DOI:
10.1039/C6RA27031J
(Paper)
RSC Adv., 2017,
7, 2051-2057
A novel composite of W18O49 nanorods on reduced graphene oxide sheets based on in situ synthesis and catalytic performance for oxygen reduction reaction†
Received
19th November 2016
, Accepted 20th December 2016
First published on 12th January 2017
Abstract
A novel composite catalyst based on in situ synthesis of W1+49 nanorods on reduced graphene oxide sheets was successfully fabricated through a one-pot solvothermal route. The as-prepared W18O49–reduced graphene oxide (rGO) composite has been systematically characterized by scanning electron microscopy, transmission electron microscopy, X-ray diffraction, Raman microscopy, X-ray photoelectron spectra, and a rotating disk electrode. Compared to W18O49 nanorods or reduced graphene oxide alone, or their physical mixture, the composite catalyst exhibited unexpectedly high ORR activity with a more positive onset-potential (close to that of Pt/C) and a higher kinetic current density (JK), which is mainly attributed to the nanorod morphology, surface oxygen vacancies of W18O49, and strong coupling interaction between the W18O49 nanorods and reduced graphene oxide sheets. These results raise the possibility of developing low-cost and efficient ORR electrocatalysts.
1. Introduction
Non-precious metal oxides have been actively pursued as catalysts because of their low price, abundance, and environmental compatibility.1–4 Recently, a wide range of research has been carried out on various metal oxide catalysts such as SnO2,5 TiO2,6 Fe3O4,7 Co3O4,8 Mn3O4–CoO,9 CoO,10 W18O49.11 The surfaces of many metal oxides are characterized by a large amount of oxygen vacancies participating in numerous physical and chemical reactions,12,13 which leads to their important applications in many aspects, including catalysis, electronics, and solar energy technologies.14 Y. Gorlin and T. Jaramillo proved that the oxygen vacancies in crystalline MnOx affected its catalytic activity for ORR under alkaline conditions.15 Dai et al. reported that the catalytic activity of Co3O4/N-doped graphene hybrid electrocatalysts toward ORR in alkaline media was sensitive to the number and activity of surface-exposed Co3+ ions.16 Our group also confirmed the presence of abundant oxygen vacancies on the surface of W18O49, which were effectively catalyzed the reduction of triiodide (I3−) to iodide (I−), promoted the photoelectric conversion efficiency of dye-sensitized solar cells.17,18 However, the electrical conductivities of these oxides are poor and restrained its applications in electrocatalysis.19,20
Tungsten oxides WO3−x (x = 0–1) have been regarded as the most promising candidates for photocatalysts due to their strong adsorption within the solar spectrum.21,22 Among various tungsten oxides, W18O49, one interesting non-stoichiometric tungsten oxide with abundant oxygen vacancies on the surface, is attracting increasing interest because it can be easily fabricated and scaled up, making it suitable for practical applications.23–25 Ye et al. prepared ultrathin W18O49 nanowires and applied to the photochemical reduction of CO2.26 W18O49 nanorods synthesized by Guo et al. could effectively adsorb visible light and serve as a photocatalytic material.27 Zhou et al. acquired W18O49 with different morphology through controlling the concentration of WCl6 via wet-chemical method.17,18 Effective combination of metal oxide nanostructures with some conductive carbon materials is believed to be one good strategy to obtain superior electrocatalysts. As a single-layer, two-dimensional sheet of hexagonal carbon, graphene has become a popular catalyst support due to its excellent conductivity, high surface area and good chemical stability. Many graphene-based metal oxide catalysts with outstanding catalytic activities have been reported.28–30 To date, few research on the controllable assembly of W18O49 nanorods supported on reduced graphene oxide (rGO) sheets as ORR electrocatalysts has been reported. Such catalysts are attractive targets, due to the surface oxygen vacancies of W18O49 and the conductivity of graphene.
In this work, we demonstrate W18O49–rGO composite, which is a novel class of W18O49 nanorods supported on reduced graphene oxide sheets synthesized via solvothermal method. This in situ growth of W18O49 on rGO sheets did not disrupt the conductive network of graphene and could significantly decrease the contact resistance between W18O49 and rGO. The as-prepared W18O49–rGO composite has been systematically characterized. We found the covalent coupling of W18O49 nanorods with rGO, suggesting that strongly coupled hybrid materials offer a promising strategy for advanced electrocatalysts. The composite takes an advantage of the activity of W18O49 derived from surface oxygen vacancies and the strong coupling with rGO, showing more positive onset potential, higher current density, lower H2O2 yield, and greater electron transfer number for ORR in alkaline media than the physical mixture of W18O49 nanorods and rGO sheets. Moreover, W18O49–rGO possesses a better durability than the commercial Pt/C catalyst.
2. Experimental
2.1 Syntheses of W18O49–rGO composite and W18O49 nanorod
In a typical procedure for the synthesis of W18O49–rGO composite, 1 mg ml−1 graphene oxide (GO) ethanol solution was subjected to ultrasonic treatment (950 W, 1 h) and then centrifuged at 4000 rpm for 0.5 h. WCl6 (60 mg) was dissolved in 48 ml of ethanol to form a clear yellow solution under magnetic stirring, and 12 ml of 1 mg ml−1 GO ethanol solution was added to the solution. The mixture was magnetically stirred for 0.5 h, loaded into a Teflon-lined stainless steel autoclave, and heated at 180 °C for 24 h. After cooling to room temperature, the resulting solid product was collected by centrifugation, washed repeatedly with water and ethanol, and vacuum drying at 50 °C overnight.
The synthesis procedure of W18O49 nanorod was the same as that for preparing W18O49–rGO composite, except that GO ethanol solution was not added during synthesis and the amount of ethanol used was 60 ml.
2.2 Materials characterization
The morphology, structure, and composition of the samples were characterized by different analytic techniques. Scanning electron microscopy (SEM) images were measured with a FEI HITACHI S-4800 field emission scanning electron microscope. Transmission electron microscopy (TEM) images, high-resolution TEM (HRTEM) images, and selected-area electron diffraction (SAED) patterns were taken with a JEOJ-2010 TEM with an acceleration voltage of 200 kV. The X-ray powder diffraction (XRD) profiles were carried out on a Rigaku D/max-rA X-ray diffractometer with Cu Kα radiation (λ = 1.54178 Å). Raman spectra were acquired from a Senterra R200-L Raman microscope, using a diode laser with excitation at 532 nm. X-ray photoelectron spectra (XPS) were recorded on an ESCALab MKII X-ray photoelectron spectrometer using Mg Kr radiation exciting source.
2.3 Electrochemical tests
Electrochemical measurements were performed at room temperature using a rotating disk working electrode made of glassy carbon (5 mm in diameter) connected to a CHI 660C electrochemical workstation (Chenhua, Shanghai). 4 mg catalyst was redispersed in 1 ml mixted solvents containing deionized water, isopropanol, and Nafion (5%) solution (v/v/v = 2.5
:
1
:
0.06) to form a 4 mg ml−1 suspension and ultrasonicated for 1 h to form a homogeneous ink. 5 μL catalyst ink was dropped on the glassy carbon disk electrode, leading to catalyst loading of ∼100 μg cm−2 and dried at room temperature. Pt plate and Ag/AgCl (PINE, 4 M KCl) were used as counter and reference electrodes, respectively. The electrochemical experiments were conducted in 0.1 M KOH electrolyte saturated with O2 at a scan rate of 10 mV s−1. Rotating disk electrode (RDE) measurements were conducted at different rotating speeds from 400 rpm to 2500 rpm. Rotating ring disk electrode (RRDE) measurements were scanned cathodically at a rate of 10 mV s−1 with 1600 rpm.
3. Results and discussion
The structure and morphology of the composite materials are examined by SEM and TEM. SEM images of the films on GC electrode with the same amount of W18O49–rGO, W18O49, rGO, and physical mixture of W18O49 and rGO (denoted as mixed W18O49 + rGO) are shown in Fig. 1 and S1.† As shown in Fig. 1a and S1a,† the W18O49 film consists of 1D nanorods with a length of 100–600 nm and diameter of 15–30 nm. At the end of the nanorods, thick nanorods bundles (NRBs) were formed by short nanorods, indicating an obvious aggregation (red line). Compared with W18O49, W18O49–rGO sample is composed of nanorods with a few NRBs (Fig. 1b and S1b†). The length of nanorods is between 110–500 nm, which is slightly less than W18O49. The diameter of nanorods is equal to W18O49, and is about 50 nm for NRBs. Besides few NRBs, no aggregates emerge in W18O49–rGO composite, suggesting that the aggregation of W18O49 nanorods is effectively reduced by the addition of rGO. The rGO shows a typical sheet structure (Fig. 1c and S1c†). After ultrasonic mixing with W18O49 nanorods and rGO, the aggregation degree of W18O49 nanorods decreased slightly (Fig. 1d and S1d†).
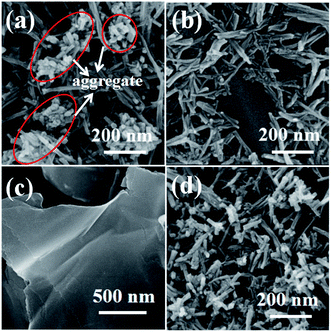 |
| Fig. 1 SEM images of (a) W18O49, (b) W18O49–rGO, (c) rGO, and (d) mixed W18O49 + rGO. | |
The TEM images of W18O49 (Fig. 2a and S2a†) show that these nanorods ranged from 100 nm to 600 nm with a width of about 20 nm. Cellular aggregation at the end of nanorods is observed, in accord with the SEM results. The HRTEM image shows an inter-planar spacing of 0.39 nm corresponding to the (010) plane of monoclinic W18O49 along the [010] direction (Fig. 2b). Among the tungsten oxides, W18O49 usually exhibits anisotropic growth along [010], thereby forming 1D nanostructures. This structure is beneficial in forming abundant active sites for oxygen adsorption, which can improve catalytic activity.31 The SAED pattern of nanorods (Fig. 2c) is consistent with monoclinic W18O49. With the existence of GO in solution, W18O49 grows along the graphene surface, and W18O49 nanorods with diameter of about 20 nm and a size range from 100 nm to 500 nm are obtained on graphene (Fig. 2d and S2b†). The HRTEM image of W18O49–rGO in Fig. 2e also displays an inter-planar spacing of 0.39 nm, corresponding to the (010) plane of monoclinic W18O49, which is consistent with the SAED pattern Fig. 2f. The growth of W18O49 prepared in the absence of GO sheets is similar to those prepared with GO sheets as template, both of which grow along the same direction.32 This phenomenon commonly occurs in one-dimensional W18O49 nanomaterials with monoclinic structure because their close-packed planes are {010}.33 Fig. 2e also discloses the lattice fringe of connected support with spacing of 0.34 nm, which corresponds well to the (001) plane of graphene.34 TEM images of graphene and mixed W18O49 + rGO are also presented in Fig. S2† for comparison. The EDX of W18O49–rGO composite shows that only C, O, and W can be detected from the TEM grid (Fig. S3†), eliminating other impure elements in the sample.
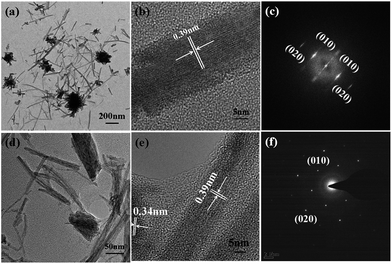 |
| Fig. 2 TEM, HRTEM, and the corresponding SAED pattern of (a–c) W18O49 nanorods and (d–f) W18O49–rGO composite. | |
The XRD patterns in Fig. 3a further confirm the formation of W18O49 nanorods and W18O49–rGO composite. All diffraction peaks of the XRD pattern of W18O49 and W18O49–rGO composite are in good agreement with that of monoclinic W18O49 (JCPDS 71-2540), proving the successful formation of W18O49.35 In the XRD pattern, two obvious diffraction peaks located approximately at 2θ values of 23.4° and 47.5° can be assigned to the reflection of the (010) and (020) planes, respectively, suggesting that W18O49 nanorods preferentially grow along the [010] direction. This result is in accordance with the above HRTEM analysis and SAED patterns. The XRD pattern of W18O49–rGO was identical to that of W18O49, except for the weak diffraction peak locate at 13.44°, which is associated with the (001) plane of rGO. These results indicates that W18O49 successfully grows on graphene sheets by a solvothermal strategy.
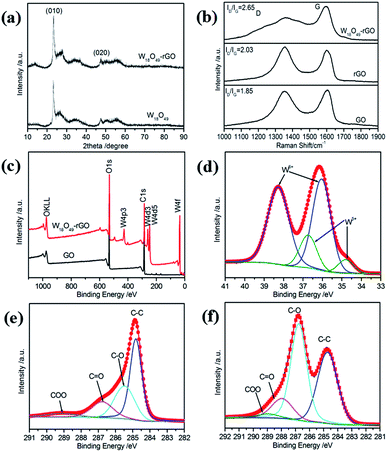 |
| Fig. 3 (a) XRD of W18O49–rGO composite catalyst. (b) Raman spectra and (c) XPS spectra of GO and W18O49–rGO composite; (d) high-resolution W 4f spectrum and corresponding deconvoluted spectra of W18O49–rGO composite; (e) and (f) C 1s core-level and corresponding deconvoluted spectra for W18O49–rGO composite and GO. | |
The structural features of GO sheets and W18O49–rGO composite have been detected using Raman spectroscopy (Fig. 3b). The Raman spectrum of GO displays two strong peaks at 1352 cm−1 and 1593 cm−1, which can be assigned to the D band (k-point phonons of A1g symmetry) and the G band (E2g phonon of C sp2 atoms), respectively.36 For W18O49–rGO composite, the two peaks is obviously shifted to about 1360 cm−1 and 1600 cm−1 due to the removal of oxygen-containing functional groups following reduction of GO sheets, suggesting an interaction between rGO and W18O49 nanorods. This interaction is conducive to promoting the stability of the catalyst interface, endowing the nanocomposites high stability. The Raman spectrum of W18O49–rGO also displays that the peak at 250 cm−1 can be assigned to the W–O–W bending vibrations and the peaks at 705 and 805 cm−1 can be assigned to the W–O–W stretching vibrations (Fig. S5†).37 Compared with GO, the ID/IG values increase from 1.85 to 2.03 for rGO and 2.65 for W18O49–rGO composite, suggesting that GO had been reduced after reaction.38 Furthermore, the ID/IG value of W18O49–rGO is higher than that of rGO, which indicates the lower overlapping degree of W18O49–rGO composite (as graphitic evolution).
The surface elemental composition of GO and W18O49–rGO composite is further assessed by XPS. The full-range XPS spectra of GO, rGO, mixed W18O49 + rGO, and W18O49–rGO composite are shown in Fig. 3c and S7a.† The survey XPS spectra for GO and rGO indicate the presence of oxygen and carbon. However, in the XPS spectra for mixed W18O49 + rGO and W18O49–rGO composite, tungsten can be detected according to the binding energies. Moreover, no impurities are detected. The deconvolution of the corresponding O 1s spectrum for W18O49–rGO composite (Fig. S6†) indicates that at least two chemical bonding states, O 2f and O 3f, are on the surface.17 The core-level XPS spectrum of W 4f can be deconvoluted into two doublets, which are associated with two different oxidation states of surface W atoms (Fig. 3d and Table 1). The first doublets consists of two peaks with binding energies of 36.1 and 38.4 eV, which belong to W 4f7/2 and W 4f5/2 of W6+ formal oxidation state, respectively. The second doublet, with lower binding energies of 34.9 and 36.8 eV, is derived from the emission of W 4f5/2 and W 4f7/2 core levels from five coordination tungsten atoms, thereby indicating the non-stoichiometric characteristics of the as-synthesized W18O49. The emergence of W5+ oxidation state shows the presence of oxygen vacancies of W18O49 nanorods, which is conducive to the adsorption of oxygen in the catalytic process. Compared with that of mixed W18O49 + rGO (Fig. S7b,† Table 1), the W 4f binding energy of W6+ of composite are increase from 38.1 eV to 38.4 eV and from 35.9 eV to 36.1 eV. The percentage of W5+ for W18O49–rGO composite is higher than that for mixed W18O49 + rGO, indicating that more oxygen vacancies are present on the surface of W18O49–rGO. The core-level XPS spectrum of C 1s and corresponding deconvoluted spectra for GO sheets are shown in Fig. 3e. The peak with binding energy centered at 284.7 eV can be attributed to the C–C bond. The deconvoluted peaks with binding energies of 286.8, 287.9, and 289.0 eV could be assigned to the C–O, C
O, and COOH bonds, respectively.39–41 The high-resolution C 1s XPS spectra for W18O49–rGO composite shown in Fig. 3f can be fitted with four different components peaks. Compared with GO, W18O49–rGO composite shows strong suppression for the oxygen-containing components of their C 1s XPS spectra, suggesting effective reduction of GO sheets via the present solvothermal technique. The presence of the binding energy profile at 285.4 eV (C–O bond) indicated a small amount of oxygen-containing carbon groups in the final W18O49–rGO composite, which is commonly found in chemically synthesized rGO (Fig. S7d†).42 Based on the deconvoluted XPS spectra of C 1s peak, the percentages and binding energy of different groups in GO, rGO, mixed W18O49 + rGO, and W18O49–rGO composite were shown in Table S1.† Compared with GO, after the formation of W18O49–rGO composite, the fraction of C–C bond increases from 32.9% to 54.7% and the fraction of C–O bond significantly decreases from 53.2% to 29.3%. Compared with the percentage of C–O of rGO and mixed W18O49 + rGO, that of W18O49–rGO composite is significantly higher because of the interaction between rGO and W18O49. Compared with rGO, the binding energy of different groups in W18O49–rGO composite have also increased in different degrees. The increase of W 4f and C 1s binding energy can facilitate the charge transfer between W18O49 and rGO. In addition, the C 1s binding energy of W18O49–rGO composite is higher compared with those of GO, rGO, and mixed W18O49 + rGO (Fig. S8†), indicating a covalent interaction between the rGO sheets and W18O49 nanorods.43 Therefore, a strong effect between W18O49 and rGO exists, which is beneficial to the adsorption and charge transfer of oxygen on the catalyst surface.
Table 1 The XPS fitting results of W 4f for mixed W18O49 + rGO and W18O49–rGO samples
Sample |
W 4f |
Position (eV) |
Percentage |
Mixed W18O49 + rGO |
W6+ |
38.1 |
40.1% |
35.9 |
48.4% |
W5+ |
34.9 |
3.5% |
36.8 |
8.0% |
W18O49–rGO |
W6+ |
38.4 |
41.2% |
36.1 |
40.6% |
W5+ |
34.9 |
5.0% |
36.8 |
13.2% |
Based on the above experimental results, the formation of the composite catalyst can be described as follows. First, large GO sheets with abundant oxygen-containing carbon groups on their basal planes and edges are negatively charged when dispersed in ethanol solution.44 W(VI) centers in the ethanol dissolution of WCl6 can bind with the oxygen-containing functional groups on GO sheets via electrostatic interactions. Upon heating at 180 °C, W18O49 nanorods growing along the [010] direction are formed through the hydrolysis of W(VI) centers on the GO sheets under reducing environment. In addition, the growth of W18O49 is accompanied with the reduction of GO to rGO during solvothermal process, leading to the formation of W18O49–rGO composite.
The ORR electrocatalytic activities of W18O49–rGO in O2-saturated 0.1 M KOH solution have been systematically investigated by cyclic voltammetry (CV), RDE and RRDE methods. Fig. S9† shows the CV curves of different samples in O2-saturated 0.1 M KOH solutions and all sample loadings are 100 μg cm−2. The ORR peak for W18O49–rGO composite is at 0.78 V with a highest current density of 1.36 mA cm−2 in all samples, indicating that O2 can be reduced much more easily on the W18O49–rGO composite than other samples.
The RDE linear sweep voltammetry (LSV) curves (Fig. 4a) shows that W18O49–rGO composite has an more positive onset potential. The half-wave potential (E1/2) of W18O49–rGO composite is 0.78 V, which is only 20 mV negative shift composite electrodes is significantly enhanced. The unique combination of W18O49 and rGO may play a role in improving the catalytic activity, and the synergetic effect of both components may contribute to enhanced ORR activity of the composite. Accordingly, in combination with the previous characterization, we speculate that W18O49 nanorod structures may have potential in providing more active sites for ORR, resulting in enhanced catalytic activity for ORR.
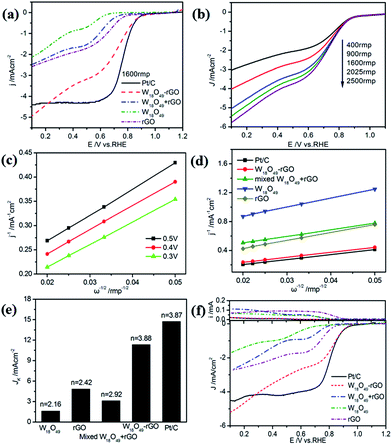 |
| Fig. 4 (a) LSV of W18O49, rGO, mixed W18O49 + rGO, W18O49–rGO, and Pt/C in O2-saturated 0.1 M KOH at a scan rate of 10 mV s−1 with an RDE rotation rate of 1600 rpm. (b) LSV of W18O49–rGO in O2-saturated 0.1 M KOH at different RDE rotation rates. (c) Calculated K–L plots of ORR from W18O49–rGO. (d) K–L plots of ORR from W18O49–rGO, W18O49 + rGO, W18O49, rGO, and Pt/C. (e) Kinetic limiting current density (JK) and electron transfer number (n) of W18O49, rGO, mixed W18O49 + rGO, W18O49–rGO, and Pt/C. (f) Ring current and disk current compared with Pt/C catalyst (0.80 V). Our results clearly demonstrate that ORR activity of W18O49 nanorods in the of W18O49, rGO, mixed W18O49 + rGO, W18O49–rGO, and Pt/C obtained from RRDE measurements. | |
To further explore the ORR behavior, the electron transport number n and kinetic current density JK have been investigated with RDE based on Koutecky–Levich (K–L) equations:44,45
|
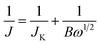 | (1) |
|
B = 0.2nF(DO2)2/3ν−1/6cO2
| (2) |
where
J is the experimental current density,
ω is the electrode rotating rate,
F is the Faraday constant (
F = 96
![[thin space (1/6-em)]](https://www.rsc.org/images/entities/char_2009.gif)
485 C mol
−1),
DO2 is the diffusion coefficient of O
2 (
DO2 = 1.9 × 10
−5 cm s
−1).
ν is the kinetic viscosity of the electrolyte (
ν = 0.1 cm
2 s
−1).
cO2 is the bulk concentration of O
2 (
cO2 = 1.2 × 10
−6 mol cm
−3). The constant 0.2 is adopted when the rotation speed is expressed in rpm. The LSV curves of W
18O
49–rGO composite at various rotating speeds are shown in
Fig. 4b. The K–L plots calculated from LSVs and compared at 0.4 V at various rotating speeds are shown in
Fig. 4c, d and S10.
† The electron transport number
n of W
18O
49–rGO composite shows the highest value of 3.88 at 0.4 V, similar to ORR catalyzed by commercial Pt/C (3.87), indicating that W
18O
49–rGO catalyst exhibits dominant four-electron oxygen reduction process.
JK value of 11.33 mA cm
−2 at 0.4 V for W
18O
49–rGO composite is also the largest among our four samples and close to that of Pt/C (14.74 mA cm
−2). This result has been suggested to proceed through simultaneous two-oxygen atom side adsorption for oxygen dissociation because of the oxygen vacancies on the surface of W
18O
49.
46 From the Tafel plots as shown in Fig. S11,
† W
18O
49–rGO catalyst occupied a much smaller Tafel slope at low over-potential than the other three catalysts, meaning excellent ORR activity. At the same time, we have optimized the ratio of rGO and W
18O
49 in composite catalyst and investigated the effect of catalytic activity for rGO content in composite catalyst (Fig. S12
†). It can be seen from the results, the catalytic activity is best when the 12 mg of GO is added. The RRDE measurements shows that the HO
2− yield of W
18O
49–rGO composite is less than 8%, significantly lower than rGO, W
18O
49 and W
18O
49 + rGO (
Fig. 4f and S13
†). The electron transfer number is calculated to be ≈ 3.8 over the potential range of 0.1–0.8 V. This is well consistent with the result obtained from the K–L plots based on RDE measurements. Based on these results, we speculate that oxygen molecule is reduced to HO
2− at W
18O
49 surface and further reduced to OH
− by rGO. Accelerated durability test results show that after 2000 cycles, the half-wave potential of W
18O
49–rGO is negatively shifted by 14 mV, compared to the 15 mV negative shift observed for the Pt/C catalyst (
Fig. 5a and b). Chronoamperometric (CA) response results show that W
18O
49–rGO exhibits excellent stability with very slow attenuation for 5 h, with 98.2% of its initial current still persisting (
Fig. 5c). This is much better than that of commercial Pt/C catalyst, which lost 23.4% current under the same conditions. The methanol crossover experiment shows that the negative cathodic ORR current of W
18O
49–rGO composite does not show a significant change after the scheduled sequential addition of 3 M methanol into the electrolyte solution, suggesting that its original ORR performance is unaffected by the addition of methanol (
Fig. 5d). The excellent long-term stability as well as high selectivity to ORR further suggest that W
18O
49–rGO composite is a very promising ORR electrocatalyst for direct methanol fuel cells.
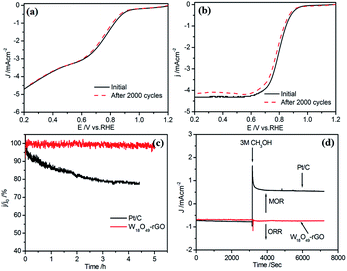 |
| Fig. 5 (a) W18O49–rGO and (b) Pt/C before and after 2000 potential cycles in O2-saturated 0.1 M KOH. The potential was cycled between 0.2 and 1.2 V at a rate of 50 mV s−1. (c) Chronoamperometric response of W18O49–rGO and Pt/C. (d) Chronoamperometric response of W18O49–rGO and Pt/C before and after addition of 3 M methanol. The tests were conducted in O2-saturated 0.1 M KOH solution at 0.70 V. | |
The excellent ORR performance of this non-precious composite material can be attributed to the nanorod morphology, surface oxygen vacancies of W18O49, and strong coupling between metal species and graphene. Specifically, the nanorod morphology can provide numerous surfaces with oxygen vacancies; the oxygen vacancies on the surface of W18O49 nanorods are greatly favourable to O2 adsorption and dissociation. Strong bonding between rGO and W18O49 can facilitate electron transfer, which is also a guarantee for the good durability. The (010) facets in W18O49 with edge-sharing W–O octahedral are proved to be favorable for catalytic processes because of the high oxidation valence and strong ability to form a redox couple.47 The prominent ORR catalytic activity of W18O49–rGO with (010) facet is probably related to the facile adsorption of oxygen on surface oxygen vacancies of (010) facets of W18O49 nanorods during the ORR process. As a result, the W18O49–rGO composite with highly exposed (010) facets displays high ORR performance, comparable to the commercial Pt/C.
4. Conclusions
In summary, we have successfully realized the in situ growth of W18O49 nanorods integrated with rGO sheets to prepare a new W18O49–rGO composite catalyst using a simple one-pot solvothermal route. This cheap and facilely prepared non-precious composite electrocatalyst possesses prominent ORR catalytic activity, which is comparable with commercial Pt/C in both reaction current density and onset potential. These advantages can be ascribed to the nanorod morphology, surface oxygen vacancies of W18O49, and strong covalent bonding between metal species and graphene. The W18O49–rGO composite catalyst also exhibited outstanding fuel crossover resistance and long-term durability in alkaline medium. The n value for ORR on the W18O49–rGO composite catalyst is 3.88, indicating that the dominant process is the favourable four-electron reduction pathway. This work suggests a great promise for designing effective ORR electrocatalysts by suitable electrical and chemical coupling of cheap functional materials, which is a potentially promising and suitable substitute for the expensive noble metal catalysts in the next generation alkaline and direct methanol fuel cells.
Acknowledgements
Financial support provided by the National Natural Science Foundation of China (Grant No. 51273032, 91333104, 51402036), the Natural Science Foundation of Education Department of Anhui (Grant No. KJ2016A171). Stable Talent Foundation of Anhui Science and Technology University. International Science & Technology Cooperation Program of China (Grant No. 2013DFA51000), the Fundamental Research Funds for the Central Universities (Grant No. DUT15YQ109). This research was also supported by the State Key Laboratory of Fine Chemicals of China.
Notes and references
- Y. Y. Liang, Y. G. Li, H. L. Wang, J. G. Zhou, J. Wang, T. Regier and H. J. Dai, Nat. Mater., 2011, 10, 780 CrossRef CAS PubMed.
- Z. S. Wu, S. Yang, Y. Su, K. Parvez, X. L. Feng and K. Müllen, J. Am. Chem. Soc., 2012, 134, 9082 CrossRef CAS PubMed.
- Y. F. Xu, M. R. Gao, Y. R. Zheng, J. Jiang and S. H. Yu, Angew. Chem., Int. Ed., 2013, 52, 8546 CrossRef CAS PubMed.
- M. R. Gao, Y. F. Xu, J. Jiang, Y. R. Zheng and S. H. Yu, J. Am. Chem. Soc., 2012, 134, 2930 CrossRef CAS PubMed.
- Y. F. Hu, J. Zhou, P. H. Yeh, Z. Li, T. Y. Wei and Z. L. Wang, Adv. Mater., 2010, 22, 3327 CrossRef CAS PubMed.
- K. Nakata and A. Fujishima, J. Photochem. Photobiol., C, 2012, 13, 169 CrossRef CAS.
- Y. X. Ye, L. Kuai and B. Y. Geng, J. Mater. Chem., 2012, 22, 19132 RSC.
- R. Jing, A. X. Shan, R. M. Wang and C. P. Chen, CrystEngComm, 2013, 15, 3597 RSC.
- J. W. Xiao, L. Wan, X. Wang, Q. Kuang, S. Dong, F. Xiao and S. Wang, J. Mater. Chem. A, 2014, 2, 3794 CAS.
- H. T. Yu, Y. C. Li, X. H. Li, L. Z. Fan and S. H. Yang, Chem.–Eur. J., 2014, 20, 3457 CrossRef CAS PubMed.
- H. W. Zhou, Y. T. Shi, L. Wang, H. Zhang, C. Y. Zhao, A. Hagfeldt and T. L. Ma, Chem. Commun., 2013, 49, 7626 RSC.
- R. Schaub, E. Wahlstrom, A. Ronnau, E. Laegsgaard, I. Stensgaard and F. Besenbacher, Science, 2003, 299, 377 CrossRef CAS PubMed.
- D. Matthey, J. G. Wang, S. Wendt, J. Matthiesen, R. Schaub, E. Lægsgaard, B. Hammer and F. Besenbacher, Science, 2007, 315, 1692 CrossRef CAS PubMed.
- H. Over, Chem. Rev., 2012, 112, 3356 CrossRef CAS PubMed.
- Y. Gorlin and T. Jaramillo, J. Am. Chem. Soc., 2010, 132, 13612 CrossRef CAS PubMed.
- Y. Y. Liang, Y. G. Li, H. L. Wang, J. G. Zhou, J. Wang, T. Regier and H. J. Dai, Nat. Mater., 2011, 10, 780 CrossRef CAS PubMed.
- H. W. Zhou, Y. T. Shi, L. Wang, H. Zhang, C. Y. Zhao, A. Hagfeldt and T. L. Ma, Chem. Commun., 2013, 49, 7626 RSC.
- H. W. Zhou, Y. T. Shi, Q. S. Dong, Y. X. Wang, C. Zhu, L. Wang, N. Wang, Y. Wei, S. Y. Tao and T. L. Ma, J. Mater. Chem. A, 2014, 2, 4347 CAS.
- G. Q. Zhang, B. Y. Xia, X. Wang and X. W. Lou, Adv. Mater., 2014, 26, 2408 CrossRef CAS PubMed.
- Y. Y. Liang, H. L. Wang, P. Diao, W. Chang, G. S. Hong, Y. G. Li, M. Gong, L. M. Xie, J. G. Zhou, J. Wang, T. Z. Regier, F. Wei and H. J. Dai, J. Am. Chem. Soc., 2012, 134, 15849 CrossRef CAS PubMed.
- X. H. Zhang, X. H. Lu, Y. Q. Chen, J. B. Han, L. Y. Yuan, L. Gong, Z. Xu, X. D. Bai, M. Wei, Y. X. Tong, Y. H. Gao, J. Chen, J. Zhou and Z. L. Wang, Chem. Commun., 2011, 47, 5804 RSC.
- Z. G. Zhao, Z. F. Liu and M. Miyauchi, Chem. Commun., 2010, 46, 3321 RSC.
- C. S. Guo, S. Yin, Q. Dong and T. Sato, RSC Adv., 2012, 2, 5041 RSC.
- S. B. Sun, Y. M. Zhao, Y. D. Xia, Z. D. Zou, G. H. Min and Y. Q. Zhu, Nanotechnology, 2008, 19, 305 Search PubMed.
- D. Wang, J. Li, X. Cao, G. S. Peng and S. H. Feng, Chem. Commun., 2010, 46, 7718 RSC.
- G. Xi, S. Ouyang, P. Li, J. Ye, Q. Ma, N. Su, H. Bai and C. Wang, Angew. Chem., Int. Ed., 2012, 51, 2395 CrossRef CAS PubMed.
- C. Guo, S. Yin, M. Yan, M. Kobayashi, M. Kukihana and T. Sato, Inorg. Chem., 2012, 51, 4763 CrossRef CAS PubMed.
- J. T. Zhang, C. X. Guo, L. Y. Zhang and C. M. Li, Chem. Commun., 2013, 49, 6334 RSC.
- Z. S. Wu, S. B. Yang, Y. Sun, K. Parvez, X. L. Feng and K. Mullen, J. Am. Chem. Soc., 2012, 134, 9082 CrossRef CAS PubMed.
- Y. Y. Liang, H. L. Wang, P. Diao, W. Chang, G. S. Hong, Y. G. Li, M. Gong, L. M. Xie, J. G. Zhou, J. Wang, T. Z. Regier, F. Wei and H. J. Dai, J. Am. Chem. Soc., 2012, 134, 15849 CrossRef CAS PubMed.
- J. Lee, G. Park, H. Lee, S. Kim, R. Cao, M. Liu and J. Cho, Nano Lett., 2011, 11, 5362 CrossRef CAS PubMed.
- X. T. Chang, L. H. Dong, Y. S. Yin and S. B. Sun, RSC Adv., 2013, 3, 15005 RSC.
- Y. Z. Jin, Y. Q. Zhu, R. L. D. Whitby, N. Yao, R. Z. Ma, P. C. P. Watts, H. W. Kroto and D. R. M. Walton, J. Phys. Chem. B, 2004, 108, 15572 CrossRef CAS.
- T. F. Yeh, J. M. Syu, C. Cheng, T. H. Chang and H. S. Teng, Adv. Funct. Mater., 2010, 20, 2255 CrossRef CAS.
- H. W. Zhou, Y. T. Shi, Q. S. Dong, Y. X. Wang, C. Zhu, L. Wang, N. Wang, Y. Wei, S. Y. Tao and T. L. Ma, J. Mater. Chem. A, 2014, 2, 4347 CAS.
- H. J. Song, X. H. Jia, N. Li, X. F. Yang and H. Tang, J. Mater. Chem., 2012, 22, 895 RSC.
- F. Xu, A. Fahmi, Y. M. Zhao, Y. D. Xia and Y. Q. Zhu, Nanoscale, 2012, 4, 7031 RSC.
- X. G. Bai, Y. T. Shi, H. J. Guo, L. G. Gao, K. Wang, Y. Du and T. L. Ma, J. Power Sources, 2016, 306, 85–91 CrossRef CAS.
- J. P. Zhao, S. F. Pei, W. C. Ren, L. B. Gao and H. M. Cheng, ACS Nano, 2010, 4, 5245 CrossRef CAS PubMed.
- H. X. Tang, G. J. Ehlert, Y. R. Lin and H. A. Sodano, Nano Lett., 2012, 12, 84 CrossRef CAS PubMed.
- Z. X. Jiang, J. J. Wang, L. H. Meng, Y. D. Huang and L. Liu, Chem. Commun., 2011, 47, 6350 RSC.
- S. Stankovich, D. A. Dikin, R. D. Piner, K. A. Kohlhaas, A. Kleinhammes, Y. Jia, Y. Wu, S. T. Nguyen and R. S. Ruoff, Carbon, 2007, 45, 1558 CrossRef CAS.
- D. Joung, V. Singh, S. Park, A. Schulte, S. Seal and S. I. Khondaker, J. Phys. Chem. C, 2011, 115, 24494 CAS.
- S. Wang, D. Yu and L. Dai, J. Am. Chem. Soc., 2011, 133, 5182 CrossRef CAS PubMed.
- L. Y. Zhang, C. X. Guo, Z. Cui, J. Guo, Z. Dong and C. M. Li, Chem.–Eur. J., 2012, 18, 15693 CrossRef CAS PubMed.
- S. Kattel, P. Atanassov and B. Kiefer, Phys. Chem. Chem. Phys., 2013, 15, 148 RSC.
- H. Huang, Q. Yu, X. Peng and Z. Ye, Chem. Commun., 2011, 47, 12831 RSC.
Footnote |
† Electronic supplementary information (ESI) available: Low-magnification SEM and TEM images of four catalyst; EDX spectrum of W18O49–rGO; O 1s core-level of W18O49–rGO; XPS spectra; CVs; LSVs; chronoamperometric response; XPS fitting results, percentages of different bindings of C 1s peak. See DOI: 10.1039/c6ra27031j |
|
This journal is © The Royal Society of Chemistry 2017 |