DOI:
10.1039/C6FO01215A
(Paper)
Food Funct., 2017,
8, 323-332
The kinetics and mechanism of α-glucosidase inhibition by F5-SP, a novel compound derived from sericin peptides
Received
15th August 2016
, Accepted 9th December 2016
First published on 12th December 2016
Abstract
The inhibition of α-glucosidase decreases postprandial blood glucose and therefore plays an important role in the treatment of type 2 diabetes mellitus. The present study investigated and characterized a peptide fraction of sericin hydrolysate, the kinetics of peptide-induced inhibition of α-glucosidase, and the interaction mechanism between the peptides and α-glucosidase. The fraction that eluted with 0.4 M NaCl (F5-SPs) on a DEAE-cellulose column exhibited significant inhibitory activity with an IC50 of 41 ± 1.94 μg mL−1. A kinetics analysis revealed that the F5-SP-induced inhibition was a reversible and parabolic mixed-type inhibition with a Ki value of 86.63 ± 0.014 μg mL−1. F5-SPs can bind to α-glucosidase at multiple sites to alter the conformation of α-glucosidase. F5-SPs were found to be rich in Gly, Ser, Glu, Tyr, Arg, and Pro, and had a sericin-conserved sequence SEDSSEVDIDLGNLG, as analyzed by Nano LC-MS/MS. Fluorescence spectra analysis showed that F5-SPs quenched the intrinsic fluorescence of α-glucosidase by a static quenching mechanism, and circular dichroism analysis suggested that the binding of F5-SPs to α-glucosidase resulted in the alteration of the secondary structure of an enzyme. The results of this study support the dietary recommendation of F5-SPs for the treatment of type 2 diabetes.
1. Introduction
In recent years, improvements in living standards and changes in the diet structure have resulted in a yearly increase in morbidity from diabetes mellitus (DM), which poses an increasingly serious public health problem.1 Type 2 DM is the predominant form of DM, and its main symptom is postprandial hyperglycemia.2,3 In medical practice, to control the development of DM and to reduce the occurrence of complications, an effective measure is to reduce the concentration of postprandial blood glucose (PBG). In intestinal villi, α-glucosidase, which is an enzyme that resolves carbohydrates into glucose, is a key molecular target for lowering blood glucose.4 By inhibiting the activity of α-glucosidase to delay and block the digestion of carbohydrates, the onset and progression of PBG is delayed.5 Furthermore, the product of α-glucosidase with an enzyme inhibitor was eliminated from the body as prototype or volatile gases, which fermented in the large intestine.6 Consequently, α-glucosidase inhibitors, such as acarbose and voglibose, are recommended for the reduction of PBG.
Recently, a newer, safer, and more effective α-glucosidase inhibitor has attracted attention. Silk proteins are native proteins derived from the production of silk. Recently, with the rapid development of medical biotechnology, silk proteins, especially sericin or sericin peptides, have been widely used and developed as new functional materials. Sericin peptides inhibit auto-oxidation and, when added to food, stabilize food quality and extend the shelf-life of foods. Foods such as teas and beverages, which contain sericin peptides, can inhibit germs, delay aging, maintain beauty, and enhance health.7–10 Moreover, sericin peptides can reduce serum lipids, prevent hypertension, reduce blood glucose, prevent blood clotting and thrombus, improve muscle energy, and prevent hyperchlorhydria.11–19 For example, Aramwit et al. reported that sericin can lower the concentration of serum lipids, elevate serum adiponectin, and improve glucose tolerance in mice fed with a high-fat diet.19 In addition, Lee et al. found that two polypeptides derived from sericin peptide E5K6 exhibited an anti-DM effect. Hence, sericin peptides are considered as potential inhibitors of α-glucosidase.20
To date, studies of the inhibition of α-glucosidase by sericin peptides have been limited to enzymatic activity assays. There are few reports on the mechanism of inhibition and the structural features of sericin peptides. Therefore, in this study, we isolated a fraction of sericin peptides with α-glucosidase inhibitory activity. We studied their structural properties and their interaction with α-glucosidase by conducting UV-vis absorption analysis, fluorescence analysis, circular dichroism (CD) analysis, and dynamics analysis, and we propose a potential inhibition mechanism.
2. Materials and methods
2.1 Materials
α-Glucosidase (EC 3.2.1.20, 19.3 units per mg) from Saccharomyces cerevisiae was obtained from Sigma-Aldrich Co. (St Louis, MO, USA) and dissolved in 0.05 M sodium phosphate buffer (pH 6.8). p-Nitrophenyl-α-D-glucopyranoside (pNPG, purity ≥ 99%) was purchased from Sigma-Aldrich Co. (St Louis, MO, USA). Sericin peptides with a molecular weight less than 3000 Da were obtained from the hydrolysis of sericin proteins by Neutrase (Novozymes Investment Co., Ltd, China), and purchased from Huzhou Xintiansi Bio-Tech Co., Ltd (Huzhou, China). All other chemicals were of analytical grade, and ultrapure water was used throughout the experiment.
2.2 Isolation of the sericin peptide fraction with α-glucosidase inhibitory activity
Sericin peptides (60 mg mL−1) were loaded onto an open column packed with DEAE cellulose DE-52 (3 × 20 cm) that was previously equilibrated with the starting buffer, 0.05 M Tris-HCl buffer (pH 7.4). The flow rate was 1 mL min−1. After elution of a sizeable quantity of unabsorbed material, the column was eluted with a stepwise gradient of 0.2 M NaCl and 0.4 M NaCl. The absorbance at 220 nm and the α-glucosidase inhibitory activity were monitored for all fractions. Each fraction was carefully transferred into Spectra/Por CE dialysis tubing with a molecular weight cutoff of 500 Da (Spectrum Laboratories, USA) and dialyzed against 2 L of ultrapure water three times at 25 °C. The collected dialysates in the tubing were lyophilized and stored at −20 °C for further investigation.
2.3 Assay for α-glucosidase inhibitory activity
The inhibitory activity of α-glucosidase was measured according to the method of Ohta et al.21 with slight modifications. The assay system was composed of 10 mM pNPG substrate and 0.175 U mL−1 enzyme solution in 50 mM sodium phosphate buffer (pH 6.8), with or without various concentrations of sericin peptides. The final volume of the reaction system was 0.21 mL. Briefly, 14 μL of different concentrations of sericin peptides were dissolved in 0.05 M sodium phosphate buffer (pH 6.8) and were mixed with 28 μL of 0.175 U mL−1 α-glucosidase in 96-well plates, and the mixture was pre-incubated at 37 °C for 30 min. Then, 28 μL of 10 mM pNPG was added to the inhibitor–enzyme mixture. The absorbance of the mixture was read at 405 nm every 30 s. The enzymatic activity measured without sericin peptides was defined as 100%. The α-glucosidase inhibitory activity of sericin peptides was expressed as the median effective concentration for inhibitory activity (IC50), that is, the amount of tested sericin peptides required for a 50% decrease in α-glucosidase activity.20 The relative enzymatic activity was calculated by the following eqn (1): |  | (1) |
2.4 Determination of the amino acid composition
The amino acid composition of the sample was measured by an automatic amino acid analyzer. The sample was hydrolyzed with 6 mol L−1 HCl at 110 °C for 24 h. The hydrolysate was dried, dissolved in 0.02 mol L−1 HCl, and centrifuged at 10
000 rpm for 25 min. The amino acid composition was then obtained by the automatic analysis algorithm of the amino acid automatic analyzer.
2.5 Nano liquid chromatography-tandem mass spectrometry (LC-MS/MS) for identification of active peptides
Nano LC-MS/MS was employed to identify the unknown eluted fraction possessing α-glucosidase inhibitory activity. The target fraction sample was desalted, concentrated, and re-dissolved in 2% acetonitrile with 0.1% formic acid. The re-dissolved sample was measured by an LC system (Nano Pump, Ultimate 3000, Dionex, Thermo fisher) with an Electrospray ionization-Quadrupole-Time of Flight (ESI-Q-TOF) mass spectrometer (maXis, Impact, Bruker Daltonik, Germany). A detailed protocol for the Nano LC-MS/MS analysis is provided in the method by Liu et al.22
2.6 Inhibition kinetics analysis
A mixed-type inhibition can be described by Lineweaver–Burk plots and is expressed in the following equation:23 | 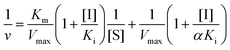 | (2) |
and secondary plots can be constructed as follows: | 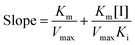 | (3) |
where Ki and Km are the inhibition constant and the Michaelis–Menten constant, respectively; v is the enzyme reaction velocity; α is the apparent coefficient; and [I] and [S] are the concentrations of the inhibitor and substrate, respectively.
Due to the parabolic relationship between the slope and [I], Ki cannot be calculated directly from the above equations of the Lineweaver–Burk plots. Thus, a modified equation for the parabolic type of inhibition was applied:24
| 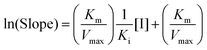 | (4) |
where the ln(Slope) is plotted
versus [I]. The plot was fitted linearly. The value of
Ki can be obtained from the modified equation.
2.7 Fluorescence spectra
Intrinsic fluorescence was detected at emission wavelengths in the range of 300–450 nm with an excitation wavelength at 280 nm at different temperatures (298 K, 304 K, and 310 K). The excitation and emission bandwidths were both set at 2.5 nm. The concentration of α-glucosidase was fixed at 0.35 U mL−1, and the sericin peptide concentration was varied from 0 to 90 μg mL−1.
To understand the hypothesized quenching mechanism between sericin peptides and α-glucosidase, the fluorescence quenching data were analyzed by the Stern–Volmer equation:25
| 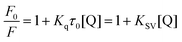 | (5) |
where
F0 and
F represent the fluorescence intensities in the absence and presence of the quencher, respectively,
Kq is the quenching rate constant of the biomolecule,
KSV is the dynamic quenching constant which was calculated by plotting the linear regression of
F0/
F against [Q],
τ0 is the average lifetime of the biomolecule without the quencher, and [Q] is the concentration of the quencher.
26
In the process of static quenching, there are two processes, bonding and dissociation, which are reversible between fluorescence quenching molecules. KD can be determined from the Lineweaver–Burk equation:
| (F0 − F)−1 = f−1F0−1 + KDF0−1f−1[Q]−1 | (6) |
where
f is the correction factor, and
KD is the dissociation constant.
2.8 CD spectra
CD measurements of α-glucosidase were performed in the absence and presence of sericin peptides at wavelengths of 200–250 nm. The CD spectra were obtained at a fixed concentration of α-glucosidase (1.4 U mL−1) and varying concentrations of sericin peptides (0–1.2 mg mL−1). All CD spectra were recorded in sodium phosphate buffer (pH 6.8) at 37 °C. The Secondary Structure Estimation program from Spectra Manager software (JASCO Corporation) was then used to analyze the contents of the different secondary structures of α-glucosidase, e.g., α-helix, β-sheet, β-turn, and random coil.
2.9 Statistical analyses
Experimental results, including the data from the α-glucosidase activity assay and inhibition kinetics analysis, were expressed as means ± standard deviation (n = 3), and data were analyzed using Origin 8.0. Statistical comparisons were made with the Student's t-test (P < 0.01).
3. Results and discussion
3.1 Isolation and characterization of sericin peptides with α-glucosidase inhibitory activity by ion exchange chromatography
Sericin peptides were separated into five fractions after being run on the DEAE cellulose DE-52 anion-exchange chromatography column, including three breakthrough peaks (F1, F2, F3), one F4 peak obtained by eluting with a stepwise gradient of 0.2 M NaCl, and one F5 peak obtained by eluting with a stepwise gradient of 0.4 M NaCl (Fig. 1). Only fraction F5 exhibited inhibitory activity toward α-glucosidase, and the yield of fraction F5 was 0.5% based on dry weight. Fraction F5 from sericin peptides was designated as F5-SP.
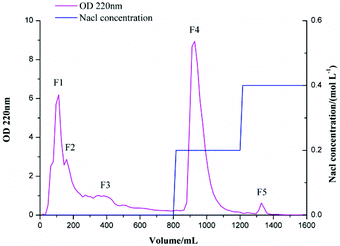 |
| Fig. 1 Ion-exchange chromatography of sericin peptides on a DEAE cellulose DE-52 column equilibrated with 0.05 M Tris-HCl buffer (pH 7.4). The peptides were eluted with a stepwise gradient of 0 M NaCl, 0.2 M NaCl, and 0.4 M NaCl, and at a flow rate of 1.0 mL min−1. The absorbance at 220 nm and the inhibitory activity toward α-glucosidase were monitored for all fractions. | |
F5-SPs exhibited dose-dependent inhibitory activity toward α-glucosidase (Fig. 2). The enzymatic activity decreased significantly when the concentration of F5-SPs was increased from 0 to 400 μg mL−1. The concentration of F5-SPs that gives rise to a 50% inhibition of activity (IC50) was found to be 41 ± 1.94 μg mL−1. F5-SPs had a similar α-glucosidase inhibitory activity as acarbose, which is reported to have an IC50 of 39.2 μg mL−1 for α-glucosidase and is widely recommended as an α-glucosidase inhibitor to treat PBG.27,28
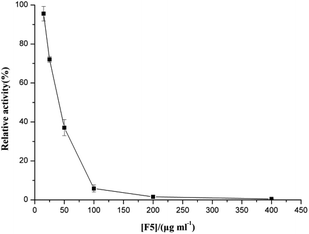 |
| Fig. 2 Inhibitory effect of F5-SPs on the activity of α-glucosidase. α-Glucosidase was incubated with or without F5-SPs at various concentrations for 30 min at 37 °C (pH 6.8) and then added to the assay system. The enzymatic activity measured without F5-SPs was defined as 100% relative activity. | |
We also investigated the reversibility of the inactivation effect of F5-SPs on α-glucosidase activity by evaluating the plots of the remaining activity versus different concentrations of α-glucosidase at various F5-SP concentrations (Fig. 3). The results showed that α-glucosidase was slightly active at low F5-SP concentrations (less than 20 μg mL−1) and was gradually inactivated in a dose-dependent manner with increasing F5-SP concentrations (Fig. 3). Moreover, in the plots of v versus [α-glucosidase], the straight lines all passed through the origin, which suggests that the inactivation of α-glucosidase induced by F5-SPs was reversible.29,30
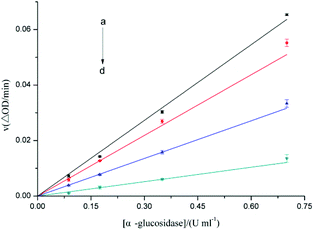 |
| Fig. 3 Plots of v versus [α-glucosidase] for evaluation of the type of inhibition that F5-SPs exert upon α-glucosidase. The v value indicates the change in absorbance at 405 nm per min at F5-SP concentrations of 0 μg mL−1 (a), 20 μg mL−1 (b), 40 μg mL−1 (c), and 80 μg mL−1 (d), under the conditions of 37 °C and pH 6.8. The final pNPG concentration was 0.67 mmol L−1. | |
3.2 Characteristics of the structure formation of F5-SPs
The amino acid composition of F5-SPs indicated that F5-SPs were rich in Gly (42.96%), Ser (5.79%), Glu (10.05%), Tyr (0.21%), Arg (1.28%), and Pro (0.99%) (Table 1). In previous studies, some peptides consisting of amino acids such as Gly, Ser, Glu, Tyr, Arg, and Pro have been considered as α-glucosidase inhibitors.20,28,31,32 For instance, Lee et al. found two tri-peptides, consisting of Gly-Tyr-Gly (GYG) and Gly-Glu-Tyr (GEY), whose IC50 values for the α-glucosidase inhibitory activity were 1.5 mg mL−1 and 2.7 mg mL−1.20
Table 1 Amino acid composition of F5-SPs
Amino acid |
Percentage (%) |
Asp |
31.09 |
Ser |
5.79 |
Glu |
10.05 |
Gly |
42.96 |
Val |
0.99 |
Ile |
0.38 |
Leu |
0.67 |
Thr |
1.99 |
Ala |
1.94 |
Cys |
0.03 |
Tyr |
0.21 |
Phe |
0.24 |
Lys |
1.02 |
His |
0.36 |
Arg |
1.28 |
Pro |
0.99 |
To identify the peptide components of F5-SPs and their sequences, the F5-SPs were eluted and analyzed by Nano RP LC-MS/MS. Fig. 4 is the total ion current chromatograph of F5-SPs, and Fig. 5 shows the chromatograms of three selected peptide fragments from F5-SPs that were identified. Using the MASCOT analysis tool, the peptide fragments from F5-SPs were compared to the sericin protein SERI1_BOMMO (NCBI number: P07856.2). The retrieving peptide summary report was obtained using the standard scoring tool with a significant threshold of P < 0.05 and an ion score or expected cut-off of 15.0. As shown in Fig. 6, the best fits for the three peptide fragments were peptide query numbers 6410, 6734, and 7174, which had the amino acid sequences SEDSSEVDIDLG, SEDSSEVDIDLGN, and SEDSSEVDIDLGNLG, respectively. Compared to SERI1_BOMMO (P07856.2), the hits of these three peptides had scores of 70, 48, and 65, respectively. Furthermore, when searching for homologous sequences for the protein SERI1_BOMMO using the BLASTP program in the NCBI protein database, the sequences of these peptide fragments showed similarity to the sequences from No. 490 to No. 504 in the protein SERI1_BOMMO (Fig. 7). Thus, the results of the Nano LC-MS/MS analysis in the peptide fingerprinting of F5-SPs revealed the presence of the conserved sequence SEDSSEVDIDLGNLG from sericin. This sequence may be a novel active peptide fragment of sericin with inhibitory activity toward α-glucosidase.
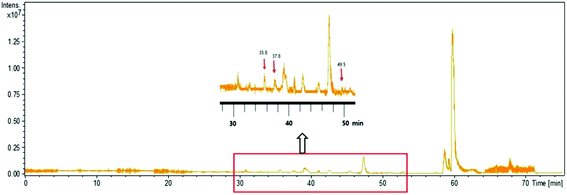 |
| Fig. 4 The total ion current chromatogram of F5-SPs. The enlarged drawing shows the ion corresponding to that of three identified sericin peptides, which were eluted at 35.8 min, 37.8 min, 49.5 min, respectively. | |
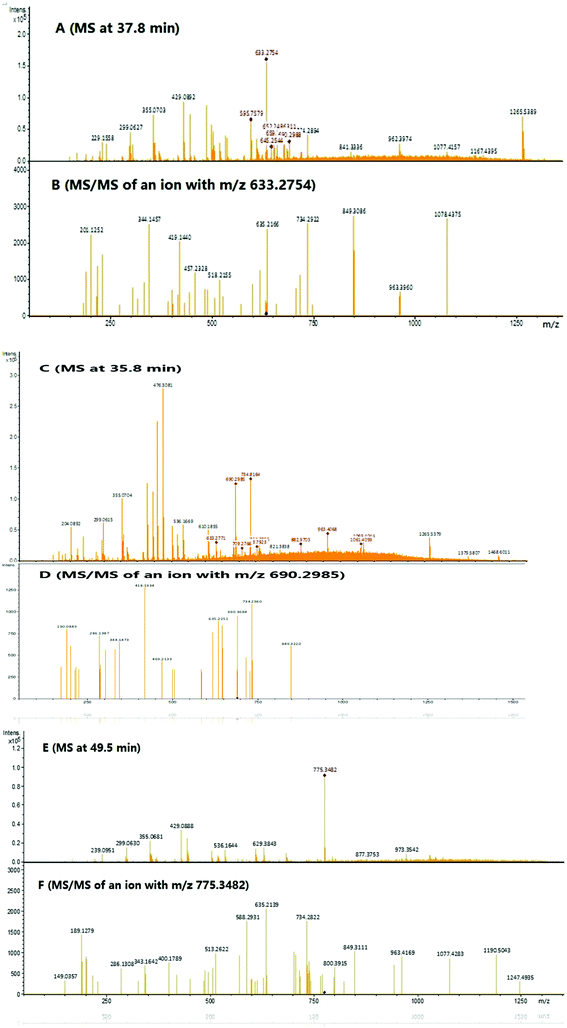 |
| Fig. 5 Large scale analysis of F5-SPs by Nano RP LC-MS/MS. (A) An MS survey scan during LC-MS/MS analysis at time 37.8 min. (B) MS/MS scan of the precursor ion m/z 633.2754. (C) An MS survey scan during LC-MS/MS analysis at time 35.8 min. (D) MS/MS scan of the precursor ion m/z 690.2985. (E) An MS survey scan during LC-MS/MS analysis at time 49.5 min. (F) MS/MS scan of the precursor ion m/z 775.3482. | |
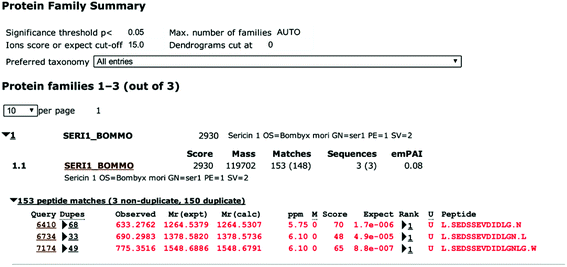 |
| Fig. 6 Identification of F5-SPs as sericin by Nano LC-MS/MS analysis and MASCOT software analysis. | |
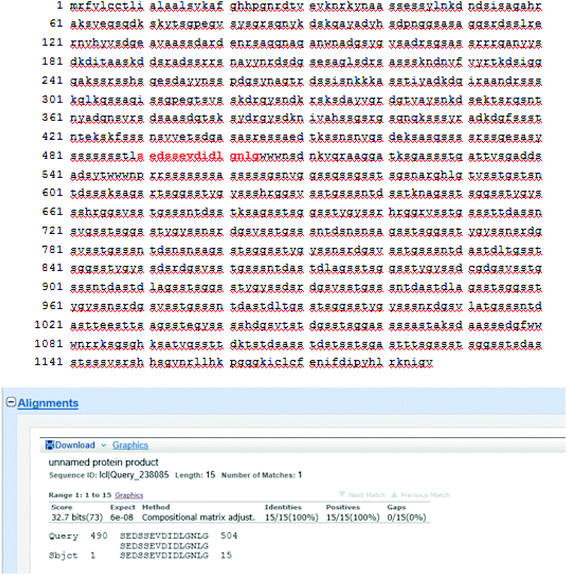 |
| Fig. 7 Homologous sequence alignment between identified peptide fragments of F5-SPs and the sericin protein SERI1_BOMMO (Prot: P07856.2). | |
3.3 Inhibition kinetics of F5-SPs on α-glucosidase activity
Lineweaver–Burk double reciprocal plots were used to evaluate the type of inhibition that F5-SPs exert upon the activity of α-glucosidase, and the initial velocity of the enzyme was monitored via absorbance changes at 405 nm. As the concentration of F5-SPs was increased from 0 to 40 μg mL−1, the slopes of the straight lines in the plots of 1/v versus 1/[pNPG] were also increased (Fig. 8A). The four straight lines did not cross one another on the 1/[pNPG] axis nor on the 1/v axis. With increasing F5-SP concentration, the value of vmax decreased, while the Km value first decreased, and then increased. Changes in the Km and Vmax values observed in the Lineweaver–Burk plot indicated that F5-SPs exerted a mixed-type inhibition on α-glucosidase, which is an intermediate of competitive and uncompetitive types of inhibition. This type of inhibition was strikingly similar to that of arabinose.33 As a mixed-type inhibitor, F5-SPs were able to bind either the free α-glucosidase or the α-glucosidase-substrate (pNPG) complex. However, the secondary replot of the slope versus [F5-SPs] yielded a parabolic curve (Fig. 8B), indicating that the F5-SPs did not act on a single inhibition site or a single class of inhibition sites of α-glucosidase. Additionally, the F5-SP-induced inhibition of α-glucosidase did not follow a linear mixed-type mechanism, but a parabolic mixed-type mechanism, which agrees with the characterization of mixed-type inhibitors, as previously reported by Zeng YF et al.24
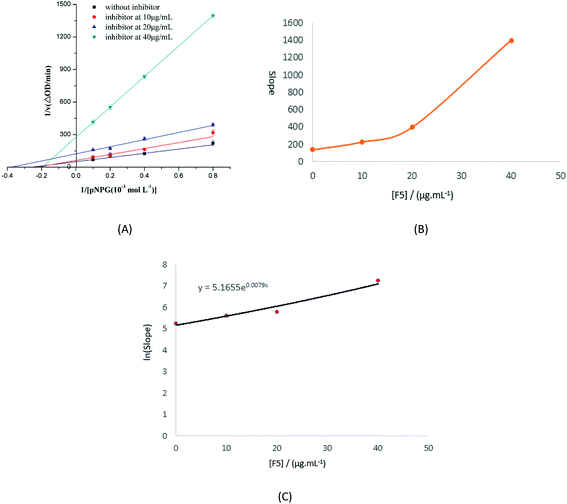 |
| Fig. 8 Determination of the type of inhibition and the inhibition constants. (A) Lineweaver–Burk plot. The F5-SP concentrations were 0 μg mL−1 (■), 10 μg mL−1 (●), 20 μg mL−1 (▲), and 40 μg mL−1 (▼). The final enzyme concentration was 0.175 U mL−1. The pH and temperature of the reaction were 6.8 and 37 °C, respectively. (B) The secondary plot of slope versus [F5]. The data were obtained from (A), and eqn (2) was used to obtain the plot. (C) The secondary replot of the parabolic type of inhibition. Eqn (3) was applied for plotting. | |
Using eqn (4), a secondary replot of the parabolic type of inhibition was obtained (Fig. 8C), and the Ki value was calculated to be 86.63 ± 0.014 μg mL−1 which is approximately equal to 1.2 × 10−4 mol L−1 (according to the molecular weight of the three best fit peptides above). Yan et al. reported that the Ki value of luteolin on α-glucosidase was (1.40 ± 0.02) × 10−4 mol L−1,26 which is close to the value that we obtained in our assay. Based on these findings, we infer that F5-SPs can bind to α-glucosidase at multiple sites to induce conformational changes in α-glucosidase, which results in altered Km and Vmax values.
3.4 Conformational changes of α-glucosidase induced by F5-SPs
Fluorescence spectra.
Conformational information, including the binding mechanism, binding constants, and binding sites, can be inferred from fluorescence spectra. Therefore, we investigated the structural changes in α-glucosidase induced by F5-SPs by measuring the intrinsic fluorescence of α-glucosidase. The fluorescence quenching spectra of α-glucosidase in response to F5-SPs are displayed in Fig. 9A. After being excited at a wavelength of 280 nm, α-glucosidase displayed a strong fluorescence emission peak at 340 nm. A significant and gradual decrease in the intrinsic fluorescence of α-glucosidase was observed when F5-SPs were added at increasing concentrations at 298 K (Fig. 9A), indicating that F5-SPs interacted with α-glucosidase to quench the intrinsic fluorescence of α-glucosidase.
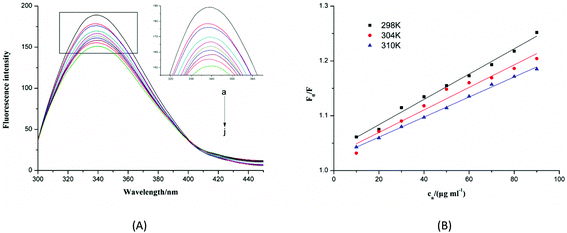 |
| Fig. 9 Changes in intrinsic α-glucosidase fluorescence at different F5-SP concentrations and temperatures. (A) Fluorescence spectra of α-glucosidase in the presence of F5-SPs at different concentrations (pH 6.8, T = 298 K, ex = 280 nm). The concentration of α-glucosidase was 0.35 U mL−1, and the concentration of F5-SPs was 0 μg mL−1 (a), 10 μg mL−1 (b), 20 μg mL−1 (c), 30 μg mL−1 (d), 40 μg mL−1 (e), 50 μg mL−1 (f), 60 μg mL−1 (j), 70 μg mL−1 (h), 80 μg mL−1 (i) and 90 μg mL−1 (j); (B) the Stern–Volmer plots for the fluorescence quenching of α-glucosidase by F5-SPs at different temperatures (pH 6.8, T = 298 K, ex = 280 nm). Cn was the concentration of F5-SPs. | |
Fluorescence quenching can occur by different mechanisms, which are usually classified as either static quenching or dynamic quenching. For dynamic quenching, the mechanism can be described by the Stern–Volmer equation (eqn (5)). Fig. 9B shows the Stern–Volmer plots for the interaction of α-glucosidase with F5-SPs at three different temperatures (298, 304, and 310 K). The values of KSV were calculated from eqn (5) and are listed in Table 2. The data clearly show that the KSV values decreased with increasing temperature, and the corresponding Kq value was approximately 1011 L mol−1 s−1 which was calculated according to eqn (5). The maximum scatter collision-quenching constant (Kq) for various quenching reactions with the biopolymer was 2 × 1010 L mol−1 s−1, so the constants for F5-SP induced α-glucosidase quenching were greater than the maximum scatter collision-quenching constant.34 These results suggested that, due to the formation of F5-SP–α-glucosidase complexes, the fluorescence quenching process was dominated not by dynamic collision but by a static quenching mechanism, which is in agreement with the report of Shahabadi et al.35
Table 2 Quenching constants (KSV) of the F5-SP–α-glucosidase interaction at different temperatures
T (K) |
Stern–Volmer equation |
R
2
|
K
sv (mL μg−1) |
298 |
F
0/F = 1.038 + 0.00231cn |
0.9903 |
0.00231 |
304 |
F
0/F = 1.028 + 0.00206cn |
0.9627 |
0.00206 |
310 |
F
0/F = 1.024 + 0.00183cn |
0.9975 |
0.00183 |
In the static quenching interaction, the processes of bonding and dissociation are reversible between fluorescence quenching molecules, and KD can be determined from the Lineweaver–Burk equation. Fig. 10 shows the Lineweaver–Burk plots for the interaction between F5-SPs and α-glucosidase at different temperatures (298 K, 304 K, and 310 K). The correlation coefficients (R2) for the Lineweaver–Burk equations were larger than 0.96 (Table 3), indicating that the variability of the data, which determines the deviation of eqn (6) was very small and the mathematical model was satisfactory. The values of the dissociation constant (KD) for the F5-SP–α-glucosidase complex were calculated from eqn (6) and are shown in Table 3. These results revealed that the values of KD increased with increasing temperature, which suggested that the binding capacity of F5-SPs to α-glucosidase declined with increasing temperature, and that the binding process was an endothermic reaction.34
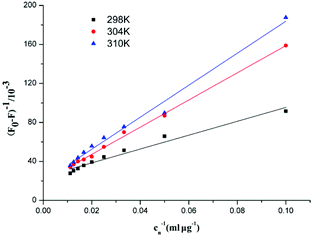 |
| Fig. 10 Lineweaver–Burk plots for the interaction between F5-SPs and α-glucosidase. Cn was the concentration of F5-SPs. | |
Table 3 Lineweaver–Burk equation for the interaction between F5-SPs and α-glucosidase
T (K) |
Lineweaver–Burk equation |
R
2
|
K
D (mL μg−1) |
298 |
(F0 − F)−1 = 0.0243 + 0.7109cn−1 |
0.9664 |
29.31 |
304 |
(F0 − F)−1 = 0.0194 + 1.3941cn−1 |
0.9973 |
72.03 |
310 |
(F0 − F)−1 = 0.0197 + 1.6413cn−1 |
0.9876 |
83.44 |
Circular dichroism.
Circular dichroism (CD) is a sensitive and precise technique for determining the conformational changes of a protein upon interaction with other molecules. In this study, we used CD to investigate the change in the secondary structure of α-glucosidase induced by the presence of F5-SPs (Fig. 11). In the CD spectrum of α-glucosidase, two negative humped peaks at 209 and 222 nm indicated that α-glucosidase has a high percentage of α-helical structures.36 In the presence of F5-SPs, the negative humped peaks at 209 nm and 222 nm were diminished compared with the control α-glucosidase (Fig. 11). This indicated that when F5-SPs were bound to α-glucosidase, the helicity of α-glucosidase was decreased. The Secondary Structure Estimation Program was used to calculate the contents of different secondary structures of α-glucosidase. With increasing F5-SP concentration, the percentages of α-helices and β-sheets in the overall secondary structure of α-glucosidase were decreased, while those of β-turns and random coils were increased (Table 4). Other α-glucosidase inhibitors, such as luteolin26 and hydroxycoumarin derivatives,37 were also found to reduce the percentage of α-helices in the overall secondary structure of α-glucosidase. These data show that the inhibitors induced changes in the secondary structure of α-glucosidase and affected the folding of the protein.37,38 Therefore, it can be concluded that the binding of F5-SPs to α-glucosidase induced changes in the secondary structure of α-glucosidase. This may contribute to the inhibition mechanism whereby F5-SPs prevent substrate binding and inactivate α-glucosidase.39
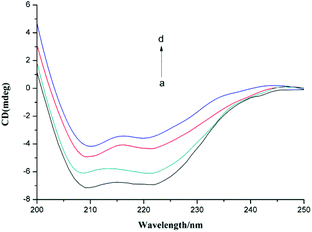 |
| Fig. 11 CD spectra of α-glucosidase in the presence of various concentrations of F5-SPs (T = 310 K, pH = 6.8). The concentration of α-glucosidase was 2.0 × 10−6 mol L−1, and the concentrations of F5-SPs were 0 mg mL−1 (a), 0.3 mg mL−1 (b), 0.6 mg mL−1 (c), and 1.2 mg mL−1 (d). | |
Table 4 The contents (CD spectra) of different secondary structures of free α-glucosidase and F5-SP–α-glucosidase complexes at pH 6.8
Concentration of F5-SPs (mg mL−1) |
α-Helix (%) |
β-Sheet (%) |
β-Turn (%) |
Random coil (%) |
0 |
33 |
28.2 |
12.9 |
25.9 |
0.3 |
26.9 |
27.7 |
17.3 |
28.1 |
0.6 |
23.1 |
24.4 |
23.7 |
28.8 |
1.2 |
20.6 |
22 |
27.6 |
29.8 |
4. Conclusion
In this study, we isolated a novel component derived from sericin peptides, F5-SP, a bioactive compound that possesses inhibitory activity toward α-glucosidase and that is rich in Gly, Ser, Glu, Tyr, Arg, and Pro. We investigated the kinetics of the F5-SP-induced inhibition of α-glucosidase and the interaction mechanisms of F5-SPs with α-glucosidase. Results showed that F5-SP significantly inhibited α-glucosidase activity with an IC50 of 41 ± 1.94 μg mL−1, and its inhibition type was a reversible and parabolic mixed-type inhibition with a Ki of 86.63 ± 0.014 μg mL−1. F5-SPs bound to α-glucosidase at multiple sites and altered the conformation of α-glucosidase. The conformational changes of α-glucosidase induced by interaction with F5-SPs were evaluated by fluorescence measurements and circular dichroism, and the results indicated that F5-SPs quenched the intrinsic fluorescence of α-glucosidase by a static quenching mechanism. With increasing concentration of F5-SPs, the overall secondary structure of α-glucosidase exhibited a decrease in the α-helical and β-sheet content and an increase in the β-turn and random coil content. F5-SPs contained the sericin-conserved sequence SEDSSEVDIDLGNLG, which indicates that it is a novel, sericin-derived active ingredient with inhibitory activity toward α-glucosidase, and it can potentially be exploited as a novel α-glucosidase inhibitor for the treatment of type 2 diabetes.
Acknowledgements
This work was supported by the National High Technology Research and Development Program of China (No. 2013AA102207), the Natural Science Foundation of China (No. 31471623, 21276154 and 31571779), and the Special National Key Research and Development Plan (No. 2016YFD0400206). The authors are grateful to the Instrumental Analysis Center of Shanghai Jiao Tong University for the equipment support.
References
- P. Zimmet, K. G. Alberti and J. Shaw, Nature, 2001, 414, 782–787 CrossRef CAS PubMed.
- M. A. H. Hazari, B. Ram Reddy, N. Uzma and B. Santhosh Kumar, Int. J. Diabetes Mellit., 2015, 3, 19–24 CrossRef.
- I. Heydari, V. Radi, S. Razmjou and A. Amiri, Int. J. Diabetes Mellit., 2010, 2, 61–63 CrossRef.
- H. Gao, Y.-N. Huang, B. Gao, P.-Y. Xu, C. Inagaki and J. Kawabata, Food Chem., 2008, 106, 1195–1201 CrossRef CAS.
- S. Xiao, J. Japanese Med., 1999, 20, 22–24 Search PubMed.
- A. D. Baron, Diabetes Res. Clin. Pract., 1998, 40, S51–S55 CrossRef CAS PubMed.
- R. Dash, M. Mandal, S. K. Ghosh and S. C. Kundu, Mol. Cell. Biochem., 2008, 311, 111–119 CrossRef CAS PubMed.
- S. C. Kundu, B. C. Dash, R. Dash and D. L. Kaplan, Prog. Polym. Sci., 2008, 33, 998–1012 CrossRef CAS.
- M. Sasaki, N. Kato, H. Watanabe and H. Yamada, Oncol. Rep., 2000, 7, 1049–1052 CAS.
- M. Sasaki, H. Yamada and N. Kato, Nutr. Res., 2000, 20, 1505–1511 CrossRef CAS.
- T. M. Singh, M. H. Kadowaki, S. Glagov and C. K. Zarins, Am. J. Surg., 1990, 160, 156–159 CrossRef CAS PubMed.
- K. Gotoh, H. Izumi, T. Kanamoto, Y. Tamada and H. Nakashima, Biosci., Biotechnol., Biochem., 2000, 64, 1664–1670 CrossRef CAS PubMed.
- K. Tsubouchi, Y. Igarashi, Y. Takasu and H. Yamada, Biosci., Biotechnol., Biochem., 2005, 69, 403–405 CrossRef CAS PubMed.
- T. Toyosawa, S. Terada, M. Sasaki, H. Yamada and M. Kino-Oka, Animal Cell Technology: Basic and Applied Aspects, 2006, 14, 155–161 Search PubMed.
- S. Terada, N. Takada, K. Itoh, T. Saitoh, M. Sasaki and H. Yamada, Cell. Technol. Cell. Pro., 2007, 397–401 Search PubMed.
- E. Y. Jung, H. S. Lee, H. J. Lee, J. M. Kim, K. W. Lee and H. J. Suh, Nutr. Res., 2010, 30, 783–790 CrossRef CAS PubMed.
- S. Shin, S. Yeon, D. Park, J. Oh, H. Kang, S. Kim, S. S. Joo, W. T. Lim, J. Y. Lee and K. C. Choi, Biol. Pharm. Bull., 2010, 33, 273–278 CAS.
- H. S. Lee, H. J. Lee and H. J. Suh, Nutr. Res., 2011, 31, 937–943 CrossRef CAS PubMed.
- P. Aramwit, T. Siritientong and T. Srichana, Waste Manage. Res., 2012, 30, 217–224 CrossRef CAS PubMed.
- H. J. Lee, H. S. Lee, J. W. Choi, K. S. Ra, J. M. Kim and H. J. Suh, J. Agric. Food Chem., 2011, 59, 11522–11525 CrossRef CAS PubMed.
- T. Ohta, S. Sasaki, T. Oohori, S. Yoshikawa and H. Kurihara, Biosci. Biotechnol. Biochem., 2002, 66, 1552–1554 CrossRef CAS PubMed.
- H. Liu, W. B. Sun, R.-B. Liang, L. Huang, J. L. Hou and J. H. Liu, J. Proteomics, 2015, 123, 14–28 CrossRef CAS PubMed.
- B. T. Burlingham and T. S. Widlanski, J. Chem. Educ., 2003, 80, 214–218 CrossRef CAS.
- Y. F. Zeng, J. Lee, Y. X. Si, L. Yan, T. R. Kim, G. Y. Qian, Z. R. Lü, Z. M. Ye and S. J. Yin, Process Biochem., 2012, 47, 2510–2517 CrossRef CAS.
- Y. Q. Li, F. C. Zhou, F. Gao, J. S. Bian and F. Shan, J. Agric. Food Chem., 2009, 57, 11463–11468 CrossRef CAS PubMed.
- J. Yan, G. Zhang, J. Pan and Y. Wang, Int. J. Biol. Macromol., 2014, 64, 213–223 CrossRef CAS PubMed.
- Y. M. Kim, M. H. Wang and H. I. Rhee, Carbohydr. Res., 2004, 339, 715–717 CrossRef CAS PubMed.
- Z. Yu, Y. Yin, W. Zhao, Y. Yu, B. Liu, J. Liu and F. Chen, Food Chem., 2011, 129, 1376–1382 CrossRef CAS.
- Y. F. Zeng, Z. R. Lü, L. Yan, S. Oh, J. M. Yang, J. Lee and Z. M. Ye, Process Biochem., 2012, 47, 2284–2290 CrossRef CAS.
- Y. Wang, M. J. Curtis-Long, B. W. Lee, H. J. Yuk, D. W. Kim, X. F. Tan and K. H. Park, Bioorg. Med. Chem., 2014, 22, 1115–1120 CrossRef CAS PubMed.
- T. Matsui, T. Oki and Y. Osajima, Z. Naturforsch., C, J. Biosci., 1999, 54, 259–263 CAS.
- J. G. Luo, X. B. Wang, L. Ma and L. Y. Kong, ChemInform, 2007, 38, 4460–4463 Search PubMed.
- W. J. Hu, L. Yan, D. Park, H. O. Jeong, H. Y. Chung, J. M. Yang, Z. M. Ye and G. Y. Qian, Int. J. Biol. Macromol., 2012, 50, 694–700 CrossRef CAS PubMed.
- P. Kandagal, S. Ashoka, J. Seetharamappa, V. Vani and S. Shaikh, J. Photochem. Photobiol. A: Chem., 2006, 179, 161–166 CrossRef CAS.
- N. Shahabadi, M. Maghsudi, Z. Kiani and M. Pourfoulad, Food Chem., 2011, 124, 1063–1068 CrossRef CAS.
- Z. Y. Du, R. R. Liu, W. Y. Shao, X. P. Mao, L. Ma, L. Q. Gu, Z. S. Huang and A. S. Chan, Eur. J. Med. Chem., 2006, 41, 213–218 CrossRef CAS PubMed.
- Q. Shen, J. Shao, Q. Peng, W. Zhang, L. Ma, A. S. Chan and L. Gu, J. Med. Chem., 2010, 53, 8252–8259 CrossRef CAS PubMed.
- J. S. Mandeville, E. Froehlich and H. Tajmir-Riahi, J. Pharm. Biomed. Anal., 2009, 49, 468–474 CrossRef CAS PubMed.
- X. J. Hu, X. B. Wang and L. Y. Kong, J. Agric. Food Chem., 2013, 61, 1501–1508 CrossRef CAS PubMed.
Footnote |
† These two authors contributed equally to this paper. |
|
This journal is © The Royal Society of Chemistry 2017 |
Click here to see how this site uses Cookies. View our privacy policy here.