanerochaete chrysosporium.23 The highest MnP activity exhibited at pH 5.0 is a very important attribute that lends further support to the potential use of MnP for the degradation of hydrocarbons and PAHs. Muhammad Asgher et al.40 reported that increases in the pH caused a rapid decrease in activity, indicative of enzymatic deactivation at non-optimal pH values. The effect of temperature on the MnP activity was investigated in the temperature range of 4–40 °C. The optimum temperature of MnP was observed at 30 °C (Fig. 8b). Similarly the optimum temperature for white-rot fungus Phanerochaete chrysosporium MnP was obtained at 30 °C.23,39 Bilal et al.41 reported that MnP showed better activity at higher temperatures, which may be due to the strengthening of the protein molecule’s structural rigidity when it binds to the solid support, reducing the molecular flexibility.
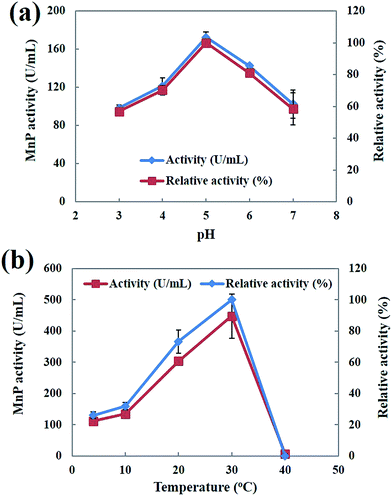 |
| Fig. 8 (a) Effect of pH on MnP activity; (b) effect of temperature on MnP activity. | |
Kinetic analysis of MnP
The substrate specificity analysis indicated that MnP was able to oxidize the Mn2+ substrate. The kinetic constants of MnP with the Mn2+ substrate are shown in Table 2. The Km and Vmax values for MnP towards Mn2+ were 1.31 μM and 185.19 μM min−1 respectively. The substrate specificity analysis elucidates the characteristics of toxic compound degradation.
Table 2 Kinetic parameters of MnP from Penicillium sp. CHY-2
Km (μM) |
1.31 |
Vmax (μM min−1) |
185.19 |
kcat (min−1) |
1395.06 |
kcat/Km (μM−1 min−1) |
1061.03 |
kd (—) |
1.25 |
Decane degradation by MnP
Fig. 9 shows the degradation rate of decane after treatment with MnP in the batch experiments. A maximum of 25.5% decane degradation was observed after 48 h under optimum pH (pH 5.5) and temperature (30 °C) conditions. The results indicate that the MnP preparations resulted in a moderate decane biodegradation efficiency, therefore MnP has potential to be used for decane biodegradation. The potential of MnP in decane degradation may involve the formation of MnP–H2O2–Mn2+ during continuous treatment, by combining the action of the enzyme, Mn2+, an organic chelator and hydrogen peroxide, to increase hydrocarbon solubility.42
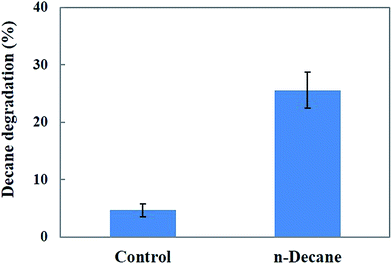 |
| Fig. 9 Effect of MnP on decane degradation. | |
Conclusions
A novel fungal strain, Penicillium sp. CHY-2, isolated from Antarctic soils, was effective for the degradation of decane at lower (20 °C) and medium (30 °C) temperatures. The degradation of decane (49.0%) by CHY-2 indicated its hydrocarbon degradation ability. Glucose was found to be the most suitable carbon source for the growth of CHY-2, and the addition of Tween 80 enhanced the growth and degradation ability of CHY-2 towards decane. The MnP from CHY-2 was purified, and showed good activity at an optimal pH and temperature of 5.0 and 30 °C respectively. The results suggest that the CHY-2 strain and the MnP produced may be considered as potential candidates for the degradation of decane.
Acknowledgements
Dr M. Govarthanan gratefully acknowledges the Muroran Institute of Technology for providing Postdoctoral fellowship. This work was supported by a Grant-in-Aid for Scientific Research C (26340067) from the Japan Society for the Promotion of Science. Thanks to Dr M. V. Reddy and Dr Yuka Yajima for their helpful discussion.
References
- S. Kuppusamy, P. Thavamani, M. Megharaj and R. Naidu, Int. Biodeterior. Biodegrad., 2016, 108, 149–157 CrossRef CAS.
- S. J. Robertson, W. B. McGill, H. B. Massicotte and P. M. Rutherford, Biol. Res., 2007, 82, 213–240 CrossRef PubMed.
- C. O. Nwoko, Journal of Geoscience and Environment Protection, 2014, 2, 9–14 CrossRef.
- M. Rajtor and Z. Piotrowska-Seget, Chemosphere, 2016, 162, 105–116 CrossRef CAS PubMed.
- A. G. Khan, C. Kuek, T. M. Chaudhry, C. S. Khoo and W. J. Hayes, Chemosphere, 2000, 41, 197–207 CrossRef CAS PubMed.
- S. Gan, E. V. Lau and H. K. Ng, J. Hazard. Mater., 2009, 172, 532–549 CrossRef CAS PubMed.
- M. Hassanshahian, G. Emtiazi and S. Cappello, Mar. Pollut. Bull., 2012, 64, 7–12 CrossRef CAS PubMed.
- K. Kubota, D. Koma, Y. Matsumiya, S. Y. Chung and M. Kubo, Biodegradation, 2008, 19, 749–757 CrossRef CAS PubMed.
- X. Song, Y. Xu, G. Li, Y. Zhang, T. Huang and Z. Hu, Mar. Pollut. Bull., 2011, 62, 2122–2128 CrossRef CAS PubMed.
- D. Hou, X. Shen, Q. Luo, Y. He, Q. Wang and Q. Liu, Mar. Pollut. Bull., 2012, 67, 146–151 CrossRef PubMed.
- V. Patel, J. Patel and D. Madamwar, Mar. Pollut. Bull., 2013, 74, 199–207 CrossRef CAS PubMed.
- H. Harms, D. Schlosser and L. Y. Wick, Nat. Rev. Microbiol., 2011, 9, 177–192 CrossRef CAS PubMed.
- K. A. Hughes and P. Bridge, Tolerance of Antarctic soil fungi to hydrocarbons and their potential role in soil bioremediation, in Polar Microbiology: The Ecology, Biodiversity and Bioremediation Potential of Microorganisms in Extremely Cold Environments, Taylor and Francis, Boca Raton, FL, 2010, pp. 277–300 Search PubMed.
- K. Steffen, A. Hatakka and M. Hofrichter, Appl. Microbiol. Biotechnol., 2002, 60, 212–217 CrossRef CAS PubMed.
- P. Baborova, M. Moder, P. Baldrian, K. Cajthamlova and T. Cajthaml, Res. Microbiol., 2006, 157, 248–253 CrossRef CAS PubMed.
- G. Eibes, T. Cajthaml, M. T. Moreira, G. Feijoo and J. M. Lema, Chemosphere, 2006, 64, 408–414 CrossRef CAS PubMed.
- E. Kerry, Polar Biol., 1990, 10, 293–299 Search PubMed.
- Y. C. Chang, S. Fuzisawa, M. V. Reddy, H. Kobayashi, E. Yoshida, Y. Yajima, T. Hoshino and D. Choi, Clean: Soil, Air, Water, 2016, 44, 1–9 Search PubMed.
- H. Wariishi, L. Akileswaranand and M. H. Gold, Biochemistry, 1988, 27, 5365–5370 CrossRef CAS PubMed.
- M. B. Martel, R. Letoublon and M. Fevre, Curr. Microbiol., 1996, 33, 243–248 CrossRef CAS PubMed.
- J. A. Tamayo-Ramos, W. J. H. van Berkel and L. H. de Graaff, Microb. Cell Fact., 2012, 11, 165 CrossRef CAS PubMed.
- U. K. Laemmli, Nature, 1970, 227, 680–685 CrossRef CAS PubMed.
- G. M. Zeng, M. H. Zhao, D. L. Huang, C. Lai, C. Huang, Z. Wei, P. Xu, N. J. Li, C. Zhang, F. L. Li and M. Cheng, Int. Biodeterior. Biodegrad., 2013, 85, 166–172 CrossRef CAS.
- J. E. Dowd and D. S. Riggs, J. Biol. Chem., 1965, 240, 863–869 CAS.
- A. L. Leitao, Int. J. Environ. Res. Public Health, 2009, 6, 1393–1417 CrossRef CAS PubMed.
- H. Singh, Mycoremediation: Fungal Bioremediation, Wiley-Interscience, New York, 2006 Search PubMed.
- S. Boonchan, M. L. Britzand and G. A. Stanley, Appl. Environ. Microbiol., 2000, 66, 1007–1019 CrossRef CAS PubMed.
- L. Launen, L. J. Pintoand and M. M. Moore, Appl. Microbiol. Biotechnol., 1999, 51, 510–515 CrossRef CAS PubMed.
- M. V. Reddy and S. V. Mohan, Bioresour. Technol., 2012, 114, 573–582 CrossRef PubMed.
- E. Z. Ron and E. Rosenberg, Curr. Opin. Biotechnol., 2014, 27, 191–194 CrossRef CAS PubMed.
- D. Garon, S. Krivobok, D. Wouessidjewe and F. Seigle-Murandi, Chemosphere, 2002, 47, 303–309 CrossRef CAS PubMed.
- C. Wang, H. Liu, J. Li and H. Sun, Environ. Sci. Pollut. Res., 2014, 21, 10614–10625 CrossRef CAS PubMed.
- K. Chen, Q. Zhu, Y. Qian, Y. Song, J. Yao and M. M. F. Choi, Ecotoxicol. Environ. Saf., 2013, 98, 361–367 CrossRef CAS PubMed.
- C. F. Budde, S. L. Riedel, F. Hubner, R. Risch, M. K. Popovic, C. K. Rha and A. J. Sinskey, Appl. Microbiol. Biotechnol., 2011, 89, 1611–1619 CrossRef CAS PubMed.
- K. Syed, A. Porollo, Y. W. Lam, P. E. Grimmett and J. S. Yadav, Appl. Environ. Microbiol., 2013, 79, 2692–2702 CrossRef CAS PubMed.
- C. G. Boer, L. Obici, C. G. M. de Souza and R. M. Peralta, Bioresour. Technol., 2004, 94, 107–112 CrossRef CAS PubMed.
- P. L. de Oliveira, M. C. T. Duarte, A. N. Ponezi and L. R. Durrant, Braz. J. Microbiol., 2009, 40, 818–826 CrossRef PubMed.
- F. Peláez, M. J. Martinez and A. T. Martinez, Mycol. Res., 1995, 99, 37–42 CrossRef.
- R. O. Urek and N. K. Pazarlioglu, Process Biochem., 2004, 39, 2061–2068 CrossRef CAS.
- M. Asgher, M. Ramzan and M. Bilal, Chin. J. Catal., 2016, 37, 561–570 CrossRef CAS.
- M. Bilal, M. Iqbal, H. Hu and X. Zhang, Biochem. Eng. J., 2016, 109, 153–161 CrossRef CAS.
- G. Eibes, T. Lú Chau, G. Feijoo, M. T. Moreira and J. M. Lema, Enzyme Microb. Technol., 2005, 37, 365–372 CrossRef CAS.
|
This journal is © The Royal Society of Chemistry 2017 |
Click here to see how this site uses Cookies. View our privacy policy here.