DOI:
10.1039/C6MB00654J
(Review Article)
Mol. BioSyst., 2017,
13, 32-41
Model studies of the effects of intracellular crowding on nucleic acid interactions
Received
14th September 2016
, Accepted 26th October 2016
First published on 7th November 2016
Abstract
Molecular interactions and reactions in living cells occur with high concentrations of background molecules and ions. Many research studies have shown that intracellular molecules have characteristics different from those obtained using simple aqueous solutions. To better understand the behavior of biomolecules in intracellular environments, biophysical experiments were conducted under cell-mimicking conditions in a test tube. It has been shown that the molecular environments at the physiological level of macromolecular crowding, spatial confinement, water activity and dielectric constant, have significant effects on the interactions of DNA and RNA for hybridization, higher-order folding, and catalytic activity. The experimental approaches using in vitro model systems are useful to reveal the origin of the environmental effects and to bridge the gap between the behaviors of nucleic acids in vitro and in vivo. This paper highlights the model experiments used to evaluate the influences of intracellular environment on nucleic acid interactions.
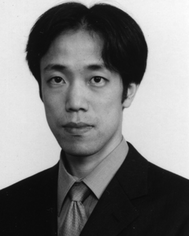
Shu-ichi Nakano
| Shu-ichi Nakano is a professor at the Department of Nanobiochemistry, Faculty of Frontiers of Innovative Research in Science and Technology (FIRST) at Konan University in Japan. He received his PhD in 1999 from Konan University, under the direction of Prof. Naoki Sugimoto. After postdoctoral work at the Pennsylvania State University in the USA, he joined Konan University in 2001. He became a professor in 2013. His research interests lie in the specificity and redundancy in the functions of nucleic acids and proteins. He has published about 100 scientific papers, reviews, and books. |
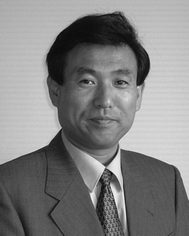
Naoki Sugimoto
| Naoki Sugimoto received his PhD in 1985 from Kyoto University, Japan. After postdoctoral work at the University of Rochester, NY in the USA, he joined Konan University, Japan in 1988 and has been a full Professor since 1994. From 2003, he has been a director at the Frontier Institute for Biomolecular Engineering Research (FIBER) at Konan University. He is a member of the Editorial Board of Nucleic Acids Research and Scientific Reports. He received the Chemical Society of Japan Award for Creative Work in 2007. His research interests focus on biofunctional chemistry, especially nucleic acid chemistry. He has published about 500 scientific papers, reviews, and books. |
1. Introduction
Biomolecules possess unique physical and chemical properties critical to cellular processes, such as spontaneous folding, self-assembly, high target specificity and catalytic activities. Most biomolecular materials including proteins and nucleic acids can now be obtained through methods of molecular biology and chemical synthesis. The artificially-prepared molecules are widely utilized to investigate biomolecular interactions and their functions in living cells. Most of the studies use simple aqueous solutions with an ionic strength and pH similar to those inside cells. In the in vitro experiments, one can apply sophisticated techniques and technologies, such as biochemical studies and spectroscopic methods, for the quantitative analysis of the structure, dynamics and thermodynamics of the biomolecules of interest. Results from these studies are useful to speculate on the interactions and functions in cells. However, the approach applies the knowledge from in vitro studies to cellular phenomena. The molecular environments are considerably different among the cytoplasm, nucleus and cell organelles,1 and these intracellular environments are quite distinct from a diluted aqueous solution.2 Recent studies have revealed the significance of the molecular environment on the efficiency of nucleic acid interactions in cells. It is remarkable that DNA hybridization kinetics, gene expression, RNA folding and the catalytic activity of ribozymes monitored in vivo are often more effective than those expected from in vitro studies.3–7 It is also an important finding that the thermal stability of an RNA structure is variable within the subcellular regions of the cytoplasm and nucleus.8 These observations indicate that the intracellular environment affects the properties of the nucleic acids differently from those studied using simple aqueous solutions. To elucidate their “real” behaviors in biosystems, it is important to elucidate the thermodynamic properties of the nucleic acid interactions in the intracellular environments.
In cells, molecular interactions and reactions occur with high amounts of background molecules and ions (Fig. 1A). The coexisting components may cause specific interactions with biomolecules and also indirectly influence the biomolecular reactions through steric hindrance. Moreover, the physical properties of intracellular water differ from a diluted aqueous solution. It is important to consider the effects of the intracellular environment for better understanding of the behavior of biomolecules in cells. However, the effects of the background cellular components are complicated, and systematic and quantitative experiments using living cells are not easy. There are computer simulation studies of cellular components within the cells. These simulations have shown alterations in the protein dynamics and structures under macromolecular crowding conditions and revealed significant contributions of the steric effect and the physical properties of the medium to the protein reactions.9–13
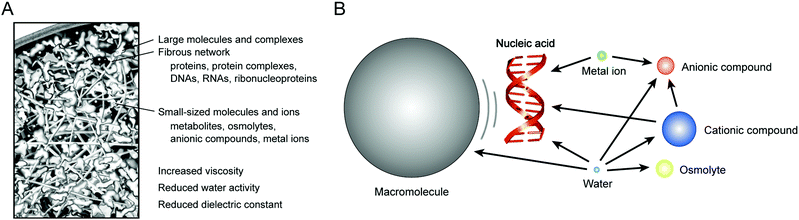 |
| Fig. 1 Schematic illustrations of the interior of a living cell and characteristic features of the intracellular environment (A) and possible interactions of a nucleic acid with various types of cellular components (B). | |
In vitro studies using the buffered solutions and nanomaterials creating cell-mimicking conditions are increasingly important as an experimental approach. It is possible to control the molecular environment comparable to the physiological level of the macromolecular crowding and solvent properties. The experiments enable reproducible analysis and assessment of the effects of a change in the molecular environment, providing the results complementary to the findings from the computer simulation studies. Research on nucleic acids is especially intriguing because the polar and charged polymer chain (anionic polyelectrolyte composed of nucleotides) interacts with many cellular components (Fig. 1B). Recent studies using various experimental model systems have provided data showing the contributions of individual environmental factors to the hybridization, conformation and enzymatic activities of nucleic acids. The phenomena are, in many cases, explained by the effects of the excluded volume, chemical interactions and solvent properties. In this review, we would like to highlight the model experiments used to evaluate the influences of the intracellular environment on the nucleic acid interactions. The characteristics of the intracellular environment and experimental methodologies are introduced in the following sections: Section 2 describes how the intracellular environment affects the nucleic acid interactions in cells. Section 3 describes the studies using water-soluble polymer cosolutes, proteins and nanoparticles for evaluating the effects of macromolecular crowding. Section 4 describes the studies using nanomaterials for evaluating the effects of spatial confinement in a small space. Section 5 describes the studies using aqueous mixtures with organic solvents for evaluating the effects of the unique solvent properties of the intracellular milieu. The model experimental systems are obviously oversimplified, however, the approaches are effective for speculating about the structures and functions of the intracellular nucleic acids in order to understand the biophysical system in the environment of the cells.
2. Effects of intracellular environments on nucleic acids in cells
Living cells contain large amounts of molecules and ions, and the cellular components directly and indirectly influence the biomolecular processes. One of the most distinguishing features of intracellular environments is the condition of macromolecular crowding.14,15Escherichia coli contains 300–400 mg of proteins and nucleic acids per mL, and eukaryotic cells contain 50–400 mg and 100–400 mg per mL of cytoplasm and nucleus, respectively.16 These amounts are much higher than those used for typical biophysical experiments using a simple aqueous solution. The crowded environment is related to the regulation of gene transcription and translation.17–19 The changes in the degree of molecular crowding accompanied by a change in the cell volume affects cellular functions, such as cell death, metabolism and the pathophysiology of several diseases.20 From the standpoint of physical chemistry, the presence of large-sized cellular components changes the equilibrium constant of molecular interactions21 and slows the diffusion and recognition of proteins and nucleic acids.22–24 It is proposed that the effects of molecular crowding on biochemical networks are mainly caused by equilibrium shifts rather than reduced diffusion rates.25 The density of proteins in the cellular membrane is also high, such as 25
000 proteins per μm2 occupying more than 20% of the area of the plasma membrane of a human red blood cell.26 The crowded environment affects the diffusion rate, interaction and conformation of membrane proteins.27 Furthermore, the actin cytoskeleton in cells and inside cell organelles create a confined space where proteins and nucleic acids are constrained in their ability to move and collide with other molecules. This confinement restricts the conformational space of polymeric molecules and increases the extent of molecular crowding. Controlled confinement of DNA in the cell nucleus is supposed to be related to genome organization and chromosome localization.28,29
There are a large number of small-sized molecules, such as metabolites and osmolytes at submolar to molar concentrations.2,30 The major intracellular osmolytes have no net charge at physiological pH. However, they may be preferentially bound to or excluded from the surface of biomolecules, causing the stabilization or destabilization of the folded structures. It is a plausible hypothesis that some natural osmolytes act as chemical chaperones to buffer the mutational variants of proteins by modulating the folding stability.31 Likewise, the stability of nucleic acid structures changes in the presence of typical osmolytes, such as trimethylamine N-oxide, glycine betain and urea at concentrations comparable to intracellular concentrations.32–34 Many types of negatively- or positively-charged molecules and ions are also present in cells. Anionic compounds that have important biological functions are abundant, such as adenosine triphosphate, at the concentration of several millimolars. It is notable that the amount of the negatively-charged phosphate group of DNA and RNA is high, on the order of a few to a hundred millimolar. In addition, fatty acids are the components of cellular and organelle membranes and create a highly negatively-charged surface. On the other hand, positively-charged polyamines, including putrescine, spermidine and spermine, are present at a few to several tens of millimolar. The concentration of monovalent metal ions reaches a few hundred millimolar and that of divalent metal ions is several to tens of millimolar; e.g., the concentration of K+ is 100–250 mM and that of Mg2+ is 20–100 mM. Nevertheless, biological cells do not contain enough diffusible metal ions because most of them are associated with anionic components.35 For example, the free concentration of Mg2+ in the cytoplasm of mammalian cells is 1 mM or less and that of Ca2+ is less than 1 μM; these amounts in an unbound state are linked to the regulation of enzymatic activities and cellular functions. Importantly, the negative charge of nucleic acids is screened by cations bound to specific sites and those bound nonspecifically through electrostatic interactions,36 which is essential for the formation of electrostatically condensed structures, including Watson–Crick base pairing and higher-order folding.37
The physical properties of intracellular water are altered as the result of association with the cellular components. The hydrating water molecules and more long-range ones are less mobile, resulting in an increase in the osmotic pressure and a reduction in the water activity compared to pure water.35,38 Because nucleic acids are highly hydrated in aqueous media, the intracellular environment with low water activity affects the formation of nucleic acid structures. Moreover, the dielectric constant of the intracellular milieu is estimated to be around 60 or less, varied between subcellular regions,1,9,39 and the values are substantially lower than the value of pure water at about 80. Because the dielectric constant determines the strength of electrostatic interactions, media with a low dielectric constant in cells and cell organelles has a significant impact on the electrostatic interactions of nucleic acids.
As previously explained, the molecular environment in cells affects the energy levels of nucleic acids and the thermodynamic properties of the structural transitions for hybridization and folding by a combination of mechanisms (Fig. 2). Recent model experiments using media mimicking intracellular conditions have demonstrated how environmental factors influence the structures and functions of DNA and RNA. These experiments are described in the following sections.
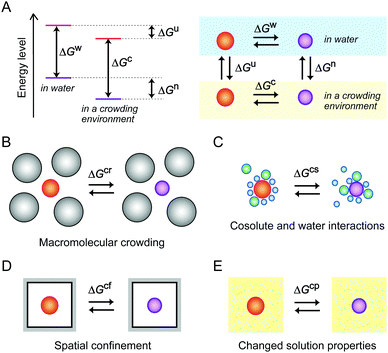 |
| Fig. 2 Gibbs free energy changes for the structural transition of nucleic acids from a less-folded state (red) to a folded state (purple) in different environments. Free energy levels and their relationships in water and in a crowded environment (A), and the free energy changes under macromolecular crowding (B), a condition of cosolute and water interactions (C), spatial confinement (D) and the condition of changed solution properties (E). | |
3. Effects of macromolecular crowding: studies using large-sized additives
3.1. Studies using water-soluble polymer cosolutes
A large proportion of the cell volume is occupied by proteins, nucleic acids and cell organelles. The occupied space is inaccessible to other molecules, resulting in the restriction of the dynamics of cellular molecules and an increase in their effective concentrations. A simple calculation assuming the excluded volume effect between hard particles predicts that the activity coefficient of biomolecules increases in a highly nonlinear fashion as the fractional volume occupancy increases.21 It is predicted that the excluded volume effect of large-sized cellular components shifts the equilibrium to facilitate the structural transitions that reduce the net volume, such as the formation of compact and ordered structures. In such cases, the value of the free energy change for the transition is lower than that in a diluted aqueous solution (ΔGcr < ΔGw, in Fig. 2B).
Aqueous solutions with large-sized additives are used as a simplified experimental system for macromolecular crowding (Fig. 3). In order to prepare a highly crowded solution comparable to the physiological level of macromolecular crowding, water-soluble polymers are convenient. The polymer concentration used in the studies is, for example, on the order of submillimolar when the molecular weight is 1 × 105. Because the polymers need to be highly soluble in water and act as cosolutes, there are limited types of polymer materials applicable for the preparation of crowded solutions. Poly(ethylene glycol) (PEG) and polysaccharides are widely used as artificial crowding agents to evaluate the effects of macromolecular crowding on proteins and nucleic acids. These polymers are water soluble and have no charged groups. Moreover, polymers with different molecular weights are commercially available. The preparation and handling of the crowded solutions are simple; a polymer material is dissolved in water containing buffering agents and salts (when necessary) to be at a few to several tens of weight percent by weight or volume, and the degree of macromolecular crowding is controlled by applying different polymer concentrations.40 For the evaluation of the excluded volume effect, polymers of different sizes are used to verify the dependence of the size of the crowding agents predicted by the steric exclusion model.21
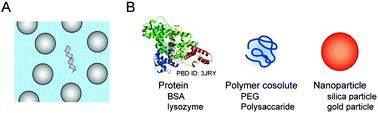 |
| Fig. 3 Model experimental systems for the study of the excluded volume effect by large cellular components (A) and the different types of additives used for the study (B). | |
There are many reports of proteins showing an increased folding stability and a higher enzymatic activity in polymer-containing solutions.41,42 One example is the compaction of intrinsically disordered proteins induced by the addition of crowding agents such as PEG, dextran, polyvinyl alcohol or polyvinylpyrrolidone, the efficiency of which depends on the size of the polymers.43 It is also remarkable that a study using mixed molecular crowding agents, dextran and Ficoll, shows a synergetic effect on the protein stability greater than the sum of the individual crowding agents, attributed to the greater excluded volume effect of the different-sized cosolutes.44 In the experiments using polymer cosolutes, the environmental factors besides the steric effect also need to be considered. There is the possibility of chemical interactions with the used polymers. In addition, dissolving a polymer in water increases the viscosity and alters the physical properties of the solution. The effects of these factors are not mutually exclusive, which is problematic in interpreting experimental data in terms of the excluded volume effect. As for nucleic acids, it is more difficult to evaluate the effects of individual environmental factors and to identify the origin of the polymer effects. This is because nucleotides have many hydrogen bonding groups, hydrophobic base moieties and phosphate groups that may preferentially interact with crowding agents and with water and ions subject to the physical properties of a solution. With the study using polymer cosolutes, it is difficult to separate the steric effect from the effects of direct interactions. It is argued that some polymers, such as PEG, interact with nucleotide bases due to their hydrophobic nature.8,45 One of the methods used to estimate specific interactions with particular polymer cosolutes is to examine chemically-different polymers and compare their effects. Therefore, special attention is necessary for choosing the crowding agents and explaining the experimental data.
DNA polynucleotides, such as genome DNA and plasmid DNA, are compacted and form aggregates and precipitates in PEG-containing solutions;46,47 this phenomenon appears to be analogous to DNA packing in the cell nucleus. This compaction is explained by the promotion of volume-reducing reactions under the condition of macromolecular crowding. Stabilization of a folded conformation and promotion of the folding cooperativity of RNA under macromolecular crowding by PEG and polysaccharides are also reported.48,49 Intriguingly, the crowded environment broadens the fitness landscape of a mutant of the Azoarcus group I ribozyme by stabilizing the compact tertiary structures. In addition, the concentration of RNA is enriched in the dextran-rich phase of the aqueous two-phase system formed by mixing PEG and dextran, and this leads to an increase in the rate of substrate cleavage by the hammerhead ribozyme.50 These observations imply the role of the environment of macromolecular crowding in the evolution of catalytic RNAs.51
Examinations with synthetic short oligonucleotides are effective for the evaluation besides the steric effect. Oligonucleotides are less likely to precipitate with polymer cosolutes even at relatively high concentrations. Moreover, sequences can be designed to adopt distinctive secondary and tertiary structures and may incorporate probe molecules and chemical modifications applicable for various experimental analyses. It was found that the condition of macromolecular crowding by PEG at a few tens of percent increases the base-pair stability of short oligonucleotides under a low, but not high, concentration of salts without disrupting the double-helical conformation.52,53 The crowded solution of a few tens of percent PEG also promotes the formation of short DNA hairpins and the hybridization of two hairpins with low salt concentrations.54,55 Furthermore, the crowded conditions due to PEG and polysaccharides accelerate the catalytic activity of several types of ribozymes (hammerhead ribozyme, human hepatitis delta virus (HDV)-like ribozyme, hairpin ribozyme and Azoarcus group I ribozyme) particularly at relatively low salt concentrations.56–61 It appears that the molecularly crowded environment at physiological salt concentrations is favorable for nucleic acid interactions for the hybridization and folding while diffusion-limited processes are unfavorable under high viscosity conditions.
3.2. Studies using model proteins and nanoparticles
Many cellular components possess charged and hydrophobic surfaces. Chemical interactions with these components change the energy levels of the nucleic acid structures, resulting in stabilization or destabilzation depending on the number and strength of the interactions (ΔGcs < ΔGw or ΔGcs > ΔGw, in Fig. 2C). A computer simulation study showed that base-pair hydrogen bonds and base stacking of a DNA duplex are strengthened or weakened at the interface of hydrophilic and hydrophobic interfaces.62 To advance the understanding of the effects of intracellular crowding, investigations with the use of protein crowders are important because proteins are composed of charged and hydrophobic amino acids. However, there are certain limitations in the experiments; the study requires concentrated protein solutions, but many proteins do not have a sufficiently high solubility in water and the preparation requires a high cost. In addition, the use of cell lysates is problematic because of easy degradation of nucleic acid strands and difficulty in controlling the experimental conditions such as protein and ion concentrations. Several studies have used commercially-available proteins that are abundant in blood plasma or chicken egg although they are not of intracellular origin. A basic protein, lysozyme, was shown to bind to and induce a change in the plasmid DNA conformation with a relatively low amount.63 The compaction of DNA polynucleotides also occurred when an acidic protein, bovine serum albumin (BSA), was used; this phenomenon was explained by the steric and electrostatic repulsion between the BSA molecules.64 These studies indicate that the electrostatic interactions significantly contribute to the effects of protein crowding on the helical geometry of polynucleotides. Further investigations regarding the effects of charged surfaces will be necessary to clarify the effects of protein crowding in cells.
The significance of surface charge has also been demonstrated through experiments using inorganic spherical particles. Nanoparticles mimic globular proteins in their spherical shape, and the boundary of the particles is impenetrable, while the boundary of polymer cosolutes is flexible and penetrable.65 Nanoparticles are prepared via a sol–gel process (e.g., for silica particles) or via chemical reduction of metal ions using a reducing agent (e.g., for gold nanoparticles). The surface of the nanoparticles has a high density of positive or negative charges, and the particle size can be controlled by varying the solution composition or by the concentration, pH and temperature during the preparation. The presence of negatively-charged silica particles of 20–130 nm at concentrations of several percent is reported to induce the compaction of DNA polynucleotides and decrease the base-pair stability.66 In contrast, another study using a lower amount of silica particles at 0.03 wt% showed that the particles of 15 nm do not change the stability of a DNA duplex but those of 100 nm slightly increase the stability, while poly-L-lysine deposited cationic silica nanoparticles at the same amount tightly bind to the DNA and inhibit its thermal denaturation.67 These observations indicate that the conformation and stability of nucleic acids are affected by negatively-charged cellular components, as well as positively-charged ones, even at a relatively low amount that provides a less excluded volume. Furthermore, anionic components not only cause repulsive interactions with nucleic acids and between each other, but also decrease the amount of cations in the media available to nucleic acids. In particular, the conditions in the presence of fatty acid vesicles, that are a model of cell membranes, decrease the catalytic activities of RNA-cleaving and RNA-ligating ribozymes, attributed to the reduction in the amount of free Mg2+ in the solutions.61,68 It can be regarded that electrostatic attractive and repulsive interactions, in addition to the steric effect, are significant for nucleic acids in the crowded environment of cells.
4. Effects of spatial confinement: studies using nanomaterials
4.1. Studies using porous and compartment materials
The cytoskeleton forms a complex network of protein fibers, and the extracellular matrix forms a fibrous network of polysaccharides and proteoglycans. These interconnected porous structures create a confined condition. The environments in the pores reduce the conformational space of polymeric molecules. It is predicted that expanded unfolded conformations are restricted in a confined space, resulting in the stabilization of the compact folded conformations (ΔGcf < ΔGw, in Fig. 2D).69,70
Hydrogels create a spatially-confined environment with a high water content (Fig. 4A). Agarose and polyacrylamide gels are often used, and their pore size can be controlled by varying the gel concentration. Agarose gel is prepared by dissolving agarose powder in water by heating, and a porous matrix with a pore size ranging from a few tens to a few hundreds of nanometers is formed due to hydrogen bonding between the polysaccharide chains. On the other hand, polyacrylamide gel is a chemical gel with pore sizes in the nanometer range formed due to covalent cross linking between the polymerized acrylamide chains. Because the polyacrylamide gel is generated by radical polymerization, the embedding of biomolecules in the gel is not straightforward.71 There are reports that several proteins have an increased resistance to the thermal fluctuation and decreased conformational dynamics when embedded in a 1% agarose gel or a 8% polyacrylamide gel.72–74 In contrast, there are few studies of nucleic acids embedded in the gels,71,75 whereas gel electrophoresis is popular for the analysis of nucleic acids.
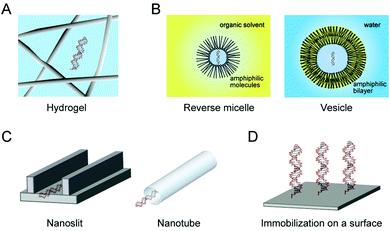 |
| Fig. 4 Model experimental systems for the study of the effects of spatial confinement in the pores of hydrogels (A), in micelles and vesicles of amphiphilic molecules (B), on the surface or inner space of nanomaterials (C) and through immobilization on a material surface (D). | |
The other method to create confined environments is the use of self-assembled molecules forming small impenetrable compartments of micelles or vesicles as a model of cellular compartmentalization (Fig. 4B). The materials are prepared via phase separation of amphiphilic molecules, and the size of the compartments is controlled by the preparation methods and the type and amount of amphiphilic molecules. It is remarkable that RNA oligonucleotides and Mg2+ in an aqueous solution are highly concentrated within the coacervates formed upon mixing nucleotides with poly(allylamine).76 There are also reports on the stability and assembly of nucleic acid structures embedded in reverse micelles with a few nanometers in diameter formed by cetyltrimethylammonium or bis(2-ethylhexyl)sulfosuccinate,77,78 and on the rate of RNA cleavage by a hammerhead ribozyme in vesicles formed by a mixture of myristoleic acid and its glycerol monoester with a 100 nm-diameter.79 These studies show the significant influences of compartmentalization on the structural stability and catalytic activity of nucleic acids. Further research is required to determine the factors causing these effects, although there are some difficulties in the preparation and investigation of the nucleic acids embedded in the compartment materials.
4.2. Studies using nanomaterials
Geometric constraints are imposed on nucleic acids in the confined spaces of micro- and nano-devices developed for nucleic acid analysis with a small sample volume and high sensitivity (Fig. 4C).80,81 The nanomaterials are prepared using nanotechnology, such as the advanced lithography technique and the controlled assembly of precursor substances. The dynamics and conformation of DNA polynucleotides in the confined space of a glass nanoslit channel with a submicrometer height and a few hundred micrometers wide are reported.82 The study showed the enrichment of counterions at the negatively-charged surface of the channel and the alteration in the efficiency of ion interactions with DNA. Intriguingly, the compaction of DNA polynucleotides induced by dextran is facilitated when confined in the flow path of a nanochannel of a few hundred nanometers,83 and the response of DNA to dextran is different among the studies using a solution, nanoslit and nanotube.84 These observations indicate that the confined environments enhance the effect of macromolecular crowding depending on the morphology of the confinement.
The condition that forces DNA to be less flexible and sterically crowded as seen in cells is created when DNA is immobilized on a solid material via covalent bonding (Fig. 4D). The conformational constraints of DNA and the steric hindrance on the surface of flat plates (e.g., DNA microarray) or nanoparticles (e.g., gold nanoparticle coated with DNA monolayers) affects the hybridization, conformation and structural stability of nucleic acids.85 Again, it was found that DNA molecules immobilized on gold nanoparticles are sensitive to the presence of PEG,86 supporting the fact that the sterically-confined conditions amplify the effect of macromolecular crowding.
Under the conditions of macromolecular crowding and spatial confinement, water interactions are perturbed and the mobility, density and hydrogen-bonded network are different from bulk water.35,87 It is important to consider the physical properties of solvent water under the intracellular environments. The next section introduces studies from the standpoint of solvent properties.
5. Effects of solvent properties: studies using organic solvents
5.1. Studies using organic solvent compounds
The energy levels of nucleic acid states and, thereby, the energy differences for hybridization and folding are changed depending on the physical properties of the medium (ΔGcp < ΔGw or ΔGcp > ΔGw, in Fig. 2E). Water-soluble organic solvents acting as cosolvents, such as small alcohols and low-molecular-weight PEG, can be used to change the physical properties of an aqueous solution. These compounds do not produce significant excluded volume effects but have the distinct nature of hydrogen bonding, polarity and hydrophobicity. The addition of organic solvents to water creates the cosolvent–cosolvent and water–cosolvent interactions and disrupts the short-range and long-range water–water interactions. Aqueous solutions mixed with a relatively large amount of organic solvents (e.g., 20%) have a low water content and a low dielectric constant (Fig. 5), which are characteristic of the intracellular milieu. Because mixed solutions at very high concentrations decrease the solubility of the nucleic acids and denature their structures, aqueous mixtures up to several tens of percent, corresponding to several molar concentrations, are used in such studies.88 By assuming that the solution properties are uniform throughout the solution, it is possible to investigate the origin of the solvent effects by varying the proportion of the organic solvents; a correlation analysis between an interaction parameter of nucleic acids (e.g., thermodynamic stability) and the parameter of a certain solvent property (e.g., water activity) can identify major environmental factors. However, a change in the proportion of the organic solvents simultaneously alters multiple solvent properties, causing difficulties in distinguishing the contribution of each property. It is alternatively possible to systematically change a certain solvent property through the use of several types of organic solvents of different natures. This approach further enables us to evaluate the possible chemical interactions with particular solvents.89,90 Such studies have highlighted the significance of the conditions of water activity and dielectric constant on the strength of nucleic acid interactions, although these environmental factors appear to act differently according to the folded conformation, the contributions of metal ions and water and the salt concentration in the medium. The following sections describe the studies on the stability of nucleic acid structures and the catalytic activities of ribozymes in terms of the water activity and dielectric constant effects.
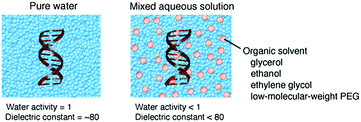 |
| Fig. 5 Schematic illustrations of the molecular environments and the solvent properties of pure water and a mixed aqueous solution. | |
5.2. Studies in terms of water activity
There are a limited number of free water molecules in cells. This condition can be created using an aqueous mixture with organic solvents that act as osmolytes reducing the activity of water. Many water molecules solvate nucleotide bases and sugar-phosphate backbones and form a hydrogen-bonding network in the double-helical groove.91 Therefore, the energy levels of the nucleic acid structures are predicted to be changed in the low water activity media. Small-sized hydrophilic organic solvents, such as glycerol, ethanol, ethylene glycol and low-molecular-weight PEG, effectively reduce the water activity of an aqueous solution. It is reported that, as the proportion of the organic solvents increases, the stability of the duplexes of DNA and RNA is decreased.92,93 On the contrary, the stabilities of the left-handed double helix, triplex, G-quadruplex and tertiary structures, in many cases, are increased, explained by the different contributions of water to the non-canonical structures compared to the duplex.16 Furthermore, the use of mixed solutions with low-molecular-weight PEG increases the stability of the catalytically-active structures of the hairpin and lead-dependent ribozymes and enhances their catalytic activities for the target RNA cleavage.94,95 The same explanation in terms of the water activity effects is applied to RNA tertiary folding and the formation of DNA–protein and DNA–drug complexes.16 These observations suggest a role for an intracellular environment with a low water activity on the structural polymorphism of nucleic acids.
5.3. Studies in terms of the dielectric constant
Aqueous mixtures with organic solvents also create an environment with a low dielectric constant. Many organic solvents have a lower dielectric constant value than water, and increasing the proportion of these organic solvents results in a decrease in this value. In principle, electrostatic attraction and repulsion become greater as the magnitude of the dielectric constant decreases. The helical conformation of DNA polynucleotides is compacted in the mixed solutions with glycerol, ethylene glycol or 1,2-dimethoxyethane due to the enhancement of DNA condensation by cations under low dielectric constant conditions.96 A study using RNA oligonucleotides further showed that the helical conformation of single strands, but not short duplexes, is changed in various types of mixed solutions, attributed to enhanced cation binding to the strands with a flexible nature.97 Solution media with a low dielectric constant are also reported to facilitate Na+ binding during duplex formation,59 stabilization of a DNA G-quadruplex formed by K+ binding,98 dimerization of two hairpins induced by cation binding,54 and changes in RNA tertiary interactions accompanied by Mg2+ binding.99 The facilitation of metal ion binding is caused by an increased electrostatic binding affinity, which makes the nucleic acid hybridization and folding more effective at relatively low salt concentrations. Metal ion binding is crucial for RNA-cleaving ribozymes during the transesterification of the RNA substrate as well as the formation of catalytically-active structures. Evaluations of relatively low salt concentrations show that the catalytic activity of ribozymes is enhanced in various solutions containing organic solvents.56–59,94,100 It is remarkable that these studies exhibit relatively small effects of the dielectric constant when the salt concentration is high. Combining the results of the effects of the water activity and the dielectric constant, it can be assumed that the effect of the dielectric constant is overcome by the effect of the water activity under near-saturating salt concentrations. Because the physiological salt concentration is not too high or too low, the effects of both the low water activity and low dielectric constant of the intracellular milieu are likely to influence the nucleic acid interactions in cells. It is speculated that the intracellular environment is the condition that facilitates the formation of nucleic acid structures accompanied by dehydration and cation binding, including non-canonical structures and tertiary structures that are often related to cellular functions.
6. Conclusions
Results from the model experimental systems have revealed significant effects of the intracellular environment on the hybridization, higher-order folding and catalytic activity of nucleic acids. Several environmental factors that affect the nucleic acid interactions have been identified; the excluded volume, chemical interactions and solvent properties. These environmental effects would provide mechanisms for regulating the nucleic acid structures and functions in cells. The magnitudes of the effects of these factors are different depending on the length and conformations of the nucleic acids, the contributions of metal ions and water and the concentration of salts. Table 1 summarizes the reports about the catalytic activities of several types of RNA-cleaving ribozymes with different sizes, catalytically-active structures and reaction mechanisms. The enhancements of the catalytic activities are driven by an increased folding stability and metal ion binding under the conditions of macromolecular crowding, low water activity and low dielectric constant, occurring in cells and possibly in prebiotic environments. It is fascinating to speculate that the environmental effects promote the evolution of functional RNAs and the emergence of an RNA world.101
Table 1 RNA-cleaving activities of several types of ribozymes studied using model experimental systems
Experimental system |
Salt condition |
Catalytic activitya |
Values of the catalytic activity were evaluated from the results for the rate constant, initial velocity, or amplitude of the reactions.
The data for amide compounds known as nucleic acid denaturants, such as urea and formamide, are excluded due to their strong specific interactions with RNA.
|
Macromolecular crowding by polymer cosolutes |
Full-length hammerhead ribozyme in 20 w/v% PEG solutions56 |
10 mM MgCl2 |
Increased by ∼5-fold |
0.5 mM MgCl2 |
Increased by ∼100-fold |
|
Minimum hammerhead ribozyme motif in 20 w/v% PEG solutions56,59,61 |
3–50 mM MgCl2 |
Increased by 2–10-fold |
5 mM MgCl2 with 32 mM anion |
Increased by 1.3–2-fold |
1.5–0.3 M NaCl |
Increased by 1.7–35-fold |
|
Minimum hammerhead ribozyme motif in 20 w/v% polysaccharide solutions56,59,61 |
10 mM MgCl2 |
Increased by 2–3-fold |
5 mM MgCl2 with 32 mM anion |
No significant change |
1.5 M NaCl |
No significant change |
|
HDV-like CPEB3 ribozyme in 30 w/v% PEG solutions57 |
10 mM MgCl2 |
No significant change |
0.5 and 0.1 mM MgCl2 |
Increased by 2–5.5-fold |
|
HDV-like CPEB3 ribozyme in 30 w/v% polysaccharide solutions57 |
10 mM MgCl2 |
No significant change |
0.5 and 0.1 mM MgCl2 |
Increased by 1.5–3-fold |
|
Hairpin ribozyme in 20 w/v% PEG solutions58 |
10 mM MgCl2 |
No significant change |
1 mM MgCl2 |
Increased by up to ∼3-fold |
|
Azoarcus group I ribozyme in 4–16 w/v% PEG solutions60 |
5 mM MgCl2 or less |
Increased by up to ∼70-fold |
|
Mixed aqueous solutions with organic solventsb |
Minimum hammerhead ribozyme motif in various types of 20 w/v% solutions56,59,100 |
10 mM MgCl2 |
Increased by 2–5-fold |
1 mM MgCl2 |
Increased by 2–17-fold |
1.5 M NaCl |
Increased by 0.7–1.5-fold |
|
HDV-like CPEB3 ribozyme in 30 w/v% PEG low-molecular-weight PEG solutions57 |
10 mM MgCl2 |
No significant change |
0.5 and 0.1 mM MgCl2 |
Increased by 2–5-fold |
|
Hairpin ribozyme in 2.5–10 v/v% low-molecular-weight PEG solutions94 |
1 mM MgCl2 |
Increased by up to ∼2-fold |
|
Hairpin ribozyme in 5 and 20 v/v% ethanol solutions94 |
1 mM MgCl2 |
Increased by up to ∼3-fold |
|
Lead-dependent ribozyme in 2.5–10 v/v% low-molecular-weight PEG solutions95 |
25 mM Pb(CH3COO)2 |
Increased by up to ∼2-fold |
Although the experimental systems do not adequately mimic the environments in cells, the approaches are useful to bridge the gap between the behaviors of nucleic acids in vitro and in vivo. The experimental data might also be eventually valuable for the production of synthetic cells.102 The quantitative data further provide insights into nucleic acid interactions under various non-diluted aqueous conditions developed for technological applications of nucleic acids. More biophysical experiments using sophisticated model systems will be helpful to fully understand and develop the predictions of the effects of molecular environments on nucleic acid interactions.
Acknowledgements
This work was supported in part by Grants-in-Aid for Scientific Research from JSPS (Japan Society for the Promotion of Science), MEXT (Ministry of Education, Culture, Sports, Science and Technology)-Supported Program for the Strategic Research Foundation at Private Universities (2014–2019), Japan, and the Hirao Taro Foundation of KONAN GAKUEN for Academic Research.
Notes and references
- D. K. Sasmal, S. Ghosh, A. K. Das and K. Bhattacharyya, Langmuir, 2013, 29, 2289–2298 Search PubMed.
- F. X. Theillet, A. Binolfi, T. Frembgen-Kesner, K. Hingorani, M. Sarkar, C. Kyne, C. Li, P. B. Crowley, L. Gierasch, G. J. Pielak, A. H. Elcock, A. Gershenson and P. Selenko, Chem. Rev., 2014, 114, 6661–6714 Search PubMed.
- C. P. Donahue, R. S. Yadava, S. M. Nesbitt and M. J. Fedor, J. Mol. Biol., 2000, 295, 693–707 Search PubMed.
- I. Schoen, H. Krammer and D. Braun, Proc. Natl. Acad. Sci. U. S. A., 2009, 106, 21649–21654 Search PubMed.
- E. M. Mahen, P. Y. Watson, J. W. Cottrell and M. J. Fedor, PLoS Biol., 2010, 8, e1000307 Search PubMed.
- B. Meyer, O. Benichou, Y. Kafri and R. Voituriez, Biophys. J., 2012, 102, 2186–2191 Search PubMed.
- J. Tyrrell, J. L. McGinnis, K. M. Weeks and G. J. Pielak, Biochemistry, 2013, 52, 8777–8785 Search PubMed.
- M. Gao, D. Gnutt, A. Orban, B. Appel, F. Righetti, R. Winter, F. Narberhaus, S. Muller and S. Ebbinghaus, Angew. Chem., Int. Ed., 2016, 55, 3224–3228 Search PubMed.
- R. Harada, Y. Sugita and M. Feig, J. Am. Chem. Soc., 2012, 134, 4842–4849 Search PubMed.
- T. Frembgen-Kesner and A. H. Elcock, Biophys. Rev., 2013, 5, 109–119 Search PubMed.
- J. S. Kim and I. Szleifer, Int. Rev. Cell Mol. Biol., 2014, 307, 73–108 Search PubMed.
- M. Feig, R. Harada, T. Mori, I. Yu, K. Takahashi and Y. Sugita, J. Mol. Graphics Modell., 2015, 58, 1–9 Search PubMed.
- I. M. Kuznetsova, B. Y. Zaslavsky, L. Breydo, K. K. Turoverov and V. N. Uversky, Molecules, 2015, 20, 1377–1409 Search PubMed.
- R. J. Ellis, Curr. Opin. Struct. Biol., 2001, 11, 114–119 Search PubMed.
- D. Leipply, D. Lambert and D. E. Draper, Methods Enzymol., 2009, 469, 433–463 Search PubMed.
- S. Nakano, D. Miyoshi and N. Sugimoto, Chem. Rev., 2014, 114, 2733–2758 Search PubMed.
- S. Klumpp, M. Scott, S. Pedersen and T. Hwa, Proc. Natl. Acad. Sci. U. S. A., 2013, 110, 16754–16759 Search PubMed.
- M. Tabaka, T. Kalwarczyk and R. Holyst, Nucleic Acids Res., 2014, 42, 727–738 Search PubMed.
- H. Matsuda, G. G. Putzel, V. Backman and I. Szleifer, Biophys. J., 2014, 106, 1801–1810 Search PubMed.
- F. Lang, J. Am. Coll. Nutr., 2007, 26, 613S–623S Search PubMed.
- A. P. Minton, Methods Enzymol., 1998, 295, 127–149 Search PubMed.
- T. Misteli, Histochem. Cell Biol., 2008, 129, 5–11 Search PubMed.
- M. R. Reichl and D. Braun, J. Am. Chem. Soc., 2014, 136, 15955–15960 Search PubMed.
- A. Mondal and A. Bhattacherjee, Nucleic Acids Res., 2015, 43, 9176–9186 Search PubMed.
- M. J. Morelli, R. J. Allen and P. R. Wolde, Biophys. J., 2011, 101, 2882–2891 Search PubMed.
- A. D. Dupuy and D. M. Engelman, Proc. Natl. Acad. Sci. U. S. A., 2008, 105, 2848–2852 Search PubMed.
- G. Guigas and M. Weiss, Biochim. Biophys. Acta, Biomembr., 2016, 1858, 2441–2450 Search PubMed.
- R. Hancock, J. Struct. Biol., 2004, 146, 281–290 Search PubMed.
- M. Buenemann and P. Lenz, PLoS One, 2010, 5, e13806 Search PubMed.
- P. H. Yancey, J. Exp. Biol., 2005, 208, 2819–2830 Search PubMed.
- A. Bandyopadhyay, K. Saxena, N. Kasturia, V. Dalal, N. Bhatt, A. Rajkumar, S. Maity, S. Sengupta and K. Chakraborty, Nat. Chem. Biol., 2012, 8, 238–245 Search PubMed.
- J. Hong, M. W. Capp, C. F. Anderson, R. M. Saecker, D. J. Felitsky, M. W. Anderson and M. T. Record, Jr., Biochemistry, 2004, 43, 14744–14758 Search PubMed.
- D. Lambert and D. E. Draper, J. Mol. Biol., 2007, 370, 993–1005 Search PubMed.
- D. Lambert, D. Leipply and D. E. Draper, J. Mol. Biol., 2010, 404, 138–157 Search PubMed.
- P. M. Wiggins, Microbiol. Rev., 1990, 54, 432–449 Search PubMed.
- C. F. Anderson and M. T. Record, Jr., Annu. Rev. Phys. Chem., 1995, 46, 657–700 Search PubMed.
-
N. V. Hud, Nucleic acid-metal ion interactions, RSC Publishing, 2008 Search PubMed.
- V. Conti Nibali and M. Havenith, J. Am. Chem. Soc., 2014, 136, 12800–12807 Search PubMed.
- W. Wang, K. Foley, X. Shan, S. Wang, S. Eaton, V. J. Nagaraj, P. Wiktor, U. Patel and N. Tao, Nat. Chem., 2011, 3, 249–255 Search PubMed.
-
H. Tateishi-Karimata, S. Nakano and N. Sugimoto, Curr. Protoc. Nucleic Acid Chem., 2013, ch. 7, unit 7.19 Search PubMed.
- H. X. Zhou, G. Rivas and A. P. Minton, Annu. Rev. Biophys., 2008, 37, 375–397 Search PubMed.
- I. M. Kuznetsova, K. K. Turoverov and V. N. Uversky, Int. J. Mol. Sci., 2014, 15, 23090–23140 Search PubMed.
- A. Soranno, I. Koenig, M. B. Borgia, H. Hofmann, F. Zosel, D. Nettels and B. Schuler, Proc. Natl. Acad. Sci. U. S. A., 2014, 111, 4874–4879 Search PubMed.
- J. Batra, K. Xu and H. X. Zhou, Proteins, 2009, 77, 133–138 Search PubMed.
- J. Tyrrell, K. M. Weeks and G. J. Pielak, Biochemistry, 2015, 54, 6447–6453 Search PubMed.
- V. A. Bloomfield, Curr. Opin. Struct. Biol., 1996, 6, 334–341 Search PubMed.
- J. E. Ramos, Jr., J. R. Neto and R. de Vries, J. Chem. Phys., 2008, 129, 185102 Search PubMed.
- D. Kilburn, J. H. Roh, L. Guo, R. M. Briber and S. A. Woodson, J. Am. Chem. Soc., 2010, 132, 8690–8696 Search PubMed.
- C. A. Strulson, J. A. Boyer, E. E. Whitman and P. C. Bevilacqua, RNA, 2014, 20, 331–347 Search PubMed.
- C. A. Strulson, R. C. Molden, C. D. Keating and P. C. Bevilacqua, Nat. Chem., 2012, 4, 941–946 Search PubMed.
- H. T. Lee, D. Kilburn, R. Behrouzi, R. M. Briber and S. A. Woodson, Nucleic Acids Res., 2015, 43, 1170–1176 Search PubMed.
- S. Nakano, H. Karimata, T. Ohmichi, J. Kawakami and N. Sugimoto, J. Am. Chem. Soc., 2004, 126, 14330–14331 Search PubMed.
- S. Nakano, L. Wu, H. Oka, H. T. Karimata, T. Kirihata, Y. Sato, S. Fujii, H. Sakai, M. Kuwahara, H. Sawai and N. Sugimoto, Mol. BioSyst., 2008, 4, 579–588 Search PubMed.
- S. Nakano, H. Hirayama, D. Miyoshi and N. Sugimoto, J. Phys. Chem. B, 2012, 116, 7406–7415 Search PubMed.
- L. E. Baltierra-Jasso, M. J. Morten, L. Laflor, S. D. Quinn and S. W. Magennis, J. Am. Chem. Soc., 2015, 137, 16020–16023 Search PubMed.
- S. Nakano, H. T. Karimata, Y. Kitagawa and N. Sugimoto, J. Am. Chem. Soc., 2009, 131, 16881–16888 Search PubMed.
- C. A. Strulson, N. H. Yennawar, R. P. Rambo and P. C. Bevilacqua, Biochemistry, 2013, 52, 8187–8197 Search PubMed.
- B. P. Paudel and D. Rueda, J. Am. Chem. Soc., 2014, 136, 16700–16703 Search PubMed.
- S. Nakano, Y. Kitagawa, D. Miyoshi and N. Sugimoto, FEBS Open Bio, 2014, 4, 643–650 Search PubMed.
- R. Desai, D. Kilburn, H. T. Lee and S. A. Woodson, J. Biol. Chem., 2014, 289, 2972–2977 Search PubMed.
- S. Nakano, Y. Kitagawa, D. Miyoshi and N. Sugimoto, J. Biol. Inorg. Chem., 2015, 20, 1049–1058 CrossRef CAS PubMed.
- R. M. Elder, J. Pfaendtner and A. Jayaraman, Biomacromolecules, 2015, 16, 1862–1869 Search PubMed.
- K. C. Lin, M. T. Wey, L. S. Kan and D. Shiuan, Appl. Biochem. Biotechnol., 2009, 158, 631–641 Search PubMed.
- K. Yoshikawa, S. Hirota, N. Makita and Y. Yoshikawa, J. Phys. Chem. Lett., 2010, 1, 1763–1766 Search PubMed.
- A. R. Denton, Int. Rev. Cell Mol. Biol., 2014, 307, 27–71 Search PubMed.
- A. Zinchenko, K. Tsumoto, S. Murata and K. Yoshikawa, J. Phys. Chem. B, 2014, 118, 1256–1262 Search PubMed.
- H. Kamata, A. Zinchenko and S. Murata, Colloid Polym. Sci., 2011, 289, 1329–1335 CAS.
- F. Anella and C. Danelon, Life, 2014, 4, 929–943 Search PubMed.
- H. X. Zhou and K. A. Dill, Biochemistry, 2001, 40, 11289–11293 Search PubMed.
- J. Mittal and R. B. Best, Proc. Natl. Acad. Sci. U. S. A., 2008, 105, 20233–20238 Search PubMed.
- S. Nakano, M. Yoshida, D. Yamaguchi and N. Sugimoto, Trans. Mater. Res. Soc. Jpn., 2014, 39, 435–438 Search PubMed.
- E. Bismuto and G. Irace, FEBS Lett., 2001, 509, 476–480 Search PubMed.
- E. Bismuto, P. L. Martelli, A. De Maio, D. G. Mita, G. Irace and R. Casadio, Biopolymers, 2002, 67, 85–95 Search PubMed.
- D. Bolis, A. S. Politou, G. Kelly, A. Pastore and P. A. Temussi, J. Mol. Biol., 2004, 336, 203–212 CrossRef CAS PubMed.
- T. Johnson, J. Zhu and R. M. Wartell, Biochemistry, 1998, 37, 12343–12350 Search PubMed.
- E. A. Frankel, P. C. Bevilacqua and C. D. Keating, Langmuir, 2016, 32, 2041–2049 Search PubMed.
- H. Workman and P. F. Flynn, J. Am. Chem. Soc., 2009, 131, 3806–3807 Search PubMed.
- S. Pramanik, S. Nagatoishi and N. Sugimoto, Chem. Commun., 2012, 48, 4815–4817 Search PubMed.
- I. A. Chen, K. Salehi-Ashtiani and J. W. Szostak, J. Am. Chem. Soc., 2005, 127, 13213–13219 Search PubMed.
- W. Reisner, J. N. Pedersen and R. H. Austin, Rep. Prog. Phys., 2012, 75, 106601 Search PubMed.
- S. M. Friedrich, H. C. Zec and T. H. Wang, Lab Chip, 2016, 16, 790–811 Search PubMed.
- C. C. Hsieh, A. Balducci and P. S. Doyle, Nano Lett., 2008, 8, 1683–1688 Search PubMed.
- C. Zhang, P. G. Shao, J. A. van Kan and J. R. van der Maarel, Proc. Natl. Acad. Sci. U. S. A., 2009, 106, 16651–16656 Search PubMed.
- J. J. Jones, J. R. van der Maarel and P. S. Doyle, Nano Lett., 2011, 11, 5047–5053 Search PubMed.
- M. Castronovo, A. Stopar, L. Coral, S. K. Redhu, M. Vidonis, V. Kumar, F. D. Ben, M. Grassi and A. W. Nicholson, Curr. Med. Chem., 2013, 20, 3539–3557 Search PubMed.
- A. Zaki, N. Dave and J. Liu, J. Am. Chem. Soc., 2012, 134, 35–38 Search PubMed.
- N. Perez-Hernandez, T. Q. Luong, C. Perez, J. D. Martin and M. Havenith, Phys. Chem. Chem. Phys., 2010, 12, 6928–6932 Search PubMed.
- S. Nakano and N. Sugimoto, Biophys. Rev., 2016, 8, 11–23 Search PubMed.
- D. B. Knowles, A. S. LaCroix, N. F. Deines, I. Shkel and M. T. Record, Jr., Proc. Natl. Acad. Sci. U. S. A., 2011, 108, 12699–12704 Search PubMed.
- R. Buscaglia, M. C. Miller, W. L. Dean, R. D. Gray, A. N. Lane, J. O. Trent and J. B. Chaires, Nucleic Acids Res., 2013, 41, 7934–7946 Search PubMed.
- E. Westhof, Annu. Rev. Biophys. Biophys. Chem., 1988, 17, 125–144 Search PubMed.
- C. H. Spink and J. B. Chaires, Biochemistry, 1999, 38, 496–508 Search PubMed.
- S. Nakano, D. Yamaguchi, H. Tateishi-Karimata, D. Miyoshi and N. Sugimoto, Biophys. J., 2012, 102, 2808–2817 Search PubMed.
- S. Tobe, T. Heams, J. Vergne, G. Herve and M. C. Maurel, Nucleic Acids Res., 2005, 33, 2557–2564 Search PubMed.
- M. Giel-Pietraszuk and J. Barciszewski, Mol. Biol. Rep., 2012, 39, 6309–6318 Search PubMed.
- S. M. Mel’niov, M. O. Khan, B. Lindman and B. Jönsson, J. Am. Chem. Soc., 1999, 121, 1130–1136 Search PubMed.
- S. Nakano, Y. Tanino, H. Hirayama and N. Sugimoto, Biophys. J., 2016, 111, 1350–1360 Search PubMed.
- I. V. Smirnov and R. H. Shafer, Biopolymers, 2007, 85, 91–101 Search PubMed.
- M. Furler, B. Knobloch and R. K. O. Sigel, Inorg. Chim. Acta, 2009, 362, 771–776 Search PubMed.
- S. Nakano, Y. Kitagawa, H. Yamashita, D. Miyoshi and N. Sugimoto, ChemBioChem, 2015, 16, 1803–1810 Search PubMed.
- J. Spitzer and B. Poolman, Microbiol. Mol. Biol. Rev., 2009, 73, 371–388 Search PubMed.
- A. Zinchenko, Adv. Colloid Interface Sci., 2016, 232, 70–79 Search PubMed.
|
This journal is © The Royal Society of Chemistry 2017 |