DOI:
10.1039/C6RA28551A
(Paper)
RSC Adv., 2017,
7, 21446-21451
A highly zinc-selective ratiometric fluorescent probe based on AIE luminogen functionalized coordination polymer nanoparticles†
Received
22nd December 2016
, Accepted 9th April 2017
First published on 20th April 2017
Abstract
Coordination polymer nanoparticles (CPNs) formed by self-assembly of metal ions (or clusters) and organic bridging ligands through coordination bonds provide a unique platform for designing multifunctional nanoparticles. In this work, we report a ratiometric fluorescent probe for Zn2+ based on CPNs, which were prepared from AIE fluorophore HDBB molecules with metal ions. The CPNs are composed of HDBB molecules with Tb3+ (named Tb-HDBB-CPNs) which displayed a matrix coordination-induced emission peak at a wavelength of 590 nm, while CPNs formed by HDBB molecules and Zn2+ (named Zn-HDBB-CPNs) showed a distinctive fluorescent property with a blue emission peak wavelength of 470 nm. Based on the cation exchange process of Tb-HDBB-CPNs with Zn2+, a highly selective ratiometric fluorescent probe for the determination of Zn2+ in aqueous solution was developed with a linear range from 0.1 to 60 μM and a detection limit of 50 nM. Our approach using AIE molecules as organic ligands for the construction of CPNs paves a way toward AIE functionalized materials with ratiometric fluorescence response and will find wide applications in chemical sensing.
1. Introduction
Coordination polymer nanoparticles, which are made from metal ions (or clusters) connected by organic ligands, have attracted growing interest because of their structural tailorability with different morphologies and physicochemical properties.1,2 To date, CPNs have been widely studied for their potential applications in gas storage and separation, heterogeneous catalysis, chemical sensing, biomedical imaging and drug delivery.3–11 Nevertheless, the rational design of functional coordination polymers nanoparticles is still in an premature, primary stage.12 Fluorescent CPNs have demonstrated their considerable potential in chemical sensing because of their hybrid structures that can offer tunable fluorescence and have been investigated in the determination of ion, drug and enzyme activity.13–16 However, the development of fluorescent CPNs has suffered from the problem of aggregation-caused quenching (ACQ), in which chromophores that show high fluorescence quantum yields in dilute solutions become nonfluorescent in the colloidal or solid state, where intermolecular interactions, such as π-stacking, often cause self-quenching.17,18
The concept of aggregation-induced emission or aggregation-induced enhanced emission (AIEE) has emerged to be a powerful and versatile strategy for the design of novel types fluorescent probes and luminescent materials to overcome ACQ problem.19–21 In this process, the fluorogens are almost non-emissive as individual molecule because of the nonradiative decay through intramolecular motion. Such intramolecular motion can be restricted once these molecules aggregate together, resulting in strong fluorescence. A typical example is tetraphenylethene (TPE) molecule, a well-known AIE fluorogen, in which the olefin stator is surrounded by phenyl rotors and the restriction of intramolecular motion (RIM) is known to account for its strong fluorescence in colloids and in the solid state.22,23 AIE-based luminescent materials not only avoid the ACQ problem caused by aggregation but also provide new approaches based on turn-on mode in the applications of chemical sensors,24–29 biological probes,30–33 and solid-state emitters.34,35
Recently, some TPE-based AIE molecules were utilized as organic ligands in the construction of metal–organic frameworks (MOFs), which is an intriguing class of inorganic–organic hybrid crystalline materials.36,37 These studies demonstrated that rigidifying AIE molecules as fluorescent links by MOFs formation efficiently and substantially tune the electronic transition energies and raise the florescent quantum yield, owing to twisted linker conformation, intramolecular hindrance, and framework rigidity.38 The applications of TPE-based MOFs with excellent luminescent behaviors are well explored as light-emitting diodes (LED), drug vehicles and chemical sensor.39–42 On the other hand, to the best of our knowledge, the utilization of AIE molecules as organic ligands in the amorphous CPNs has not been investigated. Different from the crystal CPNs with static structures, the amorphous CPNs exhibit a higher level of structural tailorability. Their structures can be depolymerized much faster under milder conditions.43 Moreover, the preferred turn-on responsive fluorescence when exposed to analytes was not obvious due to the almost complete restriction of intramolecular motion (RIM) in the ligands after MOFs formation.39,40 One way to overcome this disadvantage is developing ratiometric fluorescent probes, which display two distinctly different measurable signals in the presence and absence of analyte.
Zinc is the second most abundant heavy metal ion, after iron, in the human body and plays important roles in various biological systems. It is an essential element required by all cells with various functions, for example, the control of gene transcription, metalloenzyme function and synaptic vesicles in excitatory nerve terminals.44 Thus, zinc ions are important analyte from the view of biochemistry, medicine and environment.45 Taking envisaged applications into account, especially interesting are zinc ions probes operating in ratiometric fluorimetric mode.46
Herein, we report a new type AIE-based CPNs that is prepared from AIE fluorophore HDBB molecules with metal ions. Tb-HDBB-CPNs and Zn-HDBB-CPNs emit different wavelength fluorescence in aqueous solution upon excitation with 365 nm light. The cation exchange process of these AIE-based CPNs were studied by fluorescence emission spectra, energy-dispersive X-ray (EDX) spectra, and inductively coupled plasma (ICP) measurement. A ratiometric fluorescent probe for the detection of Zn2+ was developed on the basis of cation exchange process of Tb-HDBB-CPNs with Zn2+.
2. Experimental section
2.1 Chemicals and materials
2,4-Dihydroxybenzaldehyde, 4-carboxybenzaldehyde, terbium nitrate pentahydrate, zinc nitrate pentahydrate, disodium hydrogen phosphate and sodium dihydrogen phosphate were purchased from Aladdin. 4-Formyl-3-hydroxybenzoic acid were purchased from Bide pharmatech. All other reagents were of analytical grade and used as received. Doubly distilled water was used throughout the experiments. Tris–HCl (Tris = 2-amino-2-hydroxymethylpropane-1,3-diol) buffered solutions were prepared by titrating 0.01 M Tris solutions with a concentrated HCl (1 M) to the required pH values.
2.2 Apparatus
X-ray powder diffraction patterns were taken using a D/max-TTR III X-ray diffractometer (Rigaku, Japan) with a scan speed of 0.1 s per step and a step size of 0.01°. The morphology and composition element of CPNs were characterized by field emission scanning electron microscopy (SEM) (JEM-2100F). The Fourier transform infrared (FT-IR) spectra were obtained on a FT-IR spectrophotometer (Thermo Nicolet 365). UV-Vis absorption was characterized using a UV/Vis/NIR spectrophotometer (Shimadzu, Japan). Fluorescence spectra were recorded on a Hitachi High-Technologies Corporation Tokyo Japan 5J2-0004 model F-7000 FL spectrofluorometer. Fluorescence emission lifetimes were determined on an Edinburgh Analytical Instrument (FLS900 fluorescence spectrometer) with a light-emitting diode lamp (405 nm) and analyzed by the use of a program for exponential fits. ICP-1000 system (Shimadzu, Japan) was utilized to the detection of metal ion concentration. Particle size distribution of HDBB-CPNs was recorded by a dynamic light scattering instrument (BI-90 Plus, Brookhaven Instruments Corp).
2.3 Synthesis of HDBB, L2 and L3
The synthesis of HDBB (4,4′-(hydrazine-1,2-diylidene bis (methanylylidene)) bis (3-hydroxybenzoic acid)), L2 and L3 were the same as our previous report.47 Typically, 4-formyl-3-hydroxybenzoic acid (166.2 mg, 1.0 mmol) was dissolved in methanol (10 mL). Then hydrazine hydrate (24 μL, 0.5 mmol; 80%) was added and refluxed at 75 °C. After refluxing overnight, the resulting precipitate was filtered and washed with 30 mL methanol. After drying, HDBB was obtained as a yellow powder. The molecular structures of HDBB, L2 and L3 was verified by NMR and mass spectroscopy.
2.4 Preparation of CPNs
The preparation of the AIE-based CPNs was performed as reported previously.15 Briefly, Tb-HDBB-CPNs was synthesized by mixing an aqueous solution of Tb(NO3)3·6H2O (26.11 mg, 0.06 mmol) with Tris buffer (10 mL) containing HDBB (3.28 mg, 0.01 mmol), adjusting the pH value to 9.0 to keeping HDBB molecules at dissolved state, and then stirring 2 hours at room temperature to form a yellow suspension. The resulting precipitate was then centrifuged and washed with pH 9.0 Tris buffer solution several times. For the preparation of Zn-HDBB-CPNs, the Tb(NO3)3·6H2O was replaced by Zn(NO3)3·6H2O (11.36 mg, 0.06 mmol) and also get a yellow suspension. The dynamic light scattering measurement has been applied to investigate the stability of these CPNs. The particle sizes of Tb-HDBB-CPNs were about 50 ± 5 nm and 663 ± 28 nm after storing one month and three months in doubly distilled water at 4 °C (Fig. S1†). Zn-HDBB-CPNs displayed similar tendency. These results indicated that the HDBB-CPNs remained stable at least one month.
2.5 Quantification of HDBB, Tb3+, and Zn2+ in CPNs
HDBB molecules were dissolved in pH 11.0 0.1 M phosphate buffer solution to obtain a series of HDBB solutions with concentrations ranging from 5.0 to 200.0 μM. Absorption spectra were recorded with a UV-Vis spectrophotometer. The standard curves were plotted from linear fitting of the absorbance at 365 nm as a function of HDBB concentrations. The samples of Tb-HDBB-CPNs and Zn-HDBB-CPNs were dissolved in pH 11.0 0.1 M phosphate solution. The amounts of HDBB, Tb3+ and Zn2+ in these samples was quantified using UV-Vis spectrophotometer and ICP measurement, respectively.
2.6 Fluorescence quantum yield
The fluorescence quantum yield was calculated from the relation:
where the subscripts s and x indicate the standard and text respectively, ϕ is the fluorescence quantum yield, A corresponds to the absorbance of the solution, F is the fluorescence intensity, and n is the refractive index of the solvent. The quantum yields of Tb-HDBB-CPNs and Zn-HDBB-CPNs are 14.27% and 17.25%, respectively.
2.7 Analysis of real sample
A water sample was collected from Green Lake (Yunnan, China). The sample was filtered through a 0.22 μm membrane (Millipore) prior to be detected. One hundred microliters of the above water sample was added to 900 μL of Tris buffer solution (10 mM, pH = 7.0) containing Tb-HDBB-CPNs (20 μg mL−1) and then analyzed using the developed detection method.
3. Results and discussion
3.1 Preparation and characterization of AIE-based CPNs
A typical route for preparing CPNs is shown as Scheme 1. The Tb-HDBB-CPNs were prepared from HDBB (L1) and terbium nitrate hexahydrate in pH 9.0 Tris buffer solution at room temperature by self-assembly process. The resulting yellow products were purified by centrifugation and washed several times with Tris buffer solution (pH = 9.0). The resulting CPNs were suspended in aqueous solution and could be stored at 4 °C for three months without any changes. The control experiment showed that no CPNs were obtained in the absence of HDBB molecules or Tb3+, suggesting that both HDBB and Tb3+ are essential for the construction of CPNs. The FT-IR spectrum (Fig. 1A and B) measurement of the as-prepared Tb-HDBB-CPNs confirm the coordination of the carboxylate groups to Tb3+, as evidenced by a shift in the C–O stretching frequency to 1650 cm−1. Note that the original C–O stretching frequency of the uncoordinated HDBB was observed at 1692 cm−1. The peak located at 1435 cm−1 also indicated the presence of deprotonated carboxylic acid bonded within the CPNs.48 Zn-HDBB-CPNs show similar IR absorbance behaviors with the one of Tb-HDBB-CPNs, suggesting that the formation of Zn-HDBB-CPNs was underwent similar coordination process between HDBB molecule with Zn2+. To further validate the effect of carboxylate group on the formation of these CPNs, two salicylaldehyde hydrazine derivatives (L2, L3) were designed as organic links in the preparation of CPNs (Scheme 1). The experiment results demonstrate that CPNs can be formed in the presence of L2 with Tb3+, however, there were no CPNs obtained under similar condition with the replacement of L1 by L3 due to the lack of carboxylate group in para-position of L3. From these results, we can conclude that carboxylate group is essential for the formation of these CPNs.
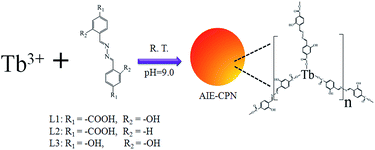 |
| Scheme 1 Schematic representation of the preparation of AIE-based CPN. | |
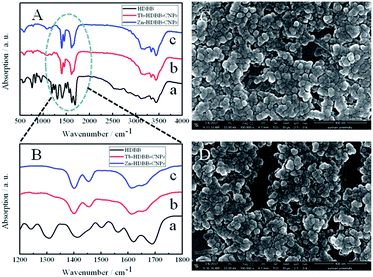 |
| Fig. 1 (A) and (B) FTIR spectra of HDBB (a), Tb-HDBB-CPNs (b), and Zn-HDBB-CPNs (c). FE-SEM images of Tb-HDBB-CPNs (C) and Zn-HDBB-CPNs (D). | |
The SEM images of Tb-HDBB-CPNs and Zn-HDBB-CPNs show typically spherical morphology with relatively uniform size of 50 nm and 55 nm, respectively (Fig. 1C and D). The chemical composition of these CPNs was determined by energy-dispersive X-ray (EDX) analysis during SEM measurement (Fig. S2 and S3†), which demonstrates the presence of Tb and Zn element, further indicating that Tb and Zn ions were involved in the construction of Tb-HDBB-CPNs and Zn-HDBB-CPNs, respectively. The lack of diffraction peaks in powder X-ray diffraction (PXRD) measurement of these CPNs reveals the amorphous structure of these nanoparticles (Fig. S4†).
To further elucidate the structure of these CPNs, the amounts of HDBB molecules and metal ions in these CPNs were quantified using UV-Vis spectra and ICP measurement (Fig. S5–S7†), respectively. The ratios of metal ions to HDBB molecules in these CPNs are about 1
:
1.5 for Tb-HDBB-CPNs and 1
:
1 for Zn-HDBB-CPNs. As a result, the HDBB molecules were in the negative state in the preparation condition (pH = 9.0) of these AIE-based CPNs, and the cations electrostatically attached to the HDBB molecule forming these CPNs. Therefore, the formation of these AIE-based coordination polymers mediated by strong metal–ligand bonding is dominant in the self-assembly process. According to the above results, the structure of Tb-HDBB-CPNs can be proposed and shown in Scheme 1.
3.2 Photochemical properties of AIE-based CPNs
To investigate photochemical properties of these AIE-based CPNs, the fluorescence and absorption spectra were measured. The absorption spectra of these AIE-based CPNs exhibit two peaks at 310 nm and 365 nm (Fig. 2B), which are the same to that of HDBB,47 and the absorption intensities are slightly increasing. These absorptions can be ascribed to the p–p* and n–p* transitions of the entire molecule.
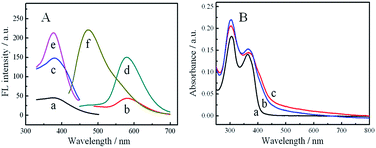 |
| Fig. 2 (A) Excitation and emission spectra of 0.1 mM HDBB (a and b), 0.035 mg mL−1 Tb-HDBB-CPNs (c and d), and 0.035 mg mL−1 Zn-HDBB-CPNs (e and f). The emission spectra were recorded at 365 nm excitation wavelength. (B) UV-Vis spectra of 0.1 mM HDBB (a), 0.035 mg mL−1 Tb-HDBB-CPNs (b), and 0.035 mg mL−1 Zn-HDBB-CPNs (c). | |
The fluorescence spectra shows that the maxima emission and excitation of Tb-HDBB-CPNs are at 590 nm and 365 nm (Fig. 2A), which are similar to the precursor HDBB. The fluorescence quantum yield of Tb-HDBB-CPNs is 14.27% in aqueous solution, which is about three times larger than the one of HDBB (5.32%). The fluorescence enhancement of Tb-HDBB-CPNs can be attributed to the immobilization of the HDBB linker as it is strongly coordinated to Tb3+. The formation of rigid CPNs via coordination of HDBB to Tb3+ prevents the torsional relaxation, thus the RIM process is activated. The fluorescence lifetime of Tb-HDBB-CPNs (2.92 ns) is longer than the one of HDBB molecules (1.97 ns) (Fig. S8†). After coordinating with Tb3+, the radiative rate constant (kr) of the HDBB increased from 27.0 × 106 s−1 to 48.86 × 106 s−1, but the non-radiative rate constant (knr) decreased from 4.80 × 108 s−1 to 2.94 × 108 s−1 (Table S1†).
These results are similar with previous reports, the lifetime and fluorescence quantum yield of many AIE molecules usually increase after aggregation owing to the reducing non-radiative processes and activating the radiative channel.19 Consequently, the formation of CPNs prevents the HDBB excited state distortion, hence the exciton immediately released the energy via fluorescence and results in a higher fluorescence quantum yield and longer lifetime.
Unlike Tb-HDBB-CPNs emit yellow fluorescence measured at 590 nm, the Zn-HDBB-CPNs emit blue lights observed at 470 nm, which is almost 120 nm blue-shifted (Fig. 2A). The maximum excitation wavelength of Zn-HDBB-CPNs is retained at 365 nm, which is consistent with its absorption spectrum. The lifetime and fluorescence quantum yield are 17.25% and 1.01 ns (Fig. S9†). The radiative rate constant and non-radiative rate constant of Zn-HDBB-CPNs were 172.5 × 106 s−1 and 8.19 × 10 s−1, respectively. This distinct emission can be ascribed to the inhibition of photo-induced electron transfer process after HDBB coordinating with Zn(II); two nitrogens on hydrazone moiety in HDBB molecule as well as phenolic oxygen may participate in binding to Zn2+.49,50
3.3 Sensing mechanism of ratiometric fluorescent probe for Zn2+
Cation exchange in CPNs and MOFs has become a powerful method tuning their composition, functionality and porosity.6,51,52 We hypothesized that cation exchange process also can occur in our AIE-based CPNs. In a typical reaction, we prepared a mixture of Tb-HDBB-CPNs and 0.15 mM Zn2+ in pH 7.0 Tris buffer solution under ambient conditions. After 3 h of cation exchange, the resulted CPNs were separated from the mixture by centrifugation. Fluorescence emission spectra, SEM, EDX spectra and ICP spectra were measured before and after cation exchange reaction of the CPNs sample. As shown in Fig. 3A, fluorescence emission centered at 470 nm increased remarkably with a decrease of fluorescence emission at 590 nm under the excitation wavelength at 365 nm. The dual emission spectrum of the resulting particles reveals successful cation exchange of Tb-HDBB-CPNs by Zn2+, consequently, blocking of photo-induced electron transfer process of HDBB molecules. Furthermore, the morphology of Tb-HDBB-CPNs after Zn2+ exchange is not much different from the one of before exchange (Fig. S10†). A relating EDX spectrum of the Tb-HDBB-CPNs measured after the cation exchange shows the expected Zn signal, meanwhile, Tb signal is also can be observed in the EDX spectrum (Fig. S11†). Finally, Tb signal observed in ICP spectrum of Tb-HDBB-CPNs supernatant after cation exchange is much larger than the one of control experiment without adding Zn2+, indicating that partly cation exchange of Tb-HDBB-CPNs by Zn2+. These experiments provided direct evidences of cation exchange in Tb-HDBB-CPNs with Zn2+. Thus, the cation exchange process of Tb-HDBB-CPNs is demonstrated as in Fig. 3B. Briefly, upon the addition of Zn2+, some carboxylic group on the surface of Tb-HDBB-CPNs bind to Zn2+ resulting in the formation Zn-Tb-HDBB-CPNs with dual emission fluorescent behavior. As a result, our strategy not only provides a facile route to introduce desired functionality into CPNs but also promotes the potential of CPNs for application, such as ratiometric fluorescence detection.
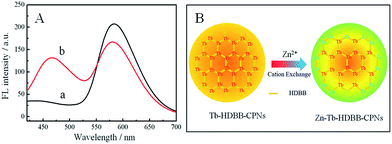 |
| Fig. 3 (A) Fluorescence emission spectra of 0.035 mg mL−1 Tb-HDBB-CPNs in the absence (curve a) and presence of 0.15 mM Zn2+ (curve b). (B) Schematic representation of the cation exchange process of Tb-HDBB-CPNs with Zn2+. | |
3.4 Optimization for Zn2+ ion detection
As is demonstrated above, the successful cation exchange process of Tb-HDBB-CPNs by Zn2+, inducing the increasing fluorescence at 470 nm and the decreasing emission at 590 nm, prompted us to apply this AIE-based CPNs for the detection of Zn2+ in aqueous solution. We can image that the ratios of I470 to I590 (i.e., I470/I590) would be closely associated with the degree of the exchange of the Tb-HDBB-CPNs caused by Zn2+, as a consequence, which could be used for the ratiometric fluorescence assay of Zn2+.
Fig. 4A depicts the typical time-dependent fluorescent intensity at 470 nm of the Tris buffer containing Tb-HDBB-CPNs after the addition of Zn2+ into the buffer. Upon the addition of Zn2+ the fluorescent spectrum of the Tb-HDBB-CPNs was recorded immediately and every on minute consecutively at room temperature. After adding Zn2+, the fluorescent intensity at 470 nm of Tb-HDBB-CPNs was enhanced significantly and reached a plateau after 2 min. The fast fluorescence response can be ascribed to the unique amorphous structure of Tb-HDBB-CPNs.
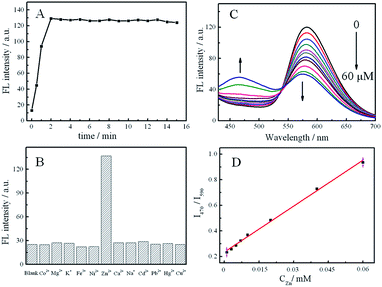 |
| Fig. 4 (A) Time-dependent fluorescence at 470 nm response of the 0.02 mg mL−1 Tb-HDBB-CPNs to 0.1 mM Zn2+ in pH 7.0 Tri buffer solution. (B) The selectivity of Tb-HDBB-CPNs based sensor for Zn2+ to other cations in pH 7.0 Tri buffer solution. The concentrations of Tb-HDBB-CPNs, Zn2+ and other cations were 0.02 mg mL−1, 0.15 mM and 0.25 mM, respectively. (C) Fluorescence emission spectra of Tb-HDBB-CPNs probe exposed to various concentrations of Zn2+. These spectra were measured in pH 7.0 Tri buffer solution. The concentration of Tb-HDBB-CPNs was 0.02 mg mL−1. The concentration of Zn2+ were 0, 0.1, 0.5, 1.0, 3.0, 5.0, 7.0, 10, 20, 40, 60 μM. (D) The linear plot of the value of I470/I590 versus the concentration of Zn2+. | |
We next examined the effects of other metal ions on the fluorescence intensity of Tb-HDBB-CPNs under identical conditions. As shown in Fig. 4B, only the addition of Zn2+ resulted in a significant fluorescence enhancement at 470 nm; no remarkable changes in the fluorescence of Tb-HDBB-CPNs were observed upon the addition of other metal ions, including Co2+, Mg2+, K+, Fe3+, Ni2+, Ca2+, Cd2+, Pb2+, Hg2+, and Cu2+. The high selectivity of Tb-HDBB-CPNs to Zn2+ is attributed to the special fluorescent behavior of the coordination compound of Zn2+ with HDBB.
The fluorescence response of Tb-HDBB-CPNs to Zn2+ in different concentrations is shown as Fig. 4C. Corresponding to the decreased fluorescence at 590 nm, the fluorescence at 470 nm was enhanced gradually with the increase of Zn2+ concentrations from 0 to 60 μM. There is a linear ratio fluorescence response to Zn2+ in the concentration range 0.1–60 μM. The detection limit is 50 nM on the basis of a signal-to-noise ratio of 3
:
1. This detection limit satisfies the maximum permitted level of 5 mg L−1 Zn2+ in drinking water regulated by the U.S. Environmental Protection Agency (EPA).45
3.5 Application in water samples
It is very important to monitor the level of Zn2+ in water samples, because Zn2+ in human bodies tend to bio-accumulate and damage central nervous system, blood composition, lungs, kidneys and liver. The practicability of Tb-HDBB-CPNs fluorescent nanoprobes was assessed by applying it to the real analysis for Zn2+ in water sample of the Green Lake, which is a famous scenic spot of Yunnan Province in China. An average value of 1.48 ± 0.18 μM Zn2+ was found for n = 4 determinations in the Green Lake water sample using this new approach with good recovery (99.15%), after the subtraction of the interception from that of the standard calibration curve (Fig. 4D), which is consistent with the result obtained by ICP spectroscopic methods, 1.34 ± 0.08 μM. This result suggests that our ratiometric fluorescence nanoprobe can be used for Zn2+ determination in water samples.
4. Conclusions
In summary, AIE-based CPNs have been developed by using AIE molecules as bridging ligands. Tb-HDBB-CPNs were further employed as ratiometric fluorescence probe to direct detection of Zn2+ in aqueous solution based on cation exchange effect. The ratio fluorescence of Zn-Tb-HDBB-CPNs was enhanced linearly with the Zn2+ concentration from 100 nM to 60 μM. The detection limit for Zn2+ in aqueous solution is 50 nM. The ratiometric fluorescent probe based on Tb-HDBB-CNPs exhibits the advantages of direct and fast detection procedure, excellent stability and selectivity. The proposed strategy might provide a new platform for the design and application of fluorescent probes based on AIE functionalized coordination polymers.
Acknowledgements
This work was financially supported by the National Natural Science Foundation of China (NSFC, 21405135, 21465025), the Project sponsored by SRF for Yunnan university (2013CK006) and Cultivation Program for Key Young Teachers of Yunnan University (XT412003).
Notes and references
- M. Oh and C. A. Mirkin, Nature, 2005, 438, 651–654 CrossRef CAS PubMed.
- Y. Liu, F. Boey, L. L. Lao, H. Zhang, X. Liu and Q. Zhang, Chem.–Asian J., 2011, 6, 1004–1006 CrossRef CAS PubMed.
- I. Imaz, M. Rubio-Martínez, L. García-Fernández, F. García, D. Ruiz-Molina, J. Hernando, V. Puntes and D. Maspoch, Chem. Commun., 2010, 46, 4737–4739 RSC.
- C. M. Hongliang Tan, Q. Li, L. Wang, F. Xu, S. Chen and A. Song, Analyst, 2014, 139, 5516–5522 RSC.
- K. E. deKrafft, Z. Xie, G. Cao, S. Tran, L. Ma, O. Z. Zhou and W. Lin, Angew. Chem., Int. Ed., 2009, 48, 9901–9904 CrossRef CAS PubMed.
- M. Oh and C. A. Mirkin, Angew. Chem., 2006, 118, 5618–5620 CrossRef.
- N. Lin, J. Li, Z. Lu, L. Bian, L. Zheng, Q. Cao and Z. Ding, Nanoscale, 2015, 7, 4971–4977 RSC.
- L. Xing, Y. Cao and S. Che, Chem. Commun., 2012, 48, 5995–5997 RSC.
- W. J. Rieter, K. M. Pott, K. M. Taylor and W. Lin, J. Am. Chem. Soc., 2008, 130, 11584–11585 CrossRef CAS PubMed.
- Y. Liao, L. He, J. Huang, J. Zhang, L. Zhuang, H. Shen and C.-Y. Su, ACS Appl. Mater. Interfaces, 2010, 2, 2333–2338 CAS.
- R. C. Huxford, K. E. Dekrafft, W. S. Boyle, D. Liu and W. Lin, Chem. Sci., 2012, 3, 198–204 RSC.
- F. Pu, E. Ju, J. Ren and X. Qu, Adv. Mater., 2014, 26, 1111–1117 CrossRef CAS PubMed.
- H. Tan, B. Liu and Y. Chen, ACS Nano, 2012, 6, 10505–10511 CrossRef CAS PubMed.
- H. Tan, L. Zhang, C. Ma, Y. Song, F. Xu, S. Chen and L. Wang, ACS Appl. Mater. Interfaces, 2013, 5, 11791–11796 CAS.
- J. Deng, P. Yu, Y. Wang and L. Mao, Anal. Chem., 2015, 87, 3080–3086 CrossRef CAS PubMed.
- R. Nishiyabu, N. Hashimoto, T. Cho, K. Watanabe, T. Yasunaga, A. Endo, K. Kaneko, T. Niidome, M. Murata and C. Adachi, J. Am. Chem. Soc., 2009, 131, 2151–2158 CrossRef CAS PubMed.
- K. Mizusawa, Y. Takaoka and I. Hamachi, J. Am. Chem. Soc., 2012, 134, 13386–13395 CrossRef CAS PubMed.
- K. Mizusawa, Y. Ishida, Y. Takaoka, M. Miyagawa, S. Tsukiji and I. Hamachi, J. Am. Chem. Soc., 2010, 132, 7291–7293 CrossRef CAS PubMed.
- Y. Hong, J. W. Lam and B. Z. Tang, Chem. Commun., 2009, 4332–4353 RSC.
- J. Mei, Y. Hong, J. W. Lam, A. Qin, Y. Tang and B. Z. Tang, Adv. Mater., 2014, 26, 5429–5479 CrossRef CAS PubMed.
- Z. Luo, X. Yuan, Y. Yu, Q. Zhang, D. T. Leong, J. Y. Lee and J. Xie, J. Am. Chem. Soc., 2012, 134, 16662–16670 CrossRef CAS PubMed.
- R. Hu, N. L. Leung and B. Z. Tang, Chem. Soc. Rev., 2014, 43, 4494–4562 RSC.
- N. B. Shustova, T.-C. Ong, A. F. Cozzolino, V. K. Michaelis, R. G. Griffin and M. Dincă, J. Am. Chem. Soc., 2012, 134, 15061–15070 CrossRef CAS PubMed.
- V. Vij, V. Bhalla and M. Kumar, ACS Appl. Mater. Interfaces, 2013, 5, 5373–5380 CAS.
- Y. Liu, C. Deng, L. Tang, A. Qin, R. Hu, J. Z. Sun and B. Z. Tang, J. Am. Chem. Soc., 2010, 133, 660–663 CrossRef PubMed.
- Y. Zhang, D. Li, Y. Li and J. Yu, Chem. Sci., 2014, 5, 2710–2716 RSC.
- L. Zhang, N. He and C. Lu, Anal. Chem., 2015, 87, 1351–1357 CrossRef CAS PubMed.
- J. Chen, Y. Wang, W. Li, H. Zhou, Y. Li and C. Yu, Anal. Chem., 2014, 86, 9866–9872 CrossRef CAS PubMed.
- D. G. Khandare, H. Joshi, M. Banerjee, M. S. Majik and A. Chatterjee, Anal. Chem., 2015, 87, 10871–10877 CrossRef CAS PubMed.
- R. T. Kwok, C. W. Leung, J. W. Lam and B. Z. Tang, Chem. Soc. Rev., 2015, 44, 4228–4238 RSC.
- J. Liang, B. Z. Tang and B. Liu, Chem. Soc. Rev., 2015, 44, 2798–2811 RSC.
- D. Ding, K. Li, B. Liu and B. Z. Tang, Acc. Chem. Res., 2013, 46, 2441–2453 CrossRef CAS PubMed.
- X. Zhang, X. Zhang, S. Wang, M. Liu, Y. Zhang, L. Tao and Y. Wei, ACS Appl. Mater. Interfaces, 2013, 5, 1943–1947 CAS.
- L. Chen, Y. Jiang, H. Nie, R. Hu, H. S. Kwok, F. Huang, A. Qin, Z. Zhao and B. Z. Tang, ACS Appl. Mater. Interfaces, 2014, 6, 17215–17225 CAS.
- D. Li, Y. Zhang, Z. Fan, J. Chen and J. Yu, Chem. Sci., 2015, 6, 6097–6101 RSC.
- N. B. Shustova, B. D. McCarthy and M. Dinca, J. Am. Chem. Soc., 2011, 133, 20126–20129 CrossRef CAS PubMed.
- Z. Wei, Z.-Y. Gu, R. K. Arvapally, Y.-P. Chen, R. N. McDougald Jr, J. F. Ivy, A. A. Yakovenko, D. Feng, M. A. Omary and H.-C. Zhou, J. Am. Chem. Soc., 2014, 136, 8269–8276 CrossRef CAS PubMed.
- Q. Zhang, J. Su, D. Feng, Z. Wei, X. Zou and H.-C. Zhou, J. Am. Chem. Soc., 2015, 137, 10064–10067 CrossRef CAS PubMed.
- Z. Hu, W. P. Lustig, J. Zhang, C. Zheng, H. Wang, S. J. Teat, Q. Gong, N. D. Rudd and J. Li, J. Am. Chem. Soc., 2015, 16209–16215 CrossRef CAS PubMed.
- X.-G. Liu, H. Wang, B. Chen, Y. Zou, Z.-G. Gu, Z. Zhao and L. Shen, Chem. Commun., 2015, 51, 1677–1680 RSC.
- L. Wang, W. Wang and Z. Xie, J. Mater. Chem. B, 2016, 4, 4263–4266 RSC.
- N. B. Shustova, A. F. Cozzolino, S. Reineke, M. Baldo and M. Dincă, J. Am. Chem. Soc., 2013, 135, 13326–13329 CrossRef CAS PubMed.
- A. M. Spokoyny, D. Kim, A. Sumrein and C. A. Mirkin, Chem. Soc. Rev., 2009, 38, 1218–1227 RSC.
- A. Takeda, M. Nakamura, H. Fujii and H. Tamano, Metallomics, 2013, 5, 417–423 RSC.
- P. Xu, S. Huang, Z. Wang and G. Lagos, Sci. Total Environ., 2006, 362, 50–55 CrossRef CAS PubMed.
- J. Du, M. Hu, J. Fan and X. Peng, Chem. Soc. Rev., 2012, 41, 4511–4535 RSC.
- N. Lin, X. Chen, S. Yan, H. Wang, Z. Lu, X. Xia, M. Liang, Y.-L. Wu, L. Zheng and Q. Cao, RSC Adv., 2016, 6, 25416–25419 RSC.
- C. McKinstry, E. J. Cussen, A. J. Fletcher, S. V. Patwardhan and J. Sefcik, Cryst. Growth Des., 2013, 13, 5481–5486 CAS.
- X. Cao, X. Zeng, L. Mu, Y. Chen, R. Wang, Y. Zhang, J. Zhang and G. Wei, Sens. Actuators, B, 2013, 177, 493–499 CrossRef CAS.
- D. Xie, Z. Ran, Z. Jin, X. Zhang and D. An, Dyes Pigm., 2013, 2013(96), 495–499 CrossRef.
- M. H. Lee, J. S. Kim and J. L. Sessler, Chem. Soc. Rev., 2015, 44, 4185–4191 RSC.
- Z. Wang and S. M. Cohen, Chem. Soc. Rev., 2009, 38, 1315–1329 RSC.
Footnote |
† Electronic supplementary information (ESI) available. See DOI: 10.1039/c6ra28551a |
|
This journal is © The Royal Society of Chemistry 2017 |