DOI:
10.1039/C6FO01139J
(Paper)
Food Funct., 2017,
8, 142-150
A novel antioxidant peptide derived from wheat germ prevents high glucose-induced oxidative stress in vascular smooth muscle cells in vitro
Received
31st July 2016
, Accepted 16th November 2016
First published on 17th November 2016
Abstract
The ingestion of whole wheat products is responsible for the prevention of a number of chronic diseases. The aim of this study is to characterize the molecular properties of a novel wheat germ-derived antioxidant peptide (AOP) in protection against high glucose-induced oxidative stress in vascular smooth muscle cells (VSMCs). AOP (amino acid sequence AREGETVVPG) was isolated from wheat germ albumin using dual-enzymatic hydrolysis/separation, followed by MS/MS. The in vitro IC50 value for Fe2+ chelation was 0.77 mM. The stability of purified AOP was confirmed by hydrolyzation in a simulated gastrointestinal (GI) digestion system in vitro. Furthermore, AOP significantly prevented high glucose-induced cell growth by 37.4 ± 2.7%, decreased the generation of intracellular reactive oxygen species (ROS) by 22.3 ± 4.6%, suppressed the phosphorylation of PKCζ, AKT and Erk1/2, and inhibited Nox4 protein expression. Our findings suggested that AOP exerted a protective role against high glucose-induced oxidative stress through the inhibition of the PKCζ/Nox4 signaling pathway in VSMCs.
1. Introduction
Epidemiological studies have shown that the increased consumption of whole grains provides a wide range of health benefits in the prevention of chronic diseases, such as diabetes, obesity, and cancer.1–5 In contrast to refined grains, whole grains retain the germ, endosperm, and bran and are considered superior to the refined products. Regarding the bioactive compounds of whole grains, previous publications have largely focused on dietary fibers, vitamins, and other phytochemicals including but not limited to carotenoids, phenolics, and lignans.2,6–9 However, the protective capacity of proteins and/or peptides derived from whole grains has not been well determined.
It is proposed that wheat proteins are the leading source of vegetable proteins exhibiting significant functions in health and diseases. However, the potential role of wheat proteins and/or derived peptides in antioxidative stress has not been well defined. Although the antioxidant activity of the wheat germ-derived peptides has been presented in a few recent studies,10,11 the mechanism by which those antioxidant peptides act in the protection of cells against oxidative stress and consequent cell survival is unknown.
Oxidative stress is caused by an imbalance between reactive oxygen species (ROS) and the antioxidant defense system and is associated with several diseases.12–14 Excessive levels of ROS may lead to increased oxidation of biological compounds such as lipids, proteins and DNA, leading to oxidative damage to the cells.15 One of the mechanisms of antioxidant activity in redox reaction is based on the ability of the substance to quench free radical chain reactions by donating hydrogen atoms to free radicals. Antioxidant peptides derived from protein hydrolysates have been proven to have activity in scavenging free radicals, in inhibiting lipid peroxidation and in metal ion chelation. In particular, the antioxidant peptides obtained from dietary proteins are provided as a supplement to protect the human body against ROS damage.16–18 In the mammalian gastrointestinal (GI) tract, peptides are released from the dietary proteins during digestion.19 The capacity of the peptide antioxidant activity can be attributed to their structure and amino acid sequences.20
Wheat germ protein accounts for 31% of the whole kernel and mainly consists of albumin, globulin, gliadin, and gluten. Among these proteins, albumin accounts for approximately 30–35% of total wheat germ protein and is recognized as a high-quality protein with a large diversity of amino acid residues. Wheat germ proteins are an excellent protein source that can be hydrolyzed into bioactive peptides, such as antioxidant peptides.10,11,21 Our preliminary studies showed that hydrolysates of wheat germ albumin have higher antioxidant activities compared to other protein hydrolysates with the same enzymes, suggesting that the amino acid sequences of wheat germ albumin protein have higher biological activities, and wheat germ albumin could be considered as a good source of antioxidant peptides, though only a few such peptides have been identified.
Exposure of cultured vascular smooth muscle cells (VSMCs) to high glucose is known to result in an increase in ROS leading to an increase in the state of cellular oxidative stress. ROS generation is significantly increased in VSMCs under hyperglycemia and Nox4 is a critical catalytic component for ROS production in quiescent VSMCs in high glucose.22,23 In this study, a novel antioxidant peptide (AOP, the amino acid residue sequence: AREGETVVPG) was isolated from wheat germ albumin hydrolysates using a two-step, dual-enzymatic hydrolysis and identified using QTOF-ESI-MS/MS. The antioxidant capacity of this peptide was determined in vitro and in high glucose-challenged vascular smooth muscle cells (VSMCs). The results suggest that there are potential applications for this antioxidant peptide in preventing diabetic atherosclerosis.
2. Materials and methods
2.1. Materials
Fresh wheat germ was donated by the Huaian Xinfeng flour mill (Jiangsu, China). Alcalase was purchased from Novo Co. (Novo Nordisk, Bagsvaerd, Denmark). Acetonitrile and formic acid were purchased from BD Biosciences (San Diego, CA, USA). The following chemicals were ordered from Sigma Chemical Co. (St Louis, MO, USA): ferrozine, pepsin, trypsin, bile extract, 3-(4,5-dimethyl-2-thiazolyl)-2,5-diphenyl-2-H-tetrazolium bromide (MTT), 2′,7′-dichlorofluorescein diacetate (DCF-DA), and 45% D-(+)-glucose solution. Dulbecco's modified Eagle's medium (DMEM) containing 5 mM glucose, fetal bovine serum (FBS), penicillin–streptomycin, and Hank's Balanced Salt Solution (HBSS) were purchased from Life Technologies (Grand Island, NY, USA). Antibodies against NADPH oxidase 4 (Nox4) and β-actin were purchased from Abcam (Cambridge, MA, USA). Anti-phospho-protein kinase ζ (PKCζ) on Thr410, phospho-AKT on Ser473, phospho-Erk1/2 on Thr202/Tyr204, Erk1/2 (extracellular signal-related kinase 1/2), and AKT were purchased from Cell Signaling Technology Inc. (Beverly, MA, USA). All other chemicals used in the experiments were analytical grade.
2.2. Preparation of antioxidant peptides
Antioxidant peptides (AOP) from the wheat germ albumin were obtained by using two-step dual-enzymatic hydrolysis. First, wheat germ albumin of 1% (w/v) was hydrolyzed by trypsin (enzyme
:
substrate ratio at 7000 U
:
1 g) in 0.1 M phosphate buffer (pH 7.6) at 37 °C for 6 h. The tryptic hydrolysates were fractionated by ultrafiltration with a membrane with a molecular weight cut-off of 10 kDa (Amicon, Cambridge, MA, USA). The antioxidant activities of these fractions were determined by the ferrous ion-chelating activity method. The crude <10 kDa fractions which exhibited high antioxidant capacity were further separated by gel filtration chromatography (Sephadex G-75, column size, 25 mm × 750 mm) into three fractions (Pa, Pb, and Pc). The fraction Pb with a higher antioxidant activity was further hydrolyzed by alcalase (enzyme
:
substrate ratio at 0.15 AU
:
1 g) in 0.1 M phosphate buffer (pH 7.6) at 50 °C for 90 min. The hydrolysates were then separated by Sephadex G-15 gel filtration chromatography (column size, 15 mm × 600 mm) into two fractions (Pd and Pe). The fraction Pd with a higher antioxidant activity was further subjected to purification by RP-HPLC (Waters XBridge prep C18, 300 Å, 5 μm, 10 mm × 150 mm) and the five fractions (P1, P2, P3, P4, and P5) were separated. Each fraction was dried by vacuum concentration/evaporation. The highest antioxidant fraction P3 was achieved and the structure was determined by ESI-TOF MS/MS.
2.3. Determination of the molecular mass and the amino acid sequence of AOP
The accurate molecular mass and amino acid sequence of the purified AOP fraction were determined using a Q-TOF (time of flight) mass spectrometer coupled with an electrospray ionization (ESI) source (Xevo G2-XS Q-TOF, Waters, USA). The freeze-dried AOP sample was dissolved in distilled, deionized water (ddwater) at a concentration of 20 μg ml−1. The solution was continuously injected. The settings of the Q-TOF mass spectrometer were as follows: capillary voltage (kV), 3.0 (+), 2.5 (−); source temperature, 120 °C; desolvation temperature, 500 °C; desolvation gas flow, 1000 (L h−1). Positive ion intensities were recorded over a mass range (m/z) of 100–1500, and the molecular mass was determined by a doubly charged [M + 2H]+2 state in the mass spectrum. Following the determination of the molecular mass, the peptide was automatically selected for fragmentation, and the sequence information was obtained by tandem mass spectrometry (MS/MS) analysis.
2.4. Peptide synthesis
The peptide AREGETVVPG was chemically synthesized using the solid-phase method and purified by HPLC by Sangon Biotech Co., Ltd (Shanghai, China). The purity (≥98%) and the sequence were confirmed by mass spectrometry.
2.5. Ferrous ion-chelating activity
The ferrous ion (Fe2+)-chelating activity was determined according to the method established by Hatami et al.24 Samples at different concentrations, as indicated, were added to a solution of 2 mM FeCl2 (50 μl). The reaction was initiated by addition of 50 μl 30% (w/v) ferrozine solution. After incubation at room temperature for 5 min, the absorbance of the mixture was read at 562 nm. The assay was performed in triplicate. The results were calculated according to the following formula:
Fe2+-chelating activity (%) = [(A0 − A1)/A0]× 100% |
where A0 is the absorbance of the control, and A1 is the absorbance in the presence of samples. The IC50 value is defined as the concentration of the AOP that is able to chelate 50% of ferrous ions. Different concentrations of the AOP were selected and evaluated for the ferrous ion-chelating activity. The IC50 of AOP was determined by plotting the ferrous ion-chelating activity against various concentrations of AOP.
2.6. Simulated gastrointestinal digestion
A two-stage hydrolysis process was carried out as previously reported but with slight modifications.25 The synthetic peptide (AOP, 10 mg ml−1) was initially hydrolyzed with pepsin [the ratio of enzyme
:
substrate is 1
:
25 (w/w)] at pH 2.0 at 37 °C for 2 h, followed by hydrolysis with a mixture of trypsin and bile extract (enzyme
:
substrate ratio of 1
:
25, w/w, and 1
:
16, w/w, respectively) at pH 7.5 and 37 °C for 4 h. The mixture was then heated at 95 °C for 10 min to inhibit the enzymes and then cooled down in an ice-bath. RP-HPLC (Agilent 1260 HPLC System, Agilent Technologies, Waldbronn, Germany) was employed to analyze the stability of the AOP during the simulated GI digestion.
2.7. Cell culture
The VSMCs (kindly provided by Dr Junyu Ning at Beijing Municipal Centers for Disease Prevention and Control, China) were prepared from porcine aortas and maintained in culture as described previously.26 The VSMCs were cultured in DMEM normal glucose (1 g L−1, 5 mM) medium supplemented with 10% fetal bovine serum (FBS), 100 μg ml−1 streptomycin, and 100 units per ml penicillin. All cells were cultured under a humidified atmosphere in a 5% CO2 incubator at 37 °C. Cultured VSMCs were used between passages 5 and 14.
2.8. MTT assay
The effect of AOP on cell growth in high glucose was determined using the MTT (3-(4,5-dimethyl-2-thiazolyl)-2,5-diphenyl-2-H-tetrazolium bromide) assay. The VSMCs were plated at a density of 5000 cells per well in a 96-well plate in DMEM normal glucose medium with 2% FBS. Cells were allowed to attach for 48 h before the medium was replaced with 100 μl DMEM containing normal glucose or high glucose (25 mM) and with or without AOP at different concentrations, as indicated. After 24 h incubation, MTT was added into each well at a final concentration of 0.5 mg ml−1. Then, the mixture was incubated continuously in the dark at 37 °C for 4 h. The medium was removed and the cells were lysed with isopropanol containing 0.04 N HCl. Absorbance was measured at a wavelength of 570 nm with a background subtraction of 630 nm.
2.9. ROS measurement
The intracellular generation of ROS in VSMCs was measured using the DCF-DA (nonpolar, 2′,7′-dichlorofluorescein diacetate) assay according to the method of Xi et al.23 Cells (at 5 × 104 cells per well) were seeded in a 24-well glass-bottom plate and incubated for 48 h; then, the cells were serum-starved for 16 h before different treatments. The experimental groups were treated with high glucose in the absence or presence of AOP at different concentrations. The experimental groups treated with normal glucose only (5 mM) or with the addition of H2O2 (200 μM) were used as negative and positive controls, respectively. The cells were washed twice with HBSS containing 2.5 mM CaCl2 and 1.0 mM MgSO4 (washing buffer). DCF-DA (10 μM in washing buffer) was loaded into the cells, and they were incubated for an additional 1 h before measuring the OD in a CytoFluor fluorescence plate reader (excitation wavelength, 485 nm/emission wavelength, 530 nm).
2.10. Immunoblotting
The VSMCs were seeded at 1 × 105 cells in a 6-well plate and grown for 5–6 days to reach confluence. The cultures were incubated in serum-free normal glucose for 16 h before being exposed to a high level of glucose with or without 5 μM AOP for 6 h. Normal glucose (5 mM)-treated cells were used as a negative control. The cells were exposed to IGF-I (100 ng ml−1) for 10 min prior to harvesting for detecting phosphorylation of AKT (Ser473). All plates were washed twice with ice-cold PBS and lysed using radio-immunoprecipitation assay (RIPA) buffer. Following cell lysis, immunoblotting was carried out as described previously27,28 using a dilution of 1
:
500 for anti-Nox4 and 1
:
1000 for anti-PKCζ, phospho-PKCζ (Thr410), phospho-AKT (Ser473), phospho-Erk1/2 (Thr202/Tyr204), Erk1/2 and β-actin antibodies. The immunoblotting bands were visualized using enhanced chemiluminescence (Thermo Fisher Scientific, Rockford, IL). Densitometric analyses of the images were carried out using National Institutes of Health ImageJ, version 1.37v. Total cellular protein in the lysates was determined using the BCA method (Thermo Fisher Scientific, Rockford, IL).
2.11. Statistical analysis
The results that were shown in all experiments are representative of at least three independent experiments and are expressed as the mean ± standard deviation (mean ± S.D.). The results were analyzed for statistically significant differences using Student's t-test and/or analysis of variance (ANOVA) followed by the Bonferroni multiple comparison post hoc test. p < 0.05 was considered statistically significant.
3. Results
3.1. The determination of the molecular mass and the sequence of the highly active antioxidant peptide generated from wheat germ albumin
In the current study, a two-step, dual-enzymatic hydrolysis and separation protocol was used to identify the antioxidant peptides from wheat germ albumin, resulting in the isolation of one antioxidant peptide, which was named AOP. The antioxidant activity of AOP in vitro was evaluated by the Fe2+ chelating ability assay. Hydrolysates derived from tryptic hydrolysis of wheat germ albumin were fractionated by ultrafiltration according to the molecular weight; then, the fractions with high antioxidant capacity were further isolated by gel filtration chromatography (Sephadex G-75). The fraction with a higher antioxidant activity was further hydrolyzed with alcalase. The hydrolysates were then subjected to purification by Sephadex G-15 gel filtration chromatography and reversed-phase HPLC (RP-HPLC). The highest antioxidant fraction was archived, and the structure was identified by QTOF-ESI-MS/MS. The m/z values of the single charged ions ([M + H]+) and the double charged ions ([M + 2H]2+) were 1014.55 and 507.78, respectively (Fig. 1A). Thus, the molecular mass of the highly active antioxidant peptide was 1013.51 Da. The amino acid sequence of this antioxidant peptide was identified as AREGETVVPG or RAEGETVVPG by tandem mass spectrometry (MS/MS) based on its y ion series of m/z 173.09 and 272.16 and its b ion series of m/z 228.15, 357.19, 414.21, 543.15, 644.30, 743.37, 842.44, and 939.49 (Fig. 1B and C). After performing protein database searches, the sequence of AOP was identified as AREGETVVPG.
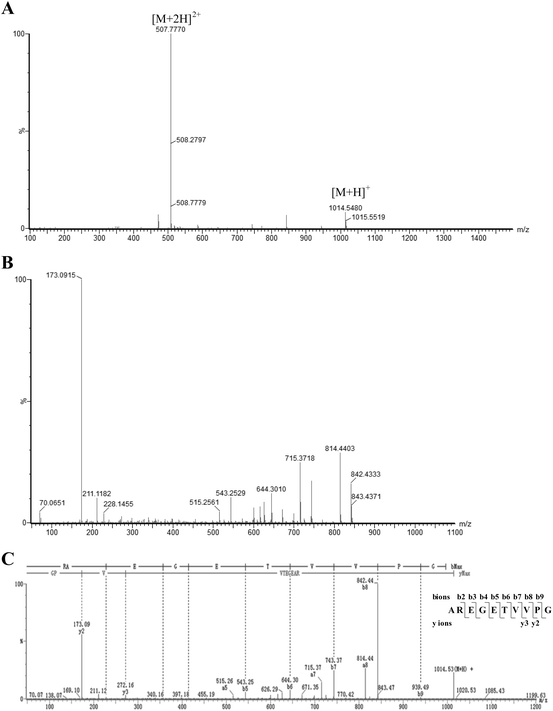 |
| Fig. 1 Sequence profile of the highly active AOP from hydrolysates of wheat germ albumin protein obtained in a QTOF-MS system (Waters Xevo G2-XS QTOF). (A) Mass spectrum of the selected fraction from HPLC. (B) TOF-MS/MS spectrum of ion m/z 1014.55 Da. (C) The information on the amino acid sequence displayed with the fragment ions; the ions of b and y are labeled. | |
3.2. AOP has a high antioxidant capacity in vitro
To confirm its antioxidant capacity, AREGETVVPG was chemically synthesized by the method of Fmoc solid-phase synthesis. The in vitro antioxidant capacity of AOP was measured utilizing the assay of Fe2+-chelating ability. As shown in Fig. 2, AOP exhibited Fe2+-chelating capacity in a concentration-dependent manner in the range from 0 to 1.6 mg ml−1. The corresponding IC50 value, which represents the concentration of AOP required to chelate 50% of Fe2+, was also determined. The IC50 values of the highly active AOP for Fe2+-chelating activities were 0.78 mg ml−1 (0.77 mM). However, AOP showed a lower Fe2+-chelating capacity than EDTA.
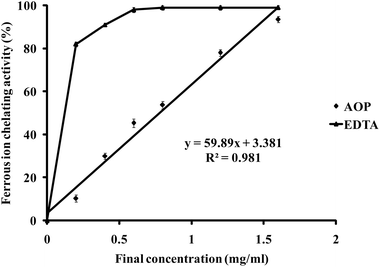 |
| Fig. 2 Ferrous ion-chelating capacity of AOP. EDTA was used as a positive control to compare the ferrous ion-chelating activity of AOP. | |
3.3. AOP inhibits ROS production in VSMCs exposed to high glucose
Our previous studies have shown that ROS generation is significantly increased in high glucose-challenged VSMCs (25 mM).23 Therefore, we determined whether the addition of AOP reduced the generation of ROS in VSMCs challenged by high glucose. As shown in Fig. 3, ROS generation was significantly increased by 22.4 ± 2.3% and 42.5 ± 5.0%, respectively, in the cells exposed to 25 mM glucose (HG) or to normal glucose (5 mM) with the addition of 200 μM H2O2 (NG + H2O2), compared to normal glucose (NG) (p < 0.01). However, high glucose-induced ROS generation was markedly prevented by AOP treatment (e.g., a 16.1 ± 5.1% decrease at 5 μM AOP, p < 0.05 and a 22.3 ± 4.6% decrease at 10 μM AOP, p < 0.01), which is comparable to that of a normal glucose condition (p > 0.05, NS).
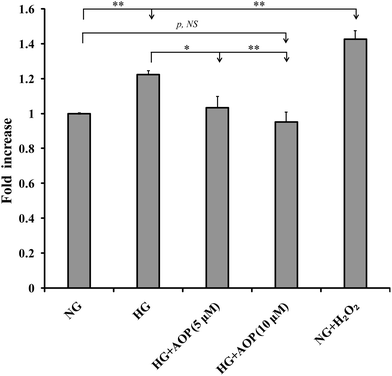 |
| Fig. 3 AOP inhibits high glucose-induced production of ROS in VSMCs. The intracellular generation of ROS in VSMCs with different treatments was measured as described under “Materials and methods.” Cells were treated with high glucose (HG), HG with AOP (HG + AOP), and/or normal glucose (5 mM) with H2O2 only (NG + H2O2, positive control). NG (5 mM glucose) served as a control. The mean fold increase in the production of ROS was shown; *p < 0.05 and/or **p < 0.01 significance compared to the either NG or HG group; NS indicates no significant difference between the two treatments. | |
3.4. AOP inhibits high glucose-stimulated PKCζ/Nox4/AKT and Erk1/2 signaling pathway and suppresses VSMC growth
To explore how AOP plays an antioxidant role in the survival and growth of VSMCs, the effects on the PKCζ/Nox4/ROS signaling pathway were determined. After starvation in a serum-free medium containing 5 mM glucose for 16 h, VSMCs were exposed to high glucose with or without AOP for 6 h. The results (Fig. 4A) demonstrated that the Nox4 protein expression was significantly increased in VSMCs exposed to high glucose (25 mM) (e.g., 276 ± 25%, p < 0.01, compared to those exposed to 5 mM glucose). The application of AOP at 5 μM significantly suppressed the Nox4 protein expression in cells exposed to high glucose (e.g., a 55.7 ± 15.2% decrease, p < 0.01), which is comparable to that of a normal glucose condition (p > 0.05, NS). Similarly, high glucose-induced PKCζ activation, indicated by Thr410 phosphorylation, was completely abolished by AOP treatment (p < 0.001) (Fig. 4B). Next, we determined the effect of AOP on IGF-I-induced AKT activation in high glucose. As shown in Fig. 4C and D, IGF-I-induced activation of AKT and phosphorylation of Erk1/2 were significantly attenuated by application of AOP at 5 μM in VSMCs exposed to high glucose (e.g., 33.8 ± 9.2% reduction, p < 0.05 and 55.5 ± 16.3% reduction, p < 0.05, respectively), which are comparable to the data from the VSMCs in a normal glucose condition (p > 0.05, NS). Moreover, the addition of AOP to the normal glucose cell culture media had no effect on the phosphorylation of PKCζ, AKT and Erk1/2, and did not inhibit the Nox4 protein expression (p > 0.05, NS) (Fig. 4, middle panels). Furthermore, as shown in Fig. 5, 25 mM glucose-challenged VSMCs exhibited a significant increase in cell growth compared to VSMCs in 5 mM glucose (NG) (64.6 ± 5.4%, p < 0.01). The addition of AOP efficiently suppressed the cell growth (e.g., 23.7 ± 3.1% decrease at 10 μM AOP, p < 0.05 and 37.4 ± 2.7% decrease at 30 μM AOP, p < 0.01, compared to high glucose). Furthermore, there was no significant difference (p > 0.05, NS) in cell growth between the cells treated with high glucose supplemented with AOP at 30 μM and those in the normal glucose condition, suggesting that AOP completely abolished the effect of high glucose on VSMC cell growth. Moreover, the addition of AOP (up to 30 μM) to the normal glucose cell culture media did not inhibit VSMC cell growth (p > 0.05, NS), indicating that AOP itself had no cytotoxic effect on VSMCs.
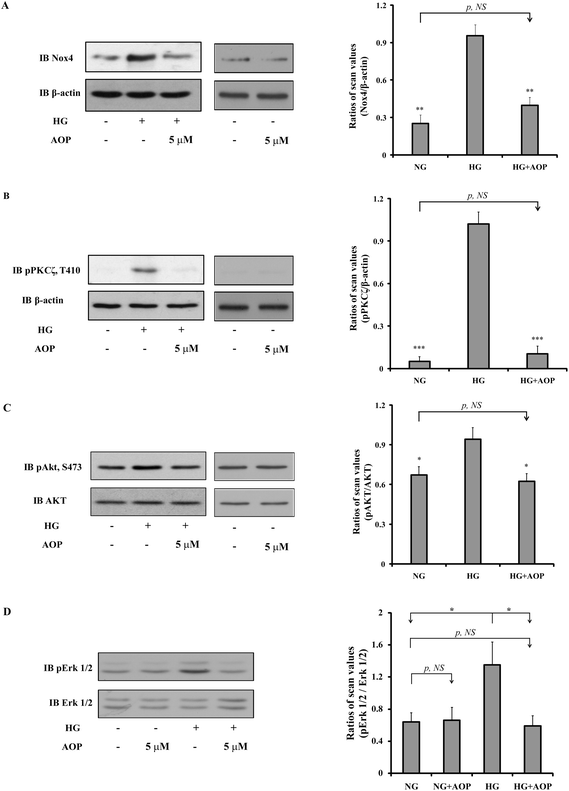 |
| Fig. 4 Effect of AOP on the high glucose-induced PKCζ/Nox4/AKT and Erk1/2 signaling pathways. VSMCs were cultured in DMEM with normal glucose (5 mM) plus 10% FBS and treated as described under “Materials and methods”. Cells were cultured in DMEM normal glucose (NG) and treated with AOP, high glucose (HG) or high glucose supplemented with AOP. Twenty microliters of cell lysates from the same experiment were used for detection of Nox4 expression levels, PKCζ and AKT activation by immunoblotting with (A) anti-Nox4, (B) anti-phospho-PKCζ at Thr410, (C) anti-phospho-AKT at Ser473, or (D) anti-phospho-Erk1/2 antibodies. For loading controls, the blots were stripped and reprobed with anti-β-actin (A and B), anti-AKT (C), or anti-Erk1/2 (D) antibodies. The protein levels were quantified using scanning densitometry and expressed as the relative ratio of the protein by its loading control. The figures are representative of three independent experiments. *p < 0.05, **p < 0.01, and ***p < 0.001 indicate significant differences between the two treatments; NS indicates no significant difference between two treatments. | |
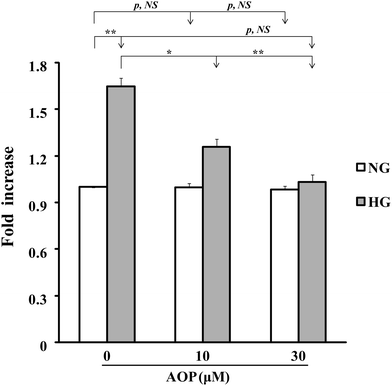 |
| Fig. 5 AOP inhibits high glucose-induced VSMC proliferation. Cell growth with different treatments was measured as described under “Materials and methods”. Cells were cultured in DMEM normal glucose (NG) and treated with AOP (10–30 μM), high glucose (HG) or high glucose supplemented with AOP (10–30 μM). The changes of VSMC cell growth are shown; NG (5 mM glucose) was used as a control. *p < 0.05 and **p < 0.01 indicate significant differences between two treatments; NS indicates no significant differences between the two treatments. | |
3.5. AOP is resistant to simulated gastrointestinal digestion
Finally, the stability of AOP against peptidase digestion in the GI tract was tested in a two-stage hydrolysis process. The digests were subjected to RP-HPLC. The results showed that there was no difference in the RP-HPLC profile of AOP before and after being treated in the simulated GI digestion system (Fig. 6). The results suggested that AOP with the sequence of AREGETVVPG was stable and might retain its activity well in the GI digestive system.
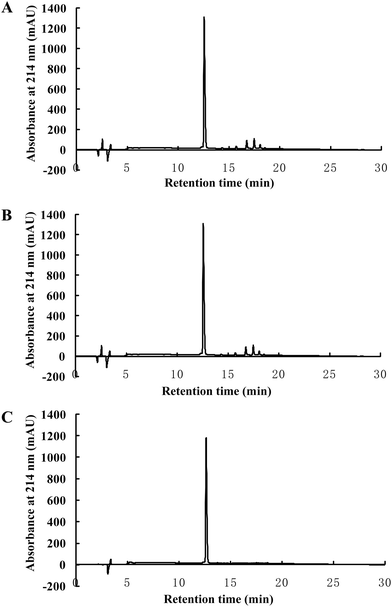 |
| Fig. 6 AOP is resistant to simulated gastrointestinal digestion in vitro. RP-HPLC chromatograms of AOP before (A) and after (B) simulated GI digestion. AOP without digestion was subjected to analysis by RP-HPLC as a positive control (C). | |
4. Discussion
Wheat germ proteins contain certain amino acid sequences with different biological activities; for example, VEV, NPPSV, QV, and AMY all have the angiotensin-converting enzyme (ACE)-inhibitory activity,29 whereas RVF exhibits high antioxidant capacity in vivo.11 These peptides are stable and easily absorbed in humans.30 In the present study we used a novel two-step, dual-enzymatic hydrolysis and separation protocol followed by QTOF-ESI-MS/MS and identified a highly antioxidant peptide with the sequence of AREGETVVPG from wheat germ albumin. This peptide exhibited a significantly high ferrous ion-chelating capacity compared to other antioxidant peptides reported previously.21
The most challenging task in research on bioactive peptides is the measurement of the antioxidant potential in vitro and in vivo, which would make mechanistic studies possible to better understand how those peptides play their antioxidant roles in biological systems.31,32 Different chemical-based assays have been developed, including the easy, simple and popular DPPH, Fe2+-chelating capacity and ORAC assays that evaluate the potential of an antioxidant by its ability to block the oxidation of a target molecule.33,34 However, the antioxidant capacity obtained by chemical-based in vitro assays cannot extrapolate the performance of the antioxidant in vivo. The antioxidant peptide functions not only as a scavenger of free radicals but also as a modulator of redox cell signaling and gene expression. The gold standard experiments are animal studies and human clinical trials. However, those are time consuming and costly. Thus, a cell-based assay is a more common and feasible method to evaluate the potential antioxidant activity of antioxidant peptides.
In the present study we used a high glucose-induced VSMC model to determine the role of AOP in the quenching of hyperglycemia-induced ROS, regulation of cell growth and gene expression. In the VSMC model, ROS generation is significantly increased when cells are exposed to hyperglycemia.22,23 High glucose increases the proliferation of VSMCs35 by enhancing the expression of growth factor receptors (such as PDGF and IGF-I receptors) via PI3K/MAPK pathways.36,37 Our results showed that the addition of AOP significantly diminished high glucose-induced ROS generation and cell proliferation in a concentration-dependent manner. In vascular cells, NADPH oxidase (Nox) is a major source of intracellular ROS.22 In particular, Nox4 is the predominant isoform that generates superoxide and its metabolic products38 depending upon protein kinase C ζ (PKCζ) activation.23 In mesangial cells, high glucose increased the Nox4 expression in a PKCζ-dependent manner.39 Our previous studies also showed that activated PKCζ phosphorylates p65 (RelA) on Ser311, subsequently activating NF-κB and leading to NOX4 synthesis in VSMCs.23,28 Therefore, we further explored the molecular mechanisms of AOP action in high glucose-challenged VSMCs by targeting ROS generation and the PKCζ/Nox4/ROS signaling pathway. Consistently, our current study clearly showed that high glucose significantly increased the Nox4 expression and PKCζ activation in VSMCs. However, the application of AOP completely reversed the process by inactivating PKCζ and subsequently reducing Nox4 expression, suggesting that AOP could have an important role in the PKCζ/Nox4/ROS signaling pathway. Therefore, the inhibitory effect of AOP on high glucose-induced ROS production could be due to the diminished Nox4 expression via decreased PKCζ activation.
The data showed that the purified AOP peptide reduced the intracellular ROS level, leading to the alteration of high glucose-induced PKCζ activation. This probably is due to a change of PKCζ protein oxidation level after peptide treatment. Inactivated PKCζ prevented high glucose-induced expression and activation of Nox4 and its mediating downstream signal events. However, the exact action site of the AOP peptide warrants further investigation. Our previous study has shown that protein oxidation is a prerequisite for Src kinase activation.23 Whether this is a case in PKCζ regulation by AOP requires further investigation.
Nox-dependent generation of ROS activates AKT and MAPK signaling through phosphorylation of AKT on Ser473 and Erk1/2 on Thr202/Tyr204 in VSMCs.23,40 The activation of MAPK and AKT is involved in cell proliferation and growth.23,41 Here we report that AOP inhibited ROS production, Erk1/2 and AKT phosphorylation, which might contribute to suppressed VSMC proliferation in hyperglycemia. AOP suppresses VSMC growth under hyperglycemia; based on this effect, AOP may have a potential application in the treatment of diabetic atherosclerosis.23,28,42 Because the stability of AOP against hydrolysis by peptidases in the GI tract is a critical feature for the function of AOP,43 a simulated digestion in vitro was performed. The results showed that AOP was stable and could not be hydrolyzed under the condition of simulated GI digestion, indicating that AOP may be able to resist physiological digestion after oral intake as a potential antioxidant. However, an in-depth study of the antioxidant mechanism and an in vivo, animal-based model of AOP action are greatly warranted in the future. In summary, our study identified a highly active antioxidant peptide, AOP, which has the amino acid sequence AREGETVVPG. To the best of our knowledge, this is the first report to demonstrate that the antioxidant peptide plays a key role in the prevention of hyperglycemia-induced ROS and in the inhibition of cell growth in VSMCs via regulation of the PKCζ/Nox4/ROS signaling pathway (Fig. 7). This study, therefore, provides insightful information for the development of antioxidant wheat germ peptides and their application in preventing diabetic atherosclerosis.
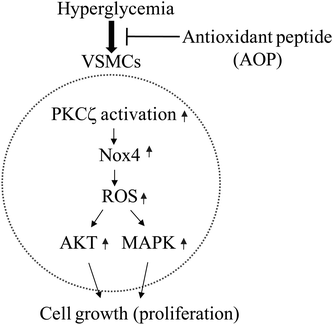 |
| Fig. 7 The possible molecular mechanism of AOP in inhibition of high glucose-induced reactive oxygen species (ROS) generation in VSMCs. High glucose (e.g., hyperglycemia) triggers PKCζ activation, resulting in enhanced expression of the Nox4 protein, which leads to elevated production of ROS. ROS may stimulate VSMC growth via activation of AKT and/or Erk signaling pathways. The application of AOP may abolish the hyperglycemia-induced growth of VSMCs through suppression of PKCζ activation directly or indirectly. | |
Conflict of interest
The authors declare no conflict of financial interests.
Abbreviations
AKT | Protein kinase B |
AOP | Antioxidant peptide |
DCF-DA | 2′,7′-Dichlorofluorescein diacetate |
DPPH | 2,2-Diphenyl-1-picrylhydrazyl |
Erk1/2 | Extracellular signal-related kinase 1/2 |
FBS | Fetal bovine serum |
GI | Gastrointestinal |
HBSS | Hank's balanced salt solution |
MTT | 3-(4,5-Dimethyl-2-thiazolyl)-2,5-diphenyl-2-H-tetrazolium bromide |
Nox4 | NADPH oxidase 4 |
PKCζ | Protein kinase C ζ |
RIPA | Radio-immunoprecipitation assay |
ROS | Reactive oxygen species |
RP-HPLC | Reversed phase HPLC |
VSMCs | Vascular smooth muscle cells |
Acknowledgements
We thank Dr Junyu Ning (Beijing Municipal Centers for Disease Prevention and Control, China) for providing the VSMCs for this research. This work was financially supported by the National Natural Science Foundation of China (31271983, 21476103). It was also supported in part by the Key Natural Science Foundation of the Jiangsu Higher Education Institutions of China (14KJA550002), the Natural Science Foundation of Jiangsu Province of China (BK20141484, BK20131435), the Universities Natural Science Research Project of Jiangsu Province (12KJB550003), the 2015 Jiangsu University Outstanding Science and Technology Innovation Team, and the Priority Academic Program Development of Jiangsu Higher Education Institutions (PAPD).
References
- A. Costabile, A. Klinder, F. Fava, A. Napolitano, V. Fogliano, C. Leonard, G. R. Gibson and K. M. Tuohy, Br. J. Nutr., 2008, 99, 110–120 CrossRef CAS PubMed.
- J. Wang, B. Sun, Y. Cao and Y. Tian, Food Chem. Toxicol., 2009, 47, 1591–1599 CrossRef CAS PubMed.
- B. Langkamp-Henken, C. Nieves Jr., T. Culpepper, A. Radford, S. A. Girard, C. Hughes, M. C. Christman, V. Mai, W. J. Dahl, T. Boileau, S. S. Jonnalagadda and F. Thielecke, J. Nutr., 2012, 142, 2025–2032 CrossRef CAS PubMed.
- M. Youn, A. S. Csallany and D. D. Gallaher, Br. J. Nutr., 2012, 107, 192–201 CrossRef CAS PubMed.
- C. I. Abuajah, A. C. Ogbonna and C. M. Osuji, J. Food Sci. Technol., 2014, 52, 2522–2529 CrossRef PubMed.
- A. Michalska, A. Ceglinska, R. Amarowicz, M. K. Piskula, D. Szawara-Nowak and H. Zielinski, J. Agric. Food Chem., 2007, 55, 734–740 CrossRef CAS PubMed.
- A. Fardet, Nutr. Res. Rev., 2010, 23, 65–134 CrossRef CAS PubMed.
- N. Mateo Anson, A. M. Aura, E. Selinheimo, I. Mattila, K. Poutanen, R. van den Berg, R. Havenaar, A. Bast and G. R. Haenen, J. Nutr., 2011, 141, 137–143 CrossRef PubMed.
- M. Whent, H. Huang, Z. Xie, H. Lutterodt, L. Yu, E. P. Fuerst, C. F. Morris, L. L. Yu and D. Luthria, J. Agric. Food Chem., 2012, 60, 2129–2135 CrossRef CAS PubMed.
- L. Y. Niu, S. T. Jiang and L. J. Pan, J. Food Sci. Technol., 2013, 50, 53–61 CrossRef CAS PubMed.
- Y. Cheng, L. Zhang, W. Sun, J. Tang, Z. Lv, Z. Xu and H. Yu, Biotechnol. Lett., 2014, 36, 1615–1622 CrossRef CAS PubMed.
- J. K. Willcox, S. L. Ash and G. L. Catignani, Crit. Rev. Food Sci. Nutr., 2004, 44, 275–295 CrossRef CAS PubMed.
- K. J. Barnham, C. L. Masters and A. I. Bush, Nat. Rev. Drug Discovery, 2004, 3, 205–214 CrossRef CAS PubMed.
- I. Suciu, V. Negrean and D. Sampelean, Rom. J. Intern. Med., 2004, 42, 395–406 CAS.
- H. J. Eisele, P. Markart and R. Schulz, Oxid. Med. Cell. Longevity, 2015 DOI:10.1155/2015/608438.
- S. J. Lee, Y. S. Kim, J. W. Hwang, E. K. Kim, S. H. Moon, B. T. Jeon, Y. J. Jeon, J. M. Kim and P. J. Park, Food Res. Int., 2012, 49, 285–295 CrossRef CAS.
- M. Memarpoor-Yazdi, A. Asoodeh and J. K. Chamani, J. Funct. Foods, 2012, 4, 278–286 CrossRef CAS.
- R. W. Yang, J. Wang and S. Y. Lin, Adv. Mater. Res., 2014, 881–883, 811–814 CrossRef.
- R. Coda, C. G. Rizzello, D. Pinto and M. Gobbetti, Appl. Environ. Microbiol., 2012, 78, 1087–1096 CrossRef CAS PubMed.
- B. H. Sarmadi and A. Ismail, Peptides, 2010, 31, 1949–1956 CrossRef CAS PubMed.
- K. Zhu, H. Zhou and H. Qian, Process Biochem., 2006, 41, 1296–1302 CrossRef CAS.
- S. H. Ellmark, G. J. Dusting, M. N. Fui, N. Guzzo-Pernell and G. R. Drummond, Cardiovasc. Res., 2005, 65, 495–504 CrossRef CAS PubMed.
- G. Xi, X. Shen, L. A. Maile, C. Wai, K. Gollahon and D. R. Clemmons, Diabetes, 2012, 61, 104–113 CrossRef CAS PubMed.
- T. Hatami, S. A. Emami, S. S. Miraghaee and M. Mojarrab, Iran. J. Pharm. Res., 2014, 13, 551–559 CAS.
- M. Jovani, R. Barbera, R. Farre and E. Martin de Aguilera, J. Agric. Food Chem., 2001, 49, 3480 CrossRef CAS PubMed.
- A. Gockerman, T. Prevette, J. I. Jones and D. R. Clemmons, Endocrinology, 1995, 136, 4168–4173 CAS.
- Y. Ling, L. A. Maile, J. Lieskovska, J. Badley-Clarke and D. R. Clemmons, Mol. Biol. Cell, 2005, 16, 3353–3364 CrossRef CAS PubMed.
- G. Xi, X. Shen, C. Wai, C. K. Vilas and D. R. Clemmons, FASEB J., 2015, 29, 4772–4782 CrossRef CAS PubMed.
- R. Yang, Y. Zou, N. Yu and Z. Gu, J. Agric. Food Chem., 2011, 59, 3598–3605 CrossRef CAS PubMed.
- D. F. Dai, T. Chen, H. Szeto, M. Nieves-Cintron, V. Kutyavin, L. F. Santana and P. S. Rabinovitch, J. Am. Coll. Cardiol., 2011, 58, 73–82 CrossRef CAS PubMed.
- C. C. Udenigwe and R. E. Aluko, J. Food Sci., 2012, 77, R11–R24 CrossRef CAS PubMed.
- X. Kou, J. Gao, Z. Xue, Z. Zhang, H. Wang and X. Wang, LWT–Food Sci. Technol., 2013, 50, 591–598 CrossRef CAS.
- M. N. Alam, N. J. Bristi and M. Rafiquzzaman, Saudi Pharm. J., 2013, 21, 143–152 CrossRef PubMed.
- C. Lopez-Alarcon and A. Denicola, Anal. Chim. Acta, 2013, 763, 1–10 CrossRef CAS PubMed.
- W. F. Graier, I. Grubenthal, P. Dittrich, T. C. Wascher and G. M. Kostner, Eur. J. Pharmacol., 1995, 294, 221–229 CrossRef CAS PubMed.
- M. Campbell, W. E. Allen, J. A. Silversides and E. R. Trimble, Diabetes, 2003, 52, 519–526 CrossRef CAS PubMed.
- Y. Radhakrishnan, L. A. Maile, Y. Ling, L. M. Graves and D. R. Clemmons, J. Biol. Chem., 2008, 283, 16320–16331 CrossRef CAS PubMed.
- F. Perez-Vizcaino, A. Cogolludo and L. Moreno, Respir. Physiol. Neurobiol., 2010, 174, 212–220 CrossRef CAS PubMed.
- L. Xia, H. Wang, S. Munk, J. Kwan, H. J. Goldberg, I. G. Fantus and C. I. Whiteside, Am. J. Physiol.: Renal. Physiol., 2008, 295, F1705–F1714 CrossRef CAS PubMed.
- A. N. Lyle and K. K. Griendling, Physiology, 2006, 21, 269–280 CrossRef CAS PubMed.
- P. A. Suwanabol, S. M. Seedial, F. Zhang, X. Shi, Y. Si, B. Liu and K. C. Kent, Am. J. Physiol.: Heart Circ. Physiol., 2012, 302, H2211–H2219 CrossRef CAS PubMed.
- M. L. Ward and D. J. Crossman, World J. Cardiol., 2014, 6, 577–584 CrossRef PubMed.
- F. Jahandideh, S. Chakrabarti, S. T. Davidge and J. Wu, J. Agric. Food Chem., 2016, 64, 113–119 CrossRef CAS PubMed.
|
This journal is © The Royal Society of Chemistry 2017 |