DOI:
10.1039/C6MB00630B
(Paper)
Mol. BioSyst., 2017,
13, 156-164
Allosteric pathways in tetrahydrofolate sensing riboswitch with dynamics correlation network†
Received
5th September 2016
, Accepted 28th October 2016
First published on 31st October 2016
Abstract
Riboswitches are cis-acting genetic control elements. Due to their fundamental importance in bacteria gene regulation, they have been proposed as antibacterial drug targets. Tetrahydrofolate (THF) is an essential cofactor of one-carbon transfer reactions and downregulates the expression of downstream genes. However, information on how to transfer from the binding site of THF to the expression platform is still unavailable. Herein, a nucleotide/nucleotide dynamics correlation network based on an all-atom molecular dynamic simulation was used to reveal the regulation mechanism of a THF-sensing riboswitch. Shortest pathway analysis based on the network illustrates that there is an allosteric pathway through the P2 helix to the pseudoknot, then to the P1 helix in the THF-riboswitch. Thus the hypothesis of “THF-binding induced allosteric switching” was proposed and evaluated using THF and pseudoknot weakened experiments. Furthermore, a possible allosteric pathway of C30–C31–G33–A34–G35–G36–G37–A38–G48–G47–U46–A90–U91–C92–G93–C94–G95–C96 was identified and confirmed through the perturbation of the network. The proposed allosteric mechanism and the underlying allosteric pathway provide fundamental insights for the regulation of THF sensing riboswitches.
Introduction
Riboswitches are RNA structures found in most bacteria, plants and fungi.1,2 Since the first TPP riboswitch3 was reported, more than 20 classes of riboswitches have been found to date.4,5 Riboswitches are typically located in the 5′-untranslated region (UTR) of mRNA and are often conceptually split into two parts: the aptamer and the expression platform.6 The aptamer domain binds to small molecules with high specificity and affinity, while the expression platform in turn supplies interfaces with many other gene expression apparatus.7 Riboswitches can regulate the activity of mRNA in transcription and translation8 levels just like regulatory ncRNAs9 in eukaryotic cells. Tetrahydrofolate (THF) is the reductive form of folate and an essential cofactor of the one-carbon transfer reaction.10 Genes involved in the transportation and biosynthesis of folate and its derivatives are always controlled by the THF riboswitch.11 In 2011, two groups reported two crystals in the THF riboswitch. Batey et al. revealed that the riboswitch can bind two THF, one in the three-way junction and another in the pseudoknot.12 Patel et al. revealed a single THF binding site in the three-way junction, which was far away from the effective site.13 It is well known that allostery is a fundamental regulatory strategy adopted by many proteins and nucleic acids,14,15 which modulates the function and adaptability sensitively. Allosteric macromolecules always contain at least two functional active sites: the effector binding site and the catalytic site.16
In order to reveal the regulation mechanism of the THF riboswitch, a single THF binding site system was used in this research. The THF riboswitch structure is shown in Fig. 1. The riboswitch consists of four helices (P1, P2, P3, P4), five joining regions (J1/2, J2/1, J2/3, J3/4, J4/2), and two hairpin loops (L3, L4). Helix P1 consists of G1–G6 and C96–U100, helix P2 G11–G20 and U78–C87, helix P3 G29–G36 and C50–A57, helix P4 C62–G73, junction J1-2/J2-1 from A7 to A10 and G88 to G95, junction J2-3/J3-4/J4-2 from C21 to U28, C58–A61 and G74–G77. THF binds to helix P3 near J2/3. Helix P3 plays a crucial role in locking the ligand and sequestering the ribosome binding site.
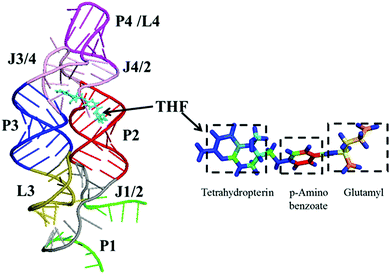 |
| Fig. 1 Ribbon representation of the NMR structure for the THF riboswitch and THF. Helices P1, P2, P3, and P4 (green, brown, blue, and purple), junctions J1/2 and J2/3/4 (gray and pink), hairpin loops L3 and L4 (yellow-green and purple), and THF (cyan) are labeled. | |
It is still unclear how the THF riboswitch downregulates gene expression. In this study, we intend to discover a regulatory pathway and the mechanism of the THF riboswitch by answering the following two questions. (1) Is there an allosteric regulation for the THF riboswitch? (2) If so, what are the allosteric pathways that connect the THF binding site and the regulating site? In order to answer these questions, community network analysis17 based on all-atom MD simulations was utilized to address each of these issues. Overall, these studies show the feasibility of using MD to understand riboswitch functions and provide an opportunity to look for common themes in ligand binding and conformational dynamics.
Dynamic network analysis, which has been previously used to illustrate the allosteric phenomenon of aminoacyl-tRNA synthetase, was utilized to elucidate the allosteric pathways of the THF sensing riboswitch.18 The pathway shows that the P3 helix and pseudoknot19 play key roles in information transfer. Mutants of G41U and G41A–G42U–U91A from the pseudoknot confirm that the pseudoknot can stabilize the base pairs of the P1 helix. From the comparisons of the networks between the free and bound riboswitches, the allosteric pathway and key bases were proposed for transferring the regulation information from the THF allosteric sites to the expression platform. The process based on an allosteric pathway might improve the regulation efficiency of downstream genes.
Materials and method
Molecular dynamics simulations
The structures of free (pdb code: 3SUY) and THF bound riboswitches (pdb code: 3SUX) were downloaded from RCSB.13 All structural visualizations were conducted in PyMOL 1.5.03. AMBER12 was used to perform efficient simulations with periodic boundary conditions. Hydrogen atoms were added using the LEaP module of AMBER12. Counter-ions were added to maintain system neutrality. All the systems were solvated in a truncated octahedron box of TIP3P waters with a buffer of 10 Å. The pairwise interactions (van der Waals and direct Coulomb) were computed with a default cutoff distance of 8 Å. Particle Mesh Ewald (PME) was utilized to treat long-range electrostatic interactions in AMBER12.20 The improved ff99SBildn21 force field was used for the intramolecular interactions. In order to compare the performance of different force fields, ff99bsc0 was also used in this study for the bound riboswitch. The SHAKE algorithm22 was used to constrain bonds involving hydrogen atoms. All MD simulations were accelerated with the CUDA version of PMEMD in GPU cores of NVIDIA® Tesla K20. Up to 10000-step steepest descent minimization was performed to relieve any structural clash in the solvated systems. This was followed by a 400 ps' heating up and a 200 ps' equilibration in the NVT ensemble at 298 K. The Langevin thermostat was used in the preparation runs with a friction constant of 1 ps−1 and the Berendsen thermostat with default settings was used in the production runs.
To compare the difference between free and bound riboswitches, four independent trajectories of 100 ns each were simulated. 1 μs trajectories in all were collected for the wild type and mutant systems at 298 K, taking about 1200 GPU hours. Detailed simulation conditions are listed in Table 1.
Table 1 Simulation conditions for all systems
System |
Temperature (K) |
Time (ns) |
Traj. No. |
Ions |
Waters |
Force field |
THF bound |
298 |
100 |
4 |
101 |
104 487 |
ff99SBildn |
THF bound |
298 |
100 |
1 |
101 |
104 487 |
ff99BSC0 |
THF free |
298 |
100 |
4 |
100 |
111 761 |
ff99SBildn |
Data analyses
RMSD and RMSF were calculated and analyzed to monitor the convergence of the system and the relative variation of nucleotides. PCA were used to monitor the motion mode of the nucleotides. Hydrophobic contacts, electrostatic (i.e. charge–charge) interactions and hydrogen bonds were used to identify the intramolecular interaction between the THF and riboswitch. A hydrogen bond is defined when the distance between two polar heavy atoms, at least one of which has a hydrogen atom, is less than 3.5 Å. For each simulation, sampling was conducted every 10 ps (10
000 snapshots for 100 ns' simulations). These interactions were handled with in-house software. All pictures were plotted using Origin 9.1.
Dynamics network analyses
Every nucleotide was divided into two nodes (backbone and base) for more detailed analysis of their fluctuation dynamics. The fluctuation correlation between any pair of nodes was calculated as
where Δ
i(t) =
i(t) − 〈
i(t)〉,
i(t), is the position of node i at time t, and 〈·〉 represents the time average. These elements were conveniently organized as a covariance matrix for a simulated system. In the current study, the covariance matrix for each system was constructed using snapshots (every 2 ps) in the last 50 ns of all simulated trajectories. Besides nodes, an “edge” that transfers allosteric information from one node to another is also a key concept in network construction. An edge is defined between any two nodes without a covalent bond but with heavy atoms closer than 4.5 Å over 75% of the sampling time. The strength of the edge is defined as the absolute value of the inter-node correlation (Cij) between nodes i and j. The number of connected edges at each node is defined as the degree of the node. Correlation-weighted degree, which is the summation of strengths of all edges connected to a given node, indicates the importance of the node. After the network construction, network topological analyses were performed using Cytoscape3.2.0.23 The Floyd–Warshall algorithm was used to calculate the shortest path between any two nodes in the network.24 For community analyses, the Girvan–Newman algorithm was utilized with the network tools developed by the Luthey-Schulten group18,24 and the strategy of the process was successfully used in the allostery of the riboswitch and protein.25,26
Results
THF stabilizing P1 helix of the riboswitch
The Cα RMSDs of THF free and bound riboswitches are shown in the ESI,† Fig. S1. This figure indicates that 100 ns simulations are sufficient for the equilibration of these systems. The RMSF of THF free and bound riboswitches are shown in Fig. 2. This figure shows that the fluctuation of the bound riboswitch is significantly lower than that of the free riboswitch, especially on the regions of the P1 helix (1–6, 90–100) and P2 helix (40–50). This shows that the binding of THF can stabilize the P1 and P2 helices.
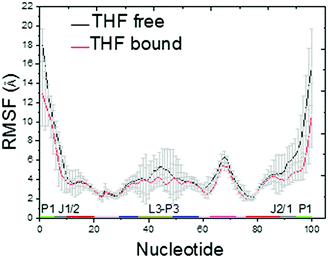 |
| Fig. 2 C5′ RMSF for the THF bound and free states. | |
In order to further evaluate the conformational adjustment of the riboswitch, the pairwise C5′ distance difference between the THF bound and free riboswitches was measured. Fig. 3 illustrates the conformational adjustment of the riboswitch upon the binding of THF. The green regions represent negative differences, indicating a more compact structure, while the red and blue regions indicate C5′ atoms pushed further away (a more extended structure). In this figure, the red and blue regions are located at nucleotides 90–100 and 38–43, suggesting that the P1 helix and pseudoknot move further away from the main domain upon the disassociation of THF. Consistent observations were also found in the principal component analysis (PCA) as discussed below.
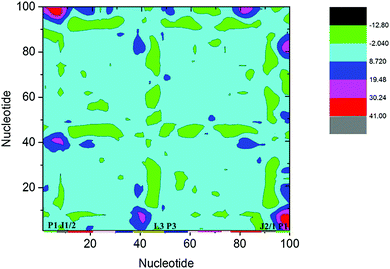 |
| Fig. 3 Distance different landscape between the THF bound and free states. The green regions represent negative differences, and the red and blue regions represent positive differences. | |
The distance different landscape indicates that the P1 helix undergoes significant conformational changes for the THF free riboswitch. To quantitatively identify the motion mode for the conformational adjustment, PCA was carried out on the THF bound and free systems. Overall, the three most dominant components (named PC1, PC2, and PC3) represent most of the overall fluctuations. To clearly display the motion of the P1 helix, structural projections along the first principal component (∼40%) are shown in Fig. 4. For the THF free riboswitch, the P1 helix is in the opening and closing motion. Pseudoknot L3 also has motion far away from the P1 helix. However, the THF bound riboswitch has no significant conformational changes. This indicates that the P1 helix was stabilized by THF binding.
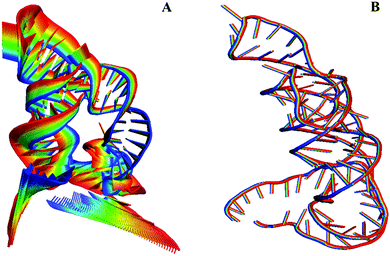 |
| Fig. 4 Motion mode of PCA analysis for the THF bound and free states. (A) THF free state. (B) THF bound state. | |
The alignment of average structures between the THF bound and free riboswitch is shown in Fig. 5. The most significant difference was found at the regions of the P1 helix and pseudoknot. In order to more clearly highlight this difference, the distances between G6 and C96, between A7 and G95 at the P1 helix are shown in Fig. 5B and C. The distance between G6 and C96 for the free riboswitch is about 33 Å and 18 Å for the bound riboswitch. The distance between A7 and G95 is about 30 Å for the THF free riboswitch and 16 Å for the THF bound riboswitch. These results also clearly indicate that the P1 helix has significant opening propensity upon the disassociation of THF.
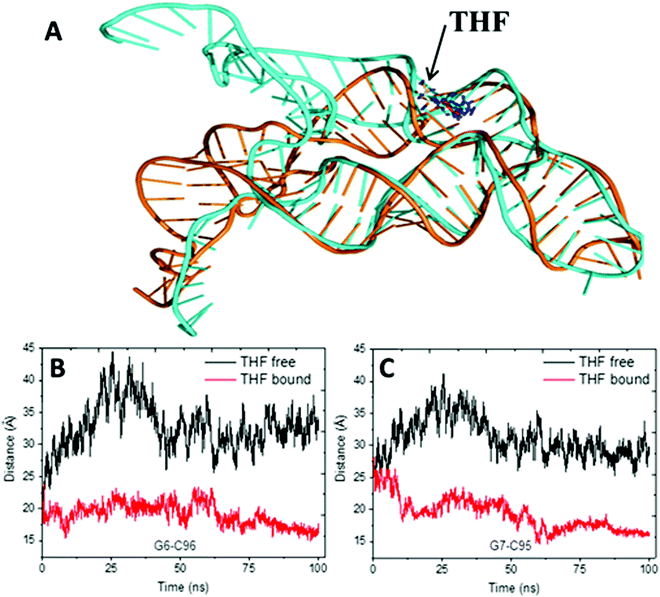 |
| Fig. 5 The alignment of the average structures for the THF free and bound riboswitch, and the distance for two pairs of the terminus base. (A) Alignment of the average structures. Cyan represents the THF free riboswitch and orange represents the bound riboswitch. (B) Distances between G6–C96. (C) Distance between G7 and G95. | |
The previous work suggests that the P1 helix will sequester the ribosome binding site (RBS) and down-regulate the production of proteins involving folate synthesis and transportation.13 Furthermore, the P1 helix is about 50 Å away from the THF binding site. Therefore, this might be an allosteric regulation in this system.
Different correlation networks for THF free and bound riboswitches
Structural analysis suggests that allosteric regulation may exist in the THF bound riboswitch. In order to reveal the allosteric molecular mechanism, a dynamics network was used to analyze the nucleotide fluctuation correlation. To construct the correlation network, the covariance matrices were first calculated for the riboswitch. The dynamics cross-correlation maps (DCCMs) for THF free and bound riboswitches are shown in Fig. 6. Positive and negative correlations are shown in the upper and lower traiangles of the DCCM, respectively. This figure indicates that the correlations between P3 and P1 are much lower in the THF bound state than those in the THF free state. These regions are more compact in the THF bound state. The correlation difference between the THF bound and free states is mainly focused on the P3 and P1 helices. This demonstrates that the P3 helix is strongly correlated with P1 upon THF binding.
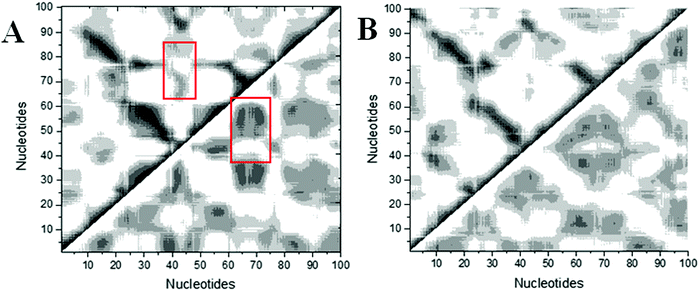 |
| Fig. 6 DCCMs for the THF bound and free states. (A) THF bound. (B) THF free. The strong (Cij = ±0.5–1.0), and weak (Cij = ±0.2–0.5) are represented by black and gray, respectively. The lower and upper triangles correspond to negative and positive correlations, respectively. | |
Based on the covariance matrices, dynamics networks were constructed. The topology parameters of the THF bound and free networks are listed in Table 2. The network centralization of the THF bound state is higher than that of the free state. The dynamics networks for the THF free and bound states are shown in Fig. 7. The number of nodes with a weighted degree higher than five is 32 for the THF bound state and more than that of the THF free state (28). These important hub nodes might play key roles in the network, such as G27, U28, C30, C51, G48, G37, G98, and other nodes. Furthermore, six bases such as G37, A38, C39, C39, G41, G48, and U49 belong to the pseudoknot of the THF bound riboswitch. Interestingly, the degrees of G98, G95, C50, C51, and C75 significantly decrease upon the dissociation of THF. For the whole network, the THF free network splits into two pieces. The information flow could not transfer from the allosteric site to the P1 helix.
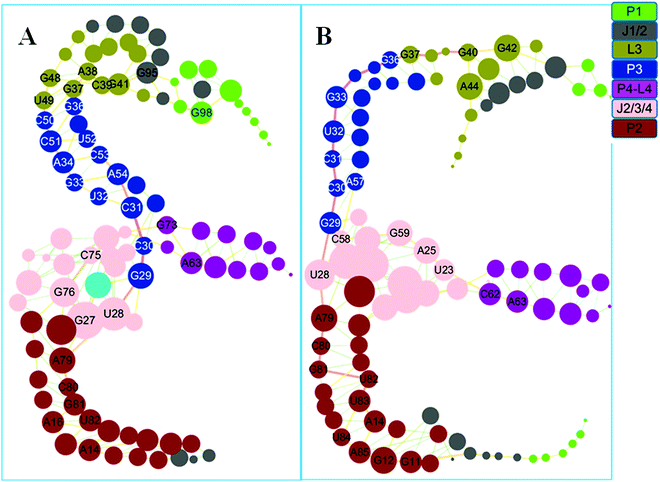 |
| Fig. 7 Dynamics correlation networks for THF bound and free riboswitches. (A) THF bound riboswitch. (B): THF free riboswitch. Nodes are drawn to size based on the actual values for degree and are colored according to their structural domains. See labels in the coloring legend. Hub nodes with degrees higher than 5 are labeled with the bases. | |
Table 2 Topology parameters of THF bound and free networks
System |
Clustering coefficient |
Network density |
Network centralization |
Network heterogeneity |
Avg. number of neighbors |
THF bound |
0.503 |
0.042 |
0.050 |
0.303 |
4.16 |
THF free |
0.428 |
0.041 |
0.042 |
0.39 |
4.00 |
Different communities for different networks
The dynamics correlation network shows that the networks are significantly different between the THF bound and free riboswitches. To reveal the information transfer pathway, the Girvan–Newman algorithm was used to split the network into communities. The community network is shown in Fig. 8, which shows that the THF bound network is clustered into seven connective communities. The information flow can freely transfer from the binding pocket of THF to the expression platform of the P1 helix. However, the community network is significantly changed and the P1 helix and L3 loop are isolated from the main community for the THF free state. Information flow can hardly transfer from the binding site to the expression platform. These results are in agreement with those of the dynamics network analysis (shown in Fig. 7). Note too, that the information intensity of the bound community network is also stronger than that of the free community network, especially for the community of the binding pocket. Moreover, the structural analysis indicates that there are two hydrogen bonds, four hydrophobic interactions, and eight electrostatic interactions between the THF and riboswitch, with a population higher than 50% (shown in Fig. 9). These results indicate fairly strong binding interactions. Therefore, a hypothesis of “binding induced allosteric switching” can be used to explain the regulation mode for the THF riboswitch.
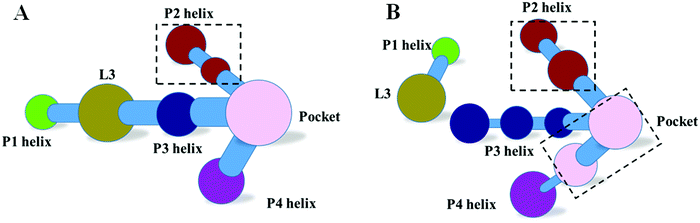 |
| Fig. 8 Community networks for the THF bound and free states. (A) THF bound. (B) THF free. | |
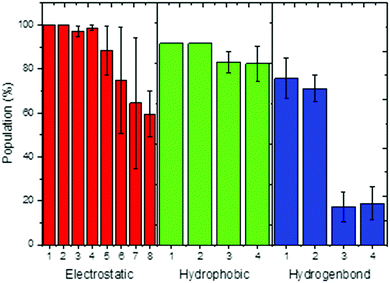 |
| Fig. 9 Interactions between THF and the riboswitch. Electrostatic interaction, 1:G59/THF,2:G77/THF,3:G29/THF,4:A60/THF,5:C30/THF,6:A26/THF,7:U28/THF,8:G27/THF; hydrophobic interaction, 1:U28/THF, 2:G29/THF, 3:C58/THF, 4:G59/THF; hydrogen bond, 1:U28/THF, 2:C58/THF, 3:G77/THF, 4:G29/THF. | |
Validation of the hypothesis through network modification
In order to validate the above hypothesis, network modification to weaken the interactions between the THF and the riboswitch was used to study the effects on the community networks. This perturbation just deleted the edges between THF and the riboswitch nodes in the network. The community network of the weakened system is shown in Fig. 10. The number of communities for the weakening operation did not change, however the nucleotides within different communities were rearranged. In summary, this modification leads to significant repartition of the community network. This finding confirms that the interactions between the THF and riboswitch indeed influence the community network and further supports our hypothesis of the binding induced allosteric switching mechanism.
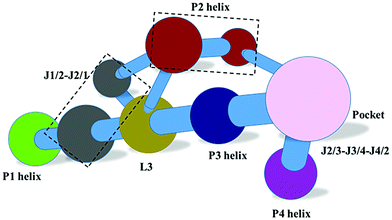 |
| Fig. 10 Community network for the THF weakened system. | |
Validation of the hypothesis through pseudoknot weakening
Pseudoknots are essential for various cellular activities by forming integral parts of the RNA structures.27–29 Previous studies confirmed that the pseudoknot forms key tertiary pairs in ydao-riboswitches.30,31 Furthermore, the community of the L3 pseudoknot was between the P1 helix and the P3 helix and plays a key role in information transfer. To further evaluate the hypothesis of “binding induced allosteric switching”, the communities of weakened pseudoknots for G41/G95 and G41/G42/G95 were constructed and are shown in Fig. 11. This figure indicates that the P1 helix was split into one isolated community and had no information exchange with L3 and the main part for the weakened G41/G95 and G41/G42/G95. Therefore, a weakened pseudoknot significantly changes the correlation community and confirms the hypothesis.
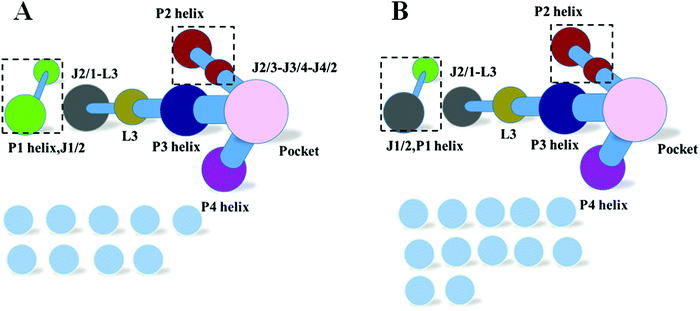 |
| Fig. 11 Community network for the weakened pseudoknot. (A) Weakened G41/G95. (B) Weakened G41/G42/G95. | |
THF allosteric pathway
The network and community analyses of the wild type and mutants confirm that the THF binding induces allostery of the riboswitch. Next, it is natural to identify the allosteric pathway based on the shortest path analysis between the allosteric site and the expression platform of the P1 helix. One pathway was identified in the THF bound riboswitch: C30–C31–G33–A34–G35–G36–G37–A38–G48–G47–U46–A90–U91–C92–G93–C94–G95–C96 (Fig. 12). However, we could not find a similar pathway in the THF free riboswitch. This indicates that these nucleotides are key nodes for information transfer in the THF bound riboswitch.
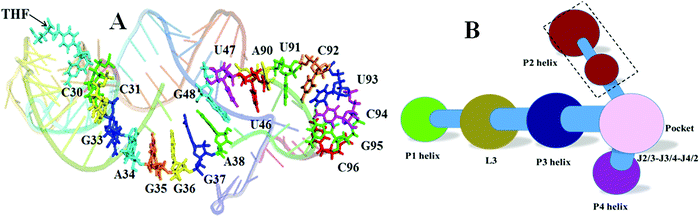 |
| Fig. 12 Allosteric pathway and community network for the weakened system. (A) Allosteric pathway. (B) Community network for weakened G48. | |
In order to further verify the allosteric pathway, network perturbation was used to study the effect of G48, which is the linkage node between the P3 helix and the L3 junction, the bottleneck of information transfer. Without carrying out another MD simulation, the perturbation was realized by weakening the edges between G48 and any nucleotide in the network. The community network is shown in Fig. 12B. There are 14 communities, more than those (11) of the THF bound system. These communities are significantly repartitioned. This weakening process decreases the effectiveness of information transfer, confirming that G48 plays a key role in this allosteric switch.
Discussion
Comparison with previous studies
The crystal structure shows that U28 and C58 can form stable hydrogen bonds with pterin of THF.13 Two stable hydrogen bonds for U28/THF and C58/THF were found in the room-temperature MD simulations. These results are in good agreement with the structural analysis that THF forms important interactions with the riboswitch. The experimental data also indicate that the triple stacks of G29–THF–G59 can stabilize the binding pocket, which is consistent with our simulations that THF can form stable hydrophobic interactions with G29 and G59 (Fig. 9). The previous experiment also suggests that pseudoknot interactions can stabilize helix P1.13 The networks and communities of pseudoknot mutants suggest that the information flow fails to transfer within the riboswitch. This indicates that the pseudoknot plays an essential role in information transfer. This is consistent with the experimental observations.
Conclusion
A dynamics correlation network was used to research the allosteric mechanism of THF riboswitches. The results suggest that the correlation network of the THF bound riboswitch has more hub nodes than that of the THF free state. The community network of the bound state is clustered into a union community. However, the P1 helix and the L3 junction are isolated from the community upon dissociation from THF. The information flow can freely transfer from the binding pocket of THF to the expression platform of the P1 helix. On the contrary, information flow can hardly transfer from the J2/3/4 junction to the expression platform in the THF free state. Therefore, a hypothesis of “THF-binding induced allosteric switching” is used to explain the THF binding and switch regulation. These observations were further confirmed by the results from community analysis based on the Girvan–Newman algorithm for pseudoknot and THF weakened networks. Finally, a possible allosteric pathway of C30–C31–G33–A34–G35–G36–G37–A38–G48–G47–U46–A90–U91–C92–G93–C94–G95–C96 was also identified based on the analysis of the network for the THF bound state and confirmed through network perturbation. Interestingly, no pathways could be found in the THF free state.
Acknowledgements
This work was supported by the Center for HPC at Shanghai Jiao Tong University, the National High-tech R&D Program of China (863 Program) (2014AA021502), the National Natural Science Foundation of China (31620103901 and J1210047), the Medical Engineering Cross Fund of Shanghai Jiaotong University (YG2013MS68, YG2014MS47, and YG2015MS56), and the National Institutes of Health/NIGMS (GM093040 & GM079383).
References
- E. R. Lee, J. L. Baker, Z. Weinberg, N. Sudarsan and R. R. Breaker, An allosteric self-splicing ribozyme triggered by a bacterial second messenger, Science, 2010, 329, 845–848 Search PubMed.
- G. T. Nguyen, M. A. Scaife, K. E. Helliwell and A. G. Smith, Role of riboswitches in gene regulation and their potential for algal biotechnology, J. Phycol., 2016, 52, 320–328 Search PubMed.
- A. Haller, R. B. Altman, M. F. Souliere, S. C. Blanchard and R. Micura, Folding and ligand recognition of the TPP riboswitch aptamer at single-molecule resolution, Proc. Natl. Acad. Sci. U. S. A., 2013, 110, 4188–4193 Search PubMed.
- A. Roth and R. R. Breaker, The structural and functional diversity of metabolite-binding riboswitches, Annu. Rev. Biochem., 2009, 78, 305–334 Search PubMed.
- W. Winkler, A. Nahvi and R. R. Breaker, Thiamine derivatives bind messenger RNAs directly to regulate bacterial gene expression, Nature, 2002, 419, 952–956 Search PubMed.
- K. D. Smith, S. V. Lipchock, T. D. Ames, J. Wang, R. R. Breaker and S. A. Strobel, Structural basis of ligand binding by a c-di-GMP riboswitch, Nat. Struct. Mol. Biol., 2009, 16, 1218–1223 Search PubMed.
- E. E. Regulski, R. H. Moy, Z. Weinberg, J. E. Barrick, Z. Yao, W. L. Ruzzo and R. R. Breaker, A widespread riboswitch candidate that controls bacterial genes involved in molybdenum cofactor and tungsten cofactor metabolism, Mol. Microbiol., 2008, 68, 918–932 Search PubMed.
- A. Serganov, Y. R. Yuan, O. Pikovskaya, A. Polonskaia, L. Malinina, A. T. Phan, C. Hobartner, R. Micura, R. R. Breaker and D. J. Patel, Structural basis for discriminative regulation of gene expression by adenine- and guanine-sensing mRNAs, Chem. Biol., 2004, 11, 1729–1741 Search PubMed.
- D. Holoch and D. Moazed, RNA-mediated epigenetic regulation of gene expression, Nat. Rev. Genet., 2015, 16, 71–84 Search PubMed.
- V. de Crecy-Lagard, B. El Yacoubi, R. D. de la Garza, A. Noiriel and A. D. Hanson, Comparative genomics of bacterial and plant folate synthesis and salvage: predictions and validations, BMC Genomics, 2007, 8, 245 Search PubMed.
- T. D. Ames, D. A. Rodionov, Z. Weinberg and R. R. Breaker, A eubacterial riboswitch class that senses the coenzyme tetrahydrofolate, Chem. Biol., 2010, 17, 681–685 Search PubMed.
- J. J. Trausch, P. Ceres, F. E. Reyes and R. T. Batey, The structure of a tetrahydrofolate-sensing riboswitch reveals two ligand binding sites in a single aptamer, Structure, 2011, 19, 1413–1423 CrossRef CAS PubMed.
- L. Huang, S. Ishibe-Murakami, D. J. Patel and A. Serganov, Long-range pseudoknot interactions dictate the regulatory response in the tetrahydrofolate riboswitch, Proc. Natl. Acad. Sci. U. S. A., 2011, 108, 14801–14806 Search PubMed.
- D. Wootten, A. Christopoulos and P. M. Sexton, Emerging paradigms in GPCR allostery: implications for drug discovery, Nat. Rev. Drug Discovery, 2013, 12, 630–644 Search PubMed.
- R. Penchovsky and R. R. Breaker, Computational design and experimental validation of oligonucleotide-sensing allosteric ribozymes, Nat. Biotechnol., 2005, 23, 1424–1433 Search PubMed.
- I. Rivalta, M. M. Sultan, N. S. Lee, G. A. Manley, J. P. Loria and V. S. Batista, Allosteric pathways in imidazole glycerol phosphate synthase, Proc. Natl. Acad. Sci. U. S. A., 2012, 109, E1428–E1436 Search PubMed.
- M. Girvan and M. E. Newman, Community structure in social and biological networks, Proc. Natl. Acad. Sci. U. S. A., 2002, 99, 7821–7826 Search PubMed.
- A. Sethi, J. Eargle, A. A. Black and Z. Luthey-Schulten, Dynamical networks in tRNA:protein complexes, Proc. Natl. Acad. Sci. U. S. A., 2009, 106, 6620–6625 Search PubMed.
- J. D. Puglisi, J. R. Wyatt and I. Tinoco, Jr., A pseudoknotted RNA oligonucleotide, Nature, 1988, 331, 283–286 Search PubMed.
- D. A. Case, T. E. Cheatham, 3rd, T. Darden, H. Gohlke, R. Luo, K. M. Merz, Jr., A. Onufriev, C. Simmerling, B. Wang and R. J. Woods, The Amber biomolecular simulation programs, J. Comput. Chem., 2005, 26, 1668–1688 Search PubMed.
- W. Ye, D. Ji, W. Wang, R. Luo and H. F. Chen, Test and Evaluation of ff99IDPs Force Field for Intrinsically Disordered Proteins, J. Chem. Inf. Model., 2015, 55, 1021–1029 Search PubMed.
- A. P. Ruymgaart, A. E. Cardenas and R. Elber, MOIL-opt: Energy-Conserving Molecular Dynamics on a GPU/CPU system, J. Chem. Theory Comput., 2011, 7, 3072–3082 Search PubMed.
- S. Brohee, K. Faust, G. Lima-Mendez, G. Vanderstocken and J. van Helden, Network Analysis Tools: from biological networks to clusters and pathways, Nat. Protoc., 2008, 3, 1616–1629 Search PubMed.
- A. del Sol, H. Fujihashi, D. Amoros and R. Nussinov, Residues crucial for maintaining short paths in network communication mediate signaling in proteins, Mol. Syst. Biol., 2006, 2, 0019 Search PubMed.
- J. Yang, H. Liu, X. Liu, C. Gu, R. Luo and H. F. Chen, Synergistic Allosteric Mechanism of Fructose-1,6-bisphosphate and Serine for Pyruvate Kinase M2 via Dynamics Fluctuation Network Analysis, J. Chem. Inf. Model., 2016, 56, 1184–1192 Search PubMed.
- W. Wang, C. Jiang, J. Zhang, W. Ye, R. Luo and H. F. Chen, Dynamics Correlation Network for Allosteric Switching of PreQ1 Riboswitch, Sci. Rep., 2016, 6, 31005 Search PubMed.
- A. Peselis and A. Serganov, Structure and function of pseudoknots involved in gene expression control, Wiley Interdiscip. Rev.: RNA, 2014, 5, 803–822 CrossRef CAS PubMed.
- M. Kang, C. D. Eichhorn and J. Feigon, Structural determinants for ligand capture by a class II preQ1 riboswitch, Proc. Natl. Acad. Sci. U. S. A., 2014, 111, E663–E671 Search PubMed.
- T. Santner, U. Rieder, C. Kreutz and R. Micura, Pseudoknot preorganization of the preQ1 class I riboswitch, J. Am. Chem. Soc., 2012, 134, 11928–11931 Search PubMed.
- A. Ren and D. J. Patel, c-di-AMP binds the ydaO riboswitch in two pseudo-symmetry-related pockets, Nat. Chem. Biol., 2014, 10, 780–786 Search PubMed.
- S. R. Wilkinson and M. D. Been, A pseudoknot in the 3′ non-core region of the glmS ribozyme enhances self-cleavage activity, RNA, 2005, 11, 1788–1794 Search PubMed.
Footnote |
† Electronic supplementary information (ESI) available. See DOI: 10.1039/c6mb00630b |
|
This journal is © The Royal Society of Chemistry 2017 |
Click here to see how this site uses Cookies. View our privacy policy here.