DOI:
10.1039/C6QM00307A
(Research Article)
Mater. Chem. Front., 2017,
1, 1051-1058
A powerful “one-pot” tool for fabrication of AIE-active luminescent organic nanoparticles through the combination of RAFT polymerization and multicomponent reactions†
Received
9th November 2016
, Accepted 6th December 2016
First published on 7th December 2016
Abstract
Multicomponent reactions (MCRs) have recently received increasing attention for the synthesis of structural complexity in a single step from three or more reactants. They have also been considered as a powerful tool for the construction of sequence-controlled multifunctional polymers owing to their good substrate adaptability, simple operation and high efficiency. In this work, we reported methods that are a combination of the three-component mercaptoacetic acid locking imine (MALI) reaction and reversible addition fragmentation chain transfer (RAFT) polymerization in one pot to form luminescent organic nanoparticles (LONs) with aggregation-induced emission (AIE) features, high-brightness, great water dispersibility, ultra-small nanoscale size and excellent biocompatibility. In the reaction system, the MALI reaction and RAFT polymerization happened simultaneously in a “one-pot” route. On the one hand, the AIE-active organic dye with one amino group ((Z)-3-(4-aminophenyl)-2-(10-hexadecyl-10H-phenothiazin-3-yl)acrylonitrile) (named as Phe-NH2) was conjugated with an aldehyde-containing monomer (10-undecenal) by the MALI reaction, while the aldehyde-containing monomer was copolymerized with the hydrophilic monomer polyethylene glycol methyl methacrylate (PEGMA) through RAFT polymerization at the same time. Compared with other fabrication strategies, “one-pot” strategies possess some advantages such as high efficiency, simplicity, and atom economy. On the other hand, due to the good applicability of RAFT polymerization and the MALI reaction, many other multifunctional AIE-active LONs could also be fabricated via adjusting the function of the substrates. Therefore, this strategy should be a general and important route for fabrication of AIE-active materials for different applications.
1. Introduction
Accompanying an increasing demand for functional polymers in a range of interdisciplinary applications, functional polymers with readily clickable groups have attracted growing research interest because of their facile and robust ability to produce versatile functional materials.1–4 In order to expand the scope of functional polymer materials produced from post-polymerization modification, many novel methods with clickable properties need to be developed and utilized. Multicomponent reactions (MCRs) could serve as similar “click reactions” to produce highly selective products because of their outstanding properties such as high yield, generation of less waste, minimization of superabundant handling and atom efficiency. In general, MCRs are usually defined as reactions in which multiple reactants (usually constrained to three or more components) are reacted in one pot to form a product that contains structural features of each reagent. Since the first discovery of isonitrile-based MCRs by Passerini in 1921, some famous MCRs such as the Passerini reaction,5 Biginelli reaction,6 Hantzsch reaction,7 Ugi reaction8 and MALI reaction9 have been intensively investigated. These MCRs have played important roles in drug synthesis, polymer chemistry and material science. For example, Meier's group adopted the Ugi reaction and other MCRs to prepare functional polymer materials.10–12 Tao et al. reported a facile strategy to obtain polymer modified CNT composites via a three-component Biginelli reaction.13 Although these older reactions have been deeply researched for modern applications, some disadvantages such as a single group's reaction and low molecular weight of the prepared polymers have been gradually perceived. Therefore, exploring a new direction for MCRs to perfect these drawbacks and enrich their potential application is extremely important.
Integration of MCRs with the traditional polymerization strategies such as atom transfer radical polymerization (ATRP) and reversible addition fragmentation chain transfer (RAFT) polymerization provides a means to extend the application of MCRs in polymer chemistry.14–16 As is well-known, ATRP and RAFT polymerization are effective methods to prepare alignment-control functional polymers with adjustable molecular weight, which fills the gaps of older MCRs. Inspired by the previous reports, much effort has been devoted from researchers to combine a series of reactants in one synthetic procedure for preparing products with complex structures. For instance, Haddleton and co-workers have reported combination of the copper-catalyzed “click” reaction and ATRP to prepare glycopolymers in a one pot procedure.17 Tao and co-workers also demonstrated an efficient synthesis of well-defined poly(1,4-dihydropyridine) by the simultaneous Hantzsch reaction and RAFT polymerization.18 Based on the previous efforts in the combination of MCRs and conventional polymerization methods, novel functional polymer materials with unique features such as fluorescence, responsiveness and biocompatibility could be easily synthesized to satisfy the needs of people for functional materials. Among them, fluorescent polymer nanoprobes have still received enthusiastic pursuits from medical scientists due to their promising potential applications such as cell imaging, targeting, tracing and therapeutics.19–26 Previously, luminescent organic nanoparticles (LONs) have mainly been focused on inorganic quantum dots,27–30 Ln ion doped nanomaterials31,32 and metallic nanoclusters33,34 due to their excellent photostability with narrow emission, subnanometer size and up-conversion performance; however, their potential toxicity and poor biodegradability largely hinder their employment in biomedical fields.35–40 While organic dyes were also regarded as ideal fluorescent agents to construct LONs to replace inorganic emitters because of their desirable biodegradability and low toxicity, the vicious aggregation caused quenching (ACQ) effect of organic fluorogens has become a fatal drawback for their biomedical applications.41,42 Therefore, scientists were seeking ideal fluorogens to fabricate LONs until the discovery of aggregation-induced emission (AIE) dyes by Tang et al. in 2001.43 AIE dyes are a novel type of fluorogen that are just the opposite to conventional organic dyes with the ACQ effect. AIE-active dyes emit strong fluorescence in an aggregated state or high concentration while weak or even no fluorescence in good solvents. Excepting ultra-strong fluorescence, AIE dyes also possess low toxicity, great biocompatibility and biodegradability. Based on these outstanding features, the fabrication and biomedical applications of AIE-active LONs have become an interesting research theme in recent years and a number of fabrication strategies have also been developed to fabricate AIE-active LONs. For example, Liu and Tang's group cooperatively reported the construction of AIE-active LONs using commercial and synthetic amphiphilic polymers as protected shells via non-covalent encapsulation, which have promising application potential in targeting imaging.44–50 Our group also reported a series of covalent fabrication methodologies that contained Schiff base condensation, RAFT polymerization, ring-opening polymerization and formation of dynamic bonds for the preparation of AIE-active LONs in recent years. These AIE-active polymeric structures have been extensively applied in cell imaging and imaging guided drug delivery.51–60 Although many contributions have been made to the fabrication methodologies of AIE-active LONs, novel fabrication methods with environmentally friendly, high-efficiency, atom economy and low cost features are still a highly urgent need in the preparation of AIE-active LONs.
In this contribution, we reported a facile method for the high efficiency synthesis of AIE-active LONs via the combination of MCRs (MALI reaction) and RAFT polymerization in a one-pot operation (Scheme 1). The three component MALI reaction is an important strategy to synthesize 4-thiazolidinones which have special functions in clinical treatments such as anti-HIV, anti-tuberculotic and anticonvulsant treatments.61,62 In this MCR, mercaptoacetic acid (MA) acts like a “lock” to covalently conjugate amine and aldehyde substrates under environmentally friendly conditions (catalyst free and room temperature) with water as the only byproduct. Therefore, the amino-containing AIE fluorogen (Phe-NH2) with orange light emission and an aldehyde-containing monomer (10-undecenal, UCL) could be locked together using MA molecules; meanwhile, PEGMA and UCL serve as polymerization monomers for the further preparation of functional polymers with strong luminescence by RAFT polymerization (Scheme 2). The resulting fluorescent amphiphilic polymer with the AIE fluorogen as a pendant would self-assemble into nanoparticles in aqueous solution due to its amphiphilic properties. These obtained fluorescent nanoparticles possess some outstanding properties such as intensive emission, ultra-small size, great water dispersibility, excellent biocompatibility and biodegradability. We expect that these LONs could serve as ideal candidates for further biomedical applications such as cell imaging, drug delivery, targeting, tracing and therapy due to their desirable features.
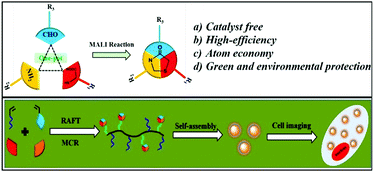 |
| Scheme 1 Schematic demonstrating the synthesis of fluorescent polymers via the combination of MCR and RAFT polymerization. The synthetic luminescent copolymers were self-assembled into LONs in aqueous solution and utilized for further cell imaging applications. The advantages of MCR are also listed in this picture. | |
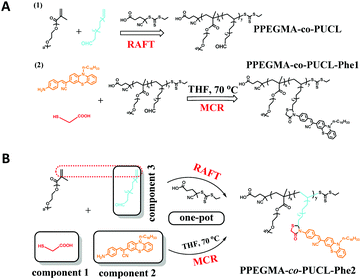 |
| Scheme 2 Schematic representing the synthetic methods of gradual and one-pot processes. (A) Gradual preparation of a fluorescent polymer (PPEGMA-co-PUCL-Phe1); (B) one-pot preparation of a fluorescent polymer (PPEGMA-co-PUCL-Phe2). | |
2. Experiment
2.1 Measurements and materials
All of the reagents and solvents were purchased from commercial sources and used directly without further purification, and distilled water was used in the experimental process. 10-Undecenal (97%, Mw: 168.28 Da), 1-bromohexadecane (97%, Mw: 305.34 Da), phenothiazine (98%, Mw: 199.27 Da), 4-aminobenzyl cyanide (97%, Mw: 132.16 Da), tetrabutylammonium hydroxide (30%, Mw: 259.47 Da), mercaptoacetic acid (98%, Mw: 92.12 Da) and polyethylene glycol methyl acrylate (98%, Mw: 950 Da) were obtained from the Aladdin company. 2-Mercaptoethanol and 4,4′-azobis(4-cyanovaleic acid) were also obtained from the Aladdin company to synthesize the chain transfer agent for RAFT. 1H nuclear magnetic resonance (NMR) spectra were recorded on a Bruker Avance-400 spectrometer with D2O and CDCl3 as the solvents. The synthetic materials were characterized by Fourier transform infrared spectroscopy (FT-IR) using KBr pellets. The FT-IR spectra were obtained from a Nicolet5700 (Thermo Nicolet corporation). Transmission electron microscopy (TEM) images were recorded on a Hitachi 7650B microscope which was operated at 80 kV. The TEM specimens were obtained by putting a drop of the nanoparticle ethanol suspension on a carbon-coated copper grid. The fluorescence data were obtained from a fluorescence spectrophotometer (FSP, model: C11367-11), which was purchased from Hamamatsu (Japan). Gel permeation chromatography (GPC) analyses of the polymers were performed using N,N-dimethyl formamide (DMF) containing 50 mM LiBr as the eluent. The GPC system was a Shimadzu LC-20AD pump system consisting of an auto injector, a MZ-Gel SDplus 10.0 μm guard column (50 × 8.0 mm, 102 Å) followed by a MZ-Gel SDplus 5.0 μm bead-size column (50–106 Å, linear) and a Shimadzu RID-10A refractive index detector. The system was calibrated with narrow molecular weight distribution polystyrene standards ranging from 200 to 106 g mol−1.
2.2 Preparation of PPEGMA-co-PUCL
The aldehyde-containing hydrophilic polymers (PEGMA-co-UCL) were synthesized using PEGMA and UCL as monomers via RAFT polymerization. The chain transfer agent (CTA) of the RAFT polymerization was synthesized according to the method previously reported.63 The monomers PEGMA (950 mg, 1 mmol) and 10-undecenal (UCL, 168 mg, 1 mmol) were dissolved in 1,4-dioxane solvent (10 mL) and this solution was put into a Schlenk tube. After that, CTA (26.3 mg, 0.1 mmol) and AIBN (8.2 mg, 0.05 mmol) were dissolved in 5 mL 1,4-dioxane and added to the Schlenk tube, which was sealed and deoxidized for 30 min and filled with N2. The reaction was carried out for 24 h, and the solution was then removed by facile rotary evaporation. The obtained crude product was purified via dialysis for three days and drenched in ethanol solution for 8 h. The resulting primrose polymers were obtained after freeze drying for one night.
2.3 Gradual synthesis of PPEGMA-co-PUCL-Phe1
In order to evaluate the advantages of the one-pot synthesis of the AIE-active fluorescent polymer (PPEGMA-co-PUCL & Phe-NH2-1), the gradual preparation of PPEGMA-co-PUCL & Phe-NH2-1 was carried out based on the following procedure. First, the amino-containing AIE dye (Phe-NH2) was synthesized according to our previous work.64PPEGMA-co-PUCL (200 mg) and Phe-NH2 (0.2 mmol, 110 mg) were mixed in dry THF (5 mL) and stirred for about 0.5 h at room temperature. Mercaptoacetic acid (MA, 27.6 mg) was subsequently added to the THF solution and reacted for 2 h. At the end of the reaction, glacial diethyl ether (0.3 mmol, 20 mL) was used to precipitate the fluorescent copolymers. These obtained copolymers were washed 5 times via dissolving circularly in THF and precipitating with diethyl ether. The final orange solid was dried under vacuum for one night.
2.4 One-pot synthesis of PPEGMA-co-PUCL-Phe2
The one-pot synthesis of AIE-active fluorescent copolymers exhibited some advantages such as saving time, convenient operation and a simple purification step compared with the gradual synthesis. In this work, the combination of the MALI reaction and RAFT polymerization to fabricate LONs was simple and had high efficiency. All of the reactants, Phe-NH2 (0.2 mmol, 110 mg), MA (0.3 mmol, 27.6 mg), UCL (0.2 mmol, 33.8 mg), PEGMA (0.3 mmol, 285 mg), CTA (0.03, 8 mg) and AIBN (0.015 mmol, 2.5 mg), were dissolved in dry THF solvent (20 mL) and put into a Schlenk tube. This Schlenk tube was sealed and filled with N2. The reaction was carried out at 70 °C for 24 h. After finishing, the fluorescent copolymers could be obtained via constantly precipitating with glacial diethyl ether and washing with THF 5 times. The orange copolymers were dried under vacuum for further characterization. After that, the synthetic PPEGMA-co-PUCL-Phe2 copolymer was further used to prepare fluorescent polymeric nanoparticles by self-assembly of this amphiphilic copolymer. The concrete process for the preparation of PPEGMA-co-PUCL-Phe2 LONs could be described as follows: synthetic amphiphilic PPEGMA-co-PUCL-Phe2 copolymer (200 mg) was dissolved in THF (10 mL) and underwent ultrasonic treatment for 30 min, followed by addition of distilled water (50 mL). The THF solvent was removed via facile rotary evaporation and the obtained PPEGMA-co-PUCL-Phe2 water solution underwent freeze drying treatment for 48 h. The PPEGMA-co-PUCL-Phe2 LONs with diameters of 100–150 nm could be obtained.
2.5 Cytotoxicity evaluation of the PPEGMA-co-PUCL-Phe2 LONs
In order to evaluate the biomedical application potential of the PPEGMA-co-PUCL-Phe2 fluorescent copolymers, the cell viability of HeLa cells with the PPEGMA-co-PUCL-Phe2 LONs was evaluated using a cell counting kit-8 (CCK-8) assay. The cells were put into 96-well microplates at a density of 5 × 104 cells mL−1 in 160 μL of a medium containing 10% fetal bovine serum (FBS). After 24 h of cell attachment, different concentrations of FNPs (10–120 μg mL−1) were incubated with the cells for 12 and 24 h. Then, the nanoparticles were removed and the cells were washed with phosphate buffer solution (PBS) three times. 10 μL of CCK-8 dye and 100 μL of Dulbecco's modified Eagle’s medium (DMEM) for cell culture were added to each well and incubated for another 3 h. Afterward, the plates were analyzed using a microplate reader (VictorIII, Perkin-Elmer). Measurements of formazan dye absorbance were carried out at 450 nm, with the reference wavelength being 620 nm. The obtained values were proportional to the number of live cells. The percent reduction of CCK-8 dye was compared to controls, which represented 100% CCK-8 reduction. Three replicate wells were used per microplate, and the experiment was carried out three times. Cell survival was expressed as absorbance relative to that of the untreated controls. The results are presented as mean ± standard deviation (SD).
2.6 Confocal microscopic imaging of the PPEGMA-co-PUCL-Phe2 LONs
The cell uptake behavior of the PPEGMA-co-PUCL-Phe2 LONs was analyzed to confirm their biomedical application potential. HeLa cells were cultured in DMEM supplemented with 10% heat-inactivated fetal bovine serum (FBS), 2 mM glutamine, 100 U mL−1 penicillin, and 100 μg mL−1 of streptomycin. The cell culture was controlled under humidified conditions of 95% air and 5% CO2 in DMEM culture medium at 37 °C. To maintain the exponential growth of cells, the culture medium should be refreshed every three days. Before treatment, the cells were seeded in a glass bottomed dish with a density of 1 × 105 cells per dish. On the day of treatment, the cells were incubated with PPEGMA-co-PUCL-Phe-2 LONs at a final concentration of 20 μg mL−1 for 3 h at 37 °C. Afterward, the cells were washed three times with PBS to remove the PPEGMA-co-PUCL-Phe2 LONs and then fixed with 4% paraformaldehyde for 10 min at room temperature. Cell images were obtained using a Zeiss 710 3-channel confocal laser scanning microscope (CLSM) (Zeiss, Germany) with an excitation wavelength of 458 nm.
3. Results and discussion
3.1 Characterization of the PPEGMA-co-PUCL-Phe2 LONs
Fluorescent bioprobes are useful tools to monitor the biological activity within sophisticated intracellular systems due to their high sensitivity, low toxicity, strong fluorescence and desirable biocompatibility, and have been successfully used as a powerful means of disease diagnosis, tracking and treatment. Therefore, exploration of new methods to fabricate fluorescent probes is very important. In this work, the combination of the MALI reaction and RAFT polymerization to construct AIE-active fluorescent bioprobes has been reported, owing to its obvious advantages such as short reaction time, easy operation and atom economy. Furthermore, AIE dye based LONs possess enhanced emission, great biodegradability and biocompatibility, making them very attractive for biomedical applications. Many characterization techniques such as TEM, 1H NMR, FT-IR, UV-Vis and fluorescence spectroscopy were used to confirm the successful fabrication of LONs using this method. As shown in Fig. 1, the 1H NMR spectra of these polymer samples demonstrate the successful preparation of LONs via the combination of MCR and RAFT polymerization. Fig. 1A is the spectrum of the intermediate polymer (PPEGMA-co-PUCL); the main chemical shifts of this polymer are labeled in this picture, and the peak at 9.6 ppm is ascribed to the formyl group (Fig. 1B), which could evidence the successful preparation of PPEGMA-co-PUCL. In order to demonstrate that the structure of the final fluorescent polymers is very similar between those obtained from the one-pot synthesis and the gradual synthesis, the 1H NMR spectra of PPEGMA-co-PUCL-Phe1 and PPEGMA-co-PUCL-Phe2 are provided in Fig. 1C and E. The structure of the luminescent polymers (PPEGMA-co-PUCL-Phe1 and PPEGMA-co-PUCL-Phe2) was also confirmed by 1H NMR spectra (Fig. 1C and E). Compared with the spectrum of PPEGMA-co-PUCL, the peak at 9.6 ppm has vanished, which could be explained by the successful conjugation between the formyl and amino groups using MA as the “lock”. On the other hand, the multiplet peak from 2.5 to 3.0 ppm first appeared in the two luminescent polymers. This signal is attributed to the methylene of the heterocycle. Furthermore, as shown in Fig. 1D and F, some new peaks were found from 6.0 to 8.0 ppm in these luminescent polymers. These peaks indicated that aromatic rings were introduced in these polymers. In other words, we could confirm that Phe-NH2 has successfully conjugated with PUCL, which could be copolymerized with PEGMA through RAFT polymerization. Furthermore, from the 1H NMR spectrum of PPEGMA-co-PUCL-Phe2, we can calculate that the monomer conversions of PEGMA and UCL are respectively about 60% and 51% by evaluation of the area ratio of specific H atoms. The degree of functionalization of Phe-NH2 in the copolymer is about 43%. The molecular weight of the resulting PPEGMA-co-PUCL-Phe2 is about 6470 Da, which is calculated from the NMR spectrum. On the other hand, the precise molecular weight of PPEGMA-co-PUCL-Phe2 as detected from the GPC result is 8850 Da, and the polydispersity index (PDI) is 1.24. The molecular weight distribution curve of PPEGMA-co-PUCL-Phe2 is also given in the ESI.† Therefore, we conclude that the formation of AIE-active fluorescent polymer has occurred via the combination of MCR and RAFT polymerization. Compared with the gradual synthesis, the one-pot process exhibits many advantages such as atom economy, simple operation and shortened reaction time. These features make the one-pot procedure more suitable for the fabrication of AIE-active copolymers.
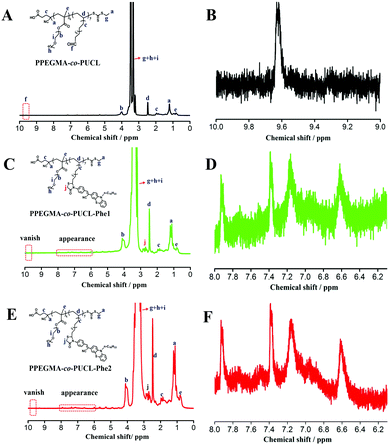 |
| Fig. 1
1H NMR spectra of different polymers: (A) PPEGMA-co-PUCL, (B) amplified region of the PPEGMA-co-PUCL spectrum ranging from 9.0 to 10.0 ppm, (C) PPEGMA-co-PUCL-Phe1 and (D) the amplified region ranging from 6.0 to 8.0 ppm, and (E) PPEGMA-co-PUCL-Phe2 and (F) the amplified region ranging from 6.0 to 8.0 ppm. | |
The successful preparation of luminescent amphiphilic polymers with strong orange emission could also be confirmed by FT-IR characterization. As shown in the IR spectrum of PPEGMA-co-PUCL (Fig. 2A), the peaks at 3599 and 3503 cm−1 are attributed to the –OH groups of the synthetic polymer, the peak at 3599 cm−1 is ascribed to the formation of intramolecular hydrogen bonds for –OH groups, and the peak at 3503 cm−1 is ascribed to the formation of intermolecular hydrogen bonds for –OH groups. On the other hand, due to the introduction of the –CHO group, the peak that appeared at 1637 cm−1 could be identified as the stretching vibration of –CHO. The above results provided solid evidence of the successful preparation of aldehyde-containing polymers. The IR spectra of PPEGMA-co-PUCL-Phe1 and PPEGMA-co-PUCL-Phe2 samples are shown in Fig. 2B. From the two curves, we can notice that there is a similar shape of the two different sample curves. Therefore, we can preliminarily consider that these two synthetic fluorescent polymers via the gradual and one-pot operations possess the same structure. In addition, comparing with the IR spectrum of the PPEGMA-co-PUCL polymer, a series of new peaks are apparent in the spectra of these fluorescent polymers. For example, the new peak at 2230 cm−1 could be regarded as the stretching vibration of the –CN groups that existed in the Phe-NH2 dye. On the other hand, there is a series of peaks that appeared between 640–980 cm−1, which could be considered as the fingerprint region of the aromatic rings. Finally, two obvious peaks at 1810 and 1345 cm−1 have emerged for the fluorescent polymers. These two peaks can be ascribed to the stretching vibration of the C(N)
O and C–N bonds, which were formed by the mercaptoacetic acid “lock” amine reaction. Therefore, we can conclude that there was a prosperous fabrication of LONs via the combination of MCR and RAFT polymerization in a mild procedure.
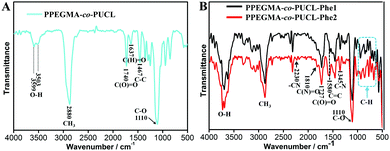 |
| Fig. 2 FT-IR spectra of PPEGMA-co-PUCL, PPEGMA-co-PUCL-Phe1 and PPEGMA-co-PUCL-Phe2. (A) IR spectrum of the PPEGMA-co-PUCL polymer; the peaks located at 1740 and 1637 cm−1 are attributed to the C O groups of C(O) O and CHO respectively, suggesting successful preparation of the aldehyde-containing polymer. (B) The IR spectra of PPEGMA-co-PUCL-Phe1 and PPEGMA-co-PUCL-Phe2; the similar IR curves of the two fluorescent polymers demonstrate that these two samples may possess the same structure. The peaks that appeared at 1810 and 1345 cm−1 are ascribed to C(N) O and C–N, suggesting that the aldehyde-containing polymer and the amino-containing AIE dye were locked by the mercaptoacetic acid molecule to form the five-member heterocycle. Furthermore, the peaks from 640 to 980 cm−1 should be the fingerprint region of the aromatic rings, demonstrating the prosperous connection between the Phe-NH2 containing benzene rings and the aldehyde-containing polymer. | |
As is well known, fluorescent nanoparticles have promising application potential as bioprobes for bioimaging, targeted labeling and biosensing because of their easy endocytosis by cells. Therefore, the size and morphology of PPEGMA-co-PUCL-Phe2 was first evaluated by TEM. As shown in Fig. 3, the TEM image of PPEGMA-co-PUCL-Phe2 showed that the size of these particles ranges from 100–150 nm. The successful formation of nanoparticles implied that the obtained polymers can self-assemble during dispersion in aqueous solution. In other words, the self-assembly of these polymers evidenced the formation of amphiphilic PPEGMA-co-PUCL-Phe2 copolymers through the combination of the MALI reaction and RAFT polymerization. Among these nanostructures, the hydrophobic segments such as the AIE dye were aggregated in the inner and as the core, while the hydrophilic PEGMA will extend into water and act as the shell. Therefore, these luminescent particles are expected to possess high luminescence and good water dispersibility.
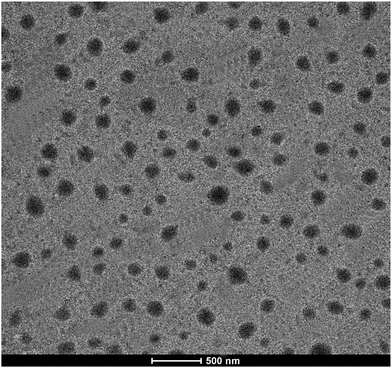 |
| Fig. 3 TEM image of PPEGMA-co-PUCL-Phe2 dispersed in aqueous solution. Scale bar = 500 nm. The quasi-spherical nanoparticles with sizes of 100–150 nm could be clearly observed. | |
These nanoparticles possess great water dispersibility, which could be verified from the inset of Fig. 4A. It can be seen that the word “AIE” was clearly observed in the background of the cuvette. The UV-Vis spectrum of the PPEGMA-co-PUCL-Phe2 water suspension exhibits two adsorption peaks at 307 nm and 428 nm (Fig. 4A). The adsorption peak at 307 nm could be attributed to the B adsorption band, suggesting that aromatic rings may exist in the fluorescent polymers. Furthermore, the adsorption peak at 428 nm should be ascribed to the n → π transition of the N and S atom direct linkage with the aromatic ring in Phe-NH2. This can serve as powerful evidence of successful conjugation of the AIE dye with the copolymer via combination of MCR and RAFT polymerization. On the other hand, because of Phe-NH2 possessing AIE features, which were reported from our previous work, the thus-obtained fluorescent copolymer also shows significant AIE characteristics.65 The fluorescence spectrum of PPEGMA-co-PUCL-Phe2 is shown in Fig. 4B; the maximum excitation and emission wavelengths are respectively located at 436 and 564 nm. The inset in Fig. 4B shows an optimal fluorescence image of PPEGMA-co-PUCL-Phe2; a strong orange fluorescence image could be observed under irradiating with a UV lamp at 365 nm. On the other hand, the fluorescence stability of LONs is an important property, which determines the performance of fluorescent probes. Therefore, the photostability of the synthetic fluorescent polymer was determined. As shown in Fig. S1 (ESI†), the position of the emission peak is not changed after irradiating under the UV lamp for 2 h, and the fluorescence intensity slightly reduces from 5860 to 5256 a.u., which is a reduction of about 604 a.u. within 2 h. Therefore, desirable photostability of PPEGMA-co-PUCL-Phe2 LONs makes them ideal candidates for biological imaging.
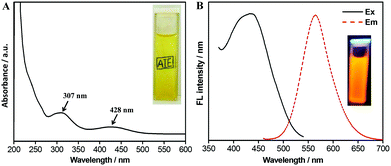 |
| Fig. 4 (A) A UV-Vis spectrum of PPEGMA-co-PUCL-Phe2 (5 mg/5 mL) dispersed in distilled water. In the inset, “AIE” was used as a background to demonstrate the great water dispersibility of the fluorescent polymer materials as shown by the clear observation of the “AIE” word. (B) The excitation and emission spectra of PPEGMA-co-PUCL & Phe-NH2-2 fluorescent materials (5 mg) dispersed in distilled water (10 mL); the inset shows strong orange light emission when irradiating under a UV lamp. | |
3.2 Biocompatibility of the PPEGMA-co-PUCL-Phe2 LONs
Great biocompatibility of fluorescent bioprobes is a basic and important condition for their promising biomedical application potential. To explore the potential biomedical application of PPEGMA-co-PUCL-Phe2 LONs, their cytocompatibility for HeLa cells was determined using the CCK-8 assay. As shown in Fig. 5, no obvious cell viability decrease could be observed after incubating with 10–120 μg mL−1PPEGMA-co-PUCL-Phe2 LONs for 12 and 24 h. The cell viability shows almost no change when incubated with 10–120 μg mL−1 fluorescent materials within 12 h. Furthermore, the cell viability even exceeds 95% when incubated with a concentration of fluorescent polymer of 120 μg mL−1 for 24 h. These results demonstrate that these AIE-active fluorescent polymers possess excellent biocompatibility for biomedical applications. Based on the unique features of PPEGMA-co-PUCL-Phe2 LONs such as AIE fluorescence, great water dispersibility, nanoscale size and desirable biocompatibility, they have promising biomedical application potential. Meanwhile, the method described in this work of combining MCR and RAFT polymerization is very simple and highly efficient due to the convenience of the one-pot reaction, and time-saving and facile conditions, leading to great potential for preparation of other functional polymer materials to extend the application breadth of conditional MCR and RAFT polymerization.
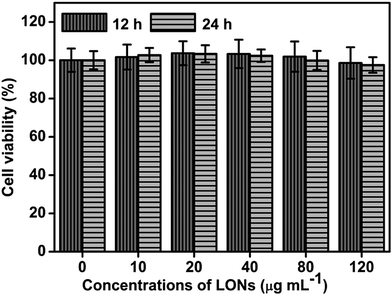 |
| Fig. 5 Cell viability evaluation of PPEGMA-co-PUCL-Phe2 LONs determined using the CCK-8 assay. HeLa cells were incubated with different concentrations of LONs for 12 and 24 h. No obvious decrease in cell viability could be observed according to the CCK-8 assay, demonstrating desirable biocompatibility of the prepared PPEGMA-co-PUCL-Phe2 LONs with living cells. | |
3.3 Biological imaging application of the PPEGMA-co-PUCL-Phe2 LONs
According to the foregoing analytical results, the prepared PPEGMA-co-PUCL-Phe2 LONs possess many outstanding features such as unique AIE fluorescence, great hydrophilicity, nanoscale size and desirable cytocompatibility. By taking advantage of these unique properties of AIE-active fluorescent micelles, their cell uptake behavior is evaluated by using Confocal Laser Scanning Microscopy (CLSM) observation. As shown in Fig. 6, after incubating with the PPEGMA-co-PUCL-Phe2 fluorescent polymer (20 μg mL−1), strong orange fluorescence could be observed in the edges of the cells, while a relatively weak fluorescence intensity is found in the core of the cells, which is ascribed to the possible position of the cell nucleus. This result demonstrates that there is a facile uptake of PPEGMA-co-PUCL-Phe2 LONs which mainly enter into the cytoplasm, and the uptake behavior of the LONs is caused by endocytosis of the cells. Furthermore, the cell imaging result suggests that synthetic PPEGMA-co-PUCL-Phe2 LONs have strong excellent stain performance for cells. Therefore, the thus-obtained PPEGMA-co-PUCL-Phe2 LONs are expected to serve as an ideal candidate for various biomedical applications such as cell imaging, drug delivery vehicles, targeting and tracing due to their excellent properties including high brightness, small sphere size, great photostability and biocompatibility. Moreover, due to the substitutability of the polymerization monomer, other functional components could be easily introduced into these fluorescent materials. Furthermore, this work provides a new fabrication method to prepare stable fluorescent nanoparticles for cell imaging.
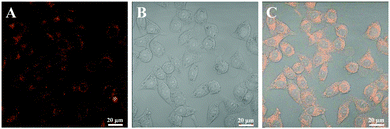 |
| Fig. 6 CLSM images of HeLa cells incubated with 20 μg mL−1PPEGMA-co-PUCL-Phe2 fluorescent polymers for 3 h at 37 °C. (A) Fluorescence imaging after excitation with a 458 nm laser, (B) bright field, and (C) merged image of A and B. Scale bar = 20 μm. | |
4. Conclusions
In summary, an AIE-active fluorescent polymer could be successfully prepared via the combination of MCRs and RAFT polymerization. The thus-obtained fluorescent amphiphilic polymers would self-assemble into nanoparticles in aqueous solution because the hydrophobic fluorogen curls up into the sphere core while the hydrophilic polymer is coated on the surface as the shell. From the characterization results, we can notice that synthetic fluorescent nanoparticles have many outstanding features such as strong fluorescence, small size, great hydrophilicity and desirable biocompatibility, which give them promising application potential in biomedical fields such as cell imaging, drug delivery vehicles, targeting markers and tracing. On the other hand, the method described in this work also has obvious advantages compared to the other strategies of construction of AIE-active polymer bioprobes. Firstly, all of the reactive components are put into one pot, which makes the operation process more convenient without undergoing complex purification procedures. Secondly, the integration of MCR and RAFT could develop a new direction for the fast preparation of more functional polymer materials, and this novel method combines the advantages of MCR (atom economy and facile procedure) and RAFT (well-organized polymer morphology and controlled molecular weight). Finally, due to the versatility of the MALI reaction for various amino and aldehyde-containing components, other functional components containing amino or aldehyde groups could be easily prepared for a versatile fluorescent nano-diagnosis system. Therefore, we expect these fluorescent nanoparticles could have wide biological application potential, and this method could provide inspiration for the preparation of other AIE-active functional materials.
Acknowledgements
This research was supported by the National Science Foundation of China (No. 51363016, 21474057, 21564006, 21561022), Natural Science Foundation of Jiangxi Province in China (No. 20161BAB203072, 20161BAB213066), the National 973 Project (No. 2011CB935700) and innovate Foundation of Nanchang University postgraduate (CX2015043).
Notes and references
- P. L. Golas and K. Matyjaszewski, Chem. Soc. Rev., 2010, 39, 1338–1354 RSC.
- A. Gole and C. J. Murphy, Langmuir, 2008, 24, 266–272 CrossRef CAS PubMed.
- R. K. Iha, K. L. Wooley, A. M. Nystrom, D. J. Burke, M. J. Kade and C. J. Hawker, Chem. Rev., 2009, 109, 5620–5686 CrossRef CAS PubMed.
- W. Xi, T. F. Scott, C. J. Kloxin and C. N. Bowman, Adv. Funct. Mater., 2014, 24, 2572–2590 CrossRef CAS.
- L. Banfi and R. Riva, Org. React., 2005, 65, 1–140 CrossRef CAS.
- J. Lu and Y. Bai, Synthesis, 2002, 0466–0470 CrossRef CAS.
- T. Nash, Biochem. J., 1953, 55, 416 CrossRef CAS PubMed.
- T. Lindhorst, H. Bock and I. Ugi, Tetrahedron, 1999, 55, 7411–7420 CrossRef CAS.
- H. Erlenmeyer and V. Oberlin, Helv. Chim. Acta, 1947, 30, 1329–1335 CrossRef CAS PubMed.
- O. Kreye, T. Tóth and M. A. Meier, J. Am. Chem. Soc., 2011, 133, 1790–1792 CrossRef CAS PubMed.
- O. Kreye, O. Türünç, A. Sehlinger, J. Rackwitz and M. A. Meier, Chem. – Eur. J., 2012, 18, 5767–5776 CrossRef CAS PubMed.
- A. Sehlinger, P. K. Dannecker, O. Kreye and M. A. Meier, Macromolecules, 2014, 47, 2774–2783 CrossRef CAS.
- X. Ren, B. Yang, Y. Zhao, X. Zhang, X. Wang, Y. Wei and L. Tao, Polymer, 2015, 64, 210–215 CrossRef CAS.
- R. Kakuchi, Angew. Chem., Int. Ed., 2014, 53, 46–48 CrossRef CAS PubMed.
- Y. Zhang, C. Fu, C. Zhu, S. Wang, L. Tao and Y. Wei, Polym. Chem., 2013, 4, 466–469 RSC.
- B. Yang, Y. Zhao, X. Ren, X. Zhang, C. Fu, Y. Zhang, Y. Wei and L. Tao, Polym. Chem., 2015, 6, 509–513 RSC.
- J. Geng, J. Lindqvist, G. Mantovani and D. M. Haddleton, Angew. Chem., Int. Ed., 2008, 47, 4180–4183 CrossRef CAS PubMed.
- Q. Zhang, Y. Zhang, Y. Zhao, B. Yang, C. Fu, Y. Wei and L. Tao, ACS Macro Lett., 2015, 4, 128–132 CrossRef CAS.
- J. Geng, K. Li, W. Qin, L. Ma, G. G. Gurzadyan, B. Z. Tang and B. Liu, Small, 2013, 9, 2012–2019 CrossRef CAS PubMed.
- X. He, J. Gao, S. S. Gambhir and Z. Cheng, Trends Mol. Med., 2010, 16, 574–583 CrossRef CAS PubMed.
- S. A. Hilderbrand and R. Weissleder, Curr. Opin. Chem. Biol., 2010, 14, 71–79 CrossRef CAS PubMed.
- F. Hu, Y. Huang, G. Zhang, R. Zhao, H. Yang and D. Zhang, Anal. Chem., 2014, 86, 7987–7995 CrossRef CAS PubMed.
- Q. Zheng, T. Lin, H. Wu, L. Guo, P. Ye, Y. Hao, Q. Guo, J. Jiang, F. Fu and G. Chen, Int. J. Pharm., 2014, 463, 22–26 CrossRef CAS PubMed.
- K. Mizusawa, Y. Takaoka and I. Hamachi, J. Am. Chem. Soc., 2012, 134, 13386–13395 CrossRef CAS PubMed.
- A. Shao, Y. Xie, S. Zhu, Z. Guo, S. Zhu, J. Guo, P. Shi, T. D. James, H. Tian and W. H. Zhu, Angew. Chem., Int. Ed., 2015, 54, 7275–7280 CrossRef CAS PubMed.
- X. Zhang, K. Wang, M. Liu, X. Zhang, L. Tao, Y. Chen and Y. Wei, Nanoscale, 2015, 7, 11486–11508 RSC.
- W. J. Jin, J. M. Costa Fernandez, R. Pereiro and A. Sanz Medel, Anal. Chim. Acta, 2004, 522, 1–8 CrossRef CAS.
- D. R. Larson, W. R. Zipfel, R. M. Williams, S. W. Clark, M. P. Bruchez, F. W. Wise and W. W. Webb, Science, 2003, 300, 1434–1436 CrossRef CAS PubMed.
- X. Michalet, F. Pinaud, L. Bentolila, J. Tsay, S. Doose, J. Li, G. Sundaresan, A. Wu, S. Gambhir and S. Weiss, Science, 2005, 307, 538–544 CrossRef CAS PubMed.
- B. Dubertret, P. Skourides, D. J. Norris, V. Noireaux, A. H. Brivanlou and A. Libchaber, Science, 2002, 298, 1759–1762 CrossRef CAS PubMed.
- W. Yin, L. Zhou, Z. Gu, G. Tian, S. Jin, L. Yan, X. Liu, G. Xing, W. Ren and F. Liu, J. Mater. Chem., 2012, 22, 6974–6981 RSC.
- X. Kang, C. Li, Z. Cheng, P. a. Ma, Z. Hou and J. Lin, Wiley Interdiscip. Rev.: Nanomed. Nanobiotechnol., 2014, 6, 80–101 CrossRef CAS PubMed.
- M. A. Habeeb Muhammed, P. K. Verma, S. K. Pal, A. Retnakumari, M. Koyakutty, S. Nair and T. Pradeep, Chem. – Eur. J., 2010, 16, 10103–10112 CrossRef CAS PubMed.
- L. Shang, S. Brandholt, F. Stockmar, V. Trouillet, M. Bruns and G. U. Nienhaus, Small, 2012, 8, 661–665 CrossRef CAS PubMed.
- N. Cao, Y. Zhao, B. Sang, Z. Wang, L. Cao, L. Sun and X. Zou, Mater. Sci. Eng., C, 2016, 69, 330–336 CrossRef CAS PubMed.
- M. E. Gallina, Y. Zhou, C. J. Johnson, D. Harris-Birtill, M. Singh, H. Zhao, D. Ma, T. Cass and D. S. Elson, Mater. Sci. Eng., C, 2016, 59, 324–332 CrossRef CAS PubMed.
- J. Liu, R. Hu, J. Liu, B. Zhang, Y. Wang, X. Liu, W.-C. Law, L. Liu, L. Ye and K.-T. Yong, Mater. Sci. Eng., C, 2015, 57, 222–231 CrossRef CAS PubMed.
- H. S. Mansur, A. A. Mansur, A. Soriano-Araújo, Z. I. Lobato, S. M. de Carvalho and M. de Fatima Leite, Mater. Sci. Eng., C, 2015, 52, 61–71 CrossRef CAS PubMed.
- A. Talib, S. Pandey, M. Thakur and H.-F. Wu, Mater. Sci. Eng., C, 2015, 48, 700–703 CrossRef CAS PubMed.
- C. Yi, L. Liu, C.-W. Li, J. Zhang and M. Yang, Mater. Sci. Eng., C, 2015, 46, 32–40 CrossRef CAS PubMed.
- S. W. Thomas, G. D. Joly and T. M. Swager, Chem. Rev., 2007, 107, 1339–1386 CrossRef CAS PubMed.
- M. Kumar and S. J. George, Nanoscale, 2011, 3, 2130–2133 RSC.
- J. Luo, Z. Xie, J. W. Lam, L. Cheng, H. Chen, C. Qiu, H. S. Kwok, X. Zhan, Y. Liu, D. Zhu and B. Z. Tang, Chem. Commun., 2001, 1740–1741 RSC.
- W. Qin, D. Ding, J. Liu, W. Z. Yuan, Y. Hu, B. Liu and B. Z. Tang, Adv. Funct. Mater., 2012, 22, 771–779 CrossRef CAS.
- D. Ding, C. C. Goh, G. Feng, Z. Zhao, J. Liu, R. Liu, N. Tomczak, J. Geng, B. Z. Tang and L. G. Ng, Adv. Mater., 2013, 25, 6083–6088 CrossRef CAS PubMed.
- K. Li, W. Qin, D. Ding, N. Tomczak, J. Geng, R. Liu, J. Liu, X. Zhang, H. Liu and B. Liu, Sci. Rep., 2013, 3, 1150 Search PubMed.
- J. Geng, K. Li, D. Ding, X. Zhang, W. Qin, J. Liu, B. Z. Tang and B. Liu, Small, 2012, 8, 3655–3663 CrossRef CAS PubMed.
- Z. Long, M. Liu, K. Wang, F. Deng, D. Xu, L. Liu, Y. Wan, X. Zhang and Y. Wei, Mater. Sci. Eng., C, 2016, 66, 215–220 CrossRef CAS PubMed.
- Z. Long, M. Liu, Q. Wan, L. Mao, H. Huang, G. Zeng, Y. Wan, F. Deng, X. Zhang and Y. Wei, Macromol. Rapid Commun., 2016, 37, 1754–1759 CrossRef CAS PubMed.
- Z. Long, M. Liu, Q. Wan, L. Mao, H. Huang, G. Zeng, Y. Wan, F. Deng, X. Zhang and Y. Wei, Macromol. Rapid Commun., 2016, 37, 1657–1661 CrossRef CAS PubMed.
- X. Zhang, M. Liu, B. Yang, X. Zhang and Y. Wei, Colloids Surf., B, 2013, 112, 81–86 CrossRef CAS PubMed.
- X. Zhang, X. Zhang, B. Yang, M. Liu, W. Liu, Y. Chen and Y. Wei, Polym. Chem., 2013, 4, 4317–4321 RSC.
- H. Li, X. Zhang, X. Zhang, B. Yang and Y. Wei, Colloids Surf., B, 2014, 121, 347–353 CrossRef CAS PubMed.
- X. Zhang, X. Zhang, B. Yang, J. Hui, M. Liu, Z. Chi, S. Liu, J. Xu and Y. Wei, Polym. Chem., 2014, 5, 683–688 RSC.
- X. Zhang, X. Zhang, B. Yang, L. Liu, F. Deng, J. Hui, M. Liu, Y. Chen and Y. Wei, RSC Adv., 2014, 4, 24189–24193 RSC.
- Z. Huang, X. Zhang, X. Zhang, S. Wang, B. Yang, K. Wang, J. Yuan, L. Tao and Y. Wei, RSC Adv., 2015, 5, 89472–89477 RSC.
- Q. Wan, C. He, K. Wang, M. Liu, H. Huang, Q. Huang, F. Deng, X. Zhang and Y. Wei, Tetrahedron, 2015, 71, 8791–8797 CrossRef CAS.
- Q. Wan, K. Wang, C. He, M. Liu, G. Zeng, H. Huang, F. Deng, X. Zhang and Y. Wei, Polym. Chem., 2015, 6, 8214–8221 RSC.
- Q. Wan, M. Liu, D. Xu, H. Huang, L. Mao, G. Zeng, F. Deng, X. Zhang and Y. Wei, J. Mater. Chem. B, 2016, 4, 4033–4039 RSC.
- Q. Wan, K. Wang, H. Du, H. Huang, M. Liu, F. Deng, Y. Dai, X. Zhang and Y. Wei, Polym. Chem., 2015, 6, 5288–5294 RSC.
- I. R. Schmolka and P. E. Spoerri, J. Am. Chem. Soc., 1957, 79, 4716–4720 CrossRef CAS.
- Y. Zhao, B. Yang, C. Zhu, Y. Zhang, S. Wang, C. Fu, Y. Wei and L. Tao, Polym. Chem., 2014, 5, 2695–2699 RSC.
- X. Zhang, J. Ji, X. Zhang, B. Yang, M. Liu, W. Liu, L. Tao, Y. Chen and Y. Wei, RSC Adv., 2013, 3, 21817–21823 RSC.
- Z. Long, M. Liu, K. Wang, F. Deng, D. Xu, L. Liu, Y. Wan, X. Zhang and Y. Wei, Mater. Sci. Eng., C, 2016, 66, 215–220 CrossRef CAS PubMed.
- J. Chen, S. Luo, D. Xu, Y. Xue, H. Huang, Q. Wan, M. Liu, X. Zhang and Y. Wei, RSC Adv., 2016, 6, 54812–54819 RSC.
Footnotes |
† Electronic supplementary information (ESI) available: The photostability of PPEGMA-co-PUCL-Phe2 was provided. See DOI: 10.1039/c6qm00307a |
‡ These authors contributed equally to this work. |
|
This journal is © the Partner Organisations 2017 |