DOI:
10.1039/C6FO01073C
(Paper)
Food Funct., 2017,
8, 132-141
Solanesol induces the expression of heme oxygenase-1 via p38 and Akt and suppresses the production of proinflammatory cytokines in RAW264.7 cells
Received
18th July 2016
, Accepted 18th November 2016
First published on 21st November 2016
Abstract
The aim of the present study was to examine the anti-inflammatory effect of solanesol and to elucidate the underlying mechanisms. Heme oxygenase-1 (HO-1) plays an important role in cytoprotection against oxidative stress and inflammation. Solanesol induced HO-1 expression both at the level of mRNA and proteins, resulting in increased HO-1 activity. Solanesol treatment enhanced the level of the phosphorylated form, nuclear translocation, ARE-binding, and transcriptional activity of Nrf2. p38 and Akt contributed to ARE-driven HO-1 expression. Solanesol activated both p38 and Akt, and treatments with SB203580 (a p38 kinase inhibitor), LY294002 (an Akt inhibitor), specific p38 siRNA and Akt siRNA suppressed the solanesol-induced activation of Nrf2, resulting in a decrease in HO-1 expression. Solanesol also elevated the autophagic protein LC3B-II level. SnPP (a HO-1 inhibitor) and HO-1 siRNA markedly abolished the anti-inflammatory effect of solanesol against LPS-induced cell damage. Likewise, SB203580, LY294002, 3-MA and Baf-A1 inhibited the solanesol-induced anti-inflammatory effect. These studies demonstrate that solanesol attenuates inflammation by HO-1 induction via p38 and Akt signaling. Thus, it is quite plausible that HO-1 induction by solanesol could trigger anti-inflammatory pathways including limiting LPS-stimulated cytokine production through autophagic signaling via p38 and Akt.
1. Introduction
HO-1 (heme oxygenase-1) is the inducible isoform of the rate-limiting enzymatic step of heme catabolism, and catalyzes the oxidative cleavage of heme into carbon monoxide, iron, and biliverdin.1 There is accumulating evidence that upregulation of HO-1 protects against atherosclerosis, oxidation and inflammation.2 HO-1 expression in macrophages constitutes an important component of its antiatherogenic effect.3 Induction of HO-1 by oxidative stress provides antioxidant cytoprotective effects.4 HO-1 has also been shown to be involved in anti-inflammatory functions. Overexpression of HO-1 via pharmacological modulation or gene therapeutic approaches has been shown to have beneficial effects in various experimental models of inflammation including sepsis and colitis.5,6 These salutary effects of HO-1 are mediated by the endproducts of heme degradation. Biliverdin and its metabolite confer potent cellular antioxidant capacities and CO acts as an important signaling molecule with anti-apoptotic and anti-inflammatory effects.7–9 Consistent with the in vitro results, HO-1 deficient mice not only exhibited pro-inflammatory cytokines but also were highly sensitive to the toxicity of LPS, leading to chronic inflammation.10,11 These studies indicate that HO-1 may serve as a therapeutic target in inflammatory diseases.
HO-1 is regulated transcriptionally via signaling pathways involved in survival and stress responses in different cell types. PI3K/Akt, MAPKs including p38, JNK, and ERK, as well as Nrf2 promote HO-1 expression.4,12 However, the anti-inflammatory effect of HO-1 may be associated with multiple signaling pathways.
Solanesol is a 45-carbon isoprenoid, found in tomatoes, potatoes, eggplant and pepper.13 Solanesol is known to exert anti-bacterial, anti-inflammation, and anti-ulcer activity.14 In the present study, we investigated the capability of solanesol to upregulate HO-1 expression via activation of p38 and Akt. Furthermore, HO-1 induction by solanesol could trigger anti-inflammatory pathways including limiting LPS-stimulated cytokine production through autophagic signaling via p38 and Akt.
2. Materials and methods
2.1. Reagents and antibodies
Solanesol, LPS (from Escherichia coli 0111: B4), SB203580, LY294002, 3-MA and Baf-A1 were obtained from Sigma. SnPP was obtained from Santa Cruz Biotechnology. Antibodies to HO-1, Nrf2, GAPDH and Lamin B were obtained from Santa Cruz Biotechnology. Phosphoserine/phosphothreonine, phospho-Akt (Ser473), Akt, polyclonal JNK/SAPK, phospho-JNK/SAPK (Thr183/Tyr185), p38MAPK, phospho-p38MAPK (Thr180/Tyr182), p42/p44MAPK, phospho-p42/44MAPK (Thr202/Tyr204), and LC3B antibodies were purchased from Cell Signaling Technology. All secondary antibodies were from Rockland Immunochemicals.
2.2. Cell culture and transfection
RAW264.7 murine macrophage-like cells, purchased from the CBCAS (Cell Bank of the Chinese Academy of Sciences, Shanghai, PR China), were cultured in DMEM (Invitrogen) containing 10% (v/v) fetal bovine serum (Hyclone) and antibiotics (100 U ml−1 penicillin and 100 μg ml−1 streptomycin) (Hyclone) at 37 °C under an atmosphere of 5% CO2. Transient transfection was performed using Lipofectamine 2000 (Invitrogen) according to the manufacturer's instructions. In all cases, the total amount of DNA was normalized by the addition of empty control plasmids. The overall transfection efficiency for cells assessed by X-Gal staining or flow cytometry assay was 35–50%.
2.3. Western blot analysis
Cells were rinsed twice with ice-cold PBS, and solubilized in lysis buffer containing 20 mM Tris (pH 7.5), 135 mM NaCl, 2 mM EDTA, 2 mM DTT, 25 mM β-glycerophosphate, 2 mM sodium pyrophosphate, 10% glycerol, 1% Triton X-100, 1 mM sodium orthovanadate, 10 mM NaF, 10 μg ml−1 aprotinin, 10 μg ml−1 leupeptin, and 1 mM PMSF for 30 min on ice. Lysates were centrifuged (15
000g) at 4 °C for 10 min. Equal amounts of the soluble protein are denatured in SDS, electrophoresed on an 11% SDS-polyacrylamide gel, and transferred to nitrocellulose membranes. The antibody–antigen complexes were visualized by using the LI-COR Odyssey Infrared Imaging System according to the manufacturer's instructions using an IRDye 800 fluorophore-conjugated antibody (LI-COR Biosciences, Lincoln, NE). Quantification was directly performed on the blot using the LI-COR Odyssey Analysis software.
2.4. RNA extraction and real-time PCR
Total RNA was isolated from RAW264.7 cells using TRIzol reagent (Invitrogen). 1 μg of total RNA was reverse-transcribed using M-MuLV reverse transcriptase. Real-time quantitative PCR was performed using the SYBR Green PCR Master Mix and the StepOnePlus real-time PCR system (Applied Biosystems) according to the manufacturer's protocol. The relative quantity of target mRNA was determined using the comparative threshold (Ct) method by normalizing target mRNA Ct values to those for GAPDH (ΔCt). The real-time PCR cycling conditions were as follows: 95 °C for 5 min, followed by 40 cycles of 30 s at 95 °C, 20 s at 55 °C, and 30 s at 72 °C. The primer sequences are as follows: HO-1-sense (5′-ACAGGTTGACAGAAGAGGCTAA-3′), HO-1-antisense (5′-AACAGGAAGCTGAGAGTGAGG-3′), GAPDH-sense (5′-AGGTGGTCTCCTCTGACTTC-3′), and GAPDH-antisense (5′-TACCAGGAAATGAGCTTGAC-3′).
2.5. Nrf2 activation analysis
The levels of Nrf2-ARE binding were measured in nuclear proteins using a Trans AM Nrf2 kit (Active Motif, Carlsbad, CA). According to the protocol provided by the manufacturer, 10 mg of nuclear protein were incubated in 96-well plates pre-coated with ARE (Antioxidant Response Element) consensus oligonucleotides (5′-GTCACAGTACTCAGCAGAATCTG-3′), and the active Nrf2 that bound to the oligonucleotides was detected by incubating with anti-Nrf2 antibodies and an appropriate HRP-conjugated secondary antibody. The final product formed as a result of the specific activity of the transcription factor in the nuclear extracts was read using a plate reader at 450 nm, and absorbance was expressed as the direct activity of Nrf2.
2.6. Cytoplasmic and nuclear extract preparation
Cells were washed with ice-cold PBS and then lysed with hypotonic buffer (20 mM HEPES (pH 7.9), 10 mM KCl, 1 mM EDTA, 0.1 mM EGTA, 1 mM DTT, 10% glycerol, 0.1 mM Na3VO4, 1 mM PMSF, and a protein inhibitor) with 0.2% NP-40 on ice for 10 min. To separate the cytoplasmic and nuclear fractions, cell pellets were processed using the NE-PER nuclear and cytoplasmic extraction kit (Pierce) according to the manufacturer's instructions. Protein concentration was determined using a Bio-Rad protein assay kit.
2.7. Transient transfection of small RNA interference (siRNA)
Control siRNA and siHO-1 were purchased from Invitrogen. siNrf2, siAkt and sip38 were acquired from Santa Cruz Biotechnology. siRNA was transfected into RAW 264.7 cells using Lipofectamine 2000 (Invitrogen) according to the manufacturer's instructions. The cells were incubated with 100 nM of target siRNA or control siRNA for 24 h, and pretreated with or without solanesol, following LPS stimulation.
2.8. HO-1 activity assay
HO-1 enzyme activity was measured using a reaction mixture containing NADPH, rat liver cytosol as a source of biliverdin reductase, and hemin as the substrate as described previously.15 The reaction was carried out in the dark for 1 h at 37 °C, and the amount of the extracted bilirubin was calculated by the difference in absorbance between 464 and 530 nm.
2.9. Determination of cytokines
ELISA kits were used to measure the concentrations of TNF-α, IL-6, and IL-1β (R&D Systems Minneapolis, MN) according to the manufacturers’ protocols.
2.10. Statistics
Analysis of variance (ANOVA) was used to compare the results between two groups. Individual points were compared using a Student's t test and differences were considered significant for p < 0.05. Data are presented as means ± SD. Western blotting analysis experiments were repeated 3 times with similar trends.
3. Results
3.1. Solanesol induces HO-1 expression and activity in RAW264.7 cells
The upregulation of HO-1 expression has been demonstrated to exert an anti-inflammatory effect.1 Therefore, we examined the effect of solanesol on HO-1 mRNA and protein expression in RAW264.7 cells. Cells treated with 40 μM solanesol induced a time-dependent increase in HO-1 mRNA, and the HO-1 mRNA levels gradually increased in a dose-dependent manner (Fig. 1A and B). In agreement with the HO-1 mRNA expression, treatment with solanesol resulted in a time-and dose-dependent increase in HO-1 protein levels (Fig. 1C and D). The effects of solanesol on HO-1 activity in RAW264.7 macrophages were also assessed. Exposure of the cells to 40 μM solanesol resulted in increased HO activity. A significant increase in HO-1 activity was observed at 20 and 40 μM of solanesol (Fig. 1E and F). These results suggest that solanesol enhances HO-1 expression and activity.
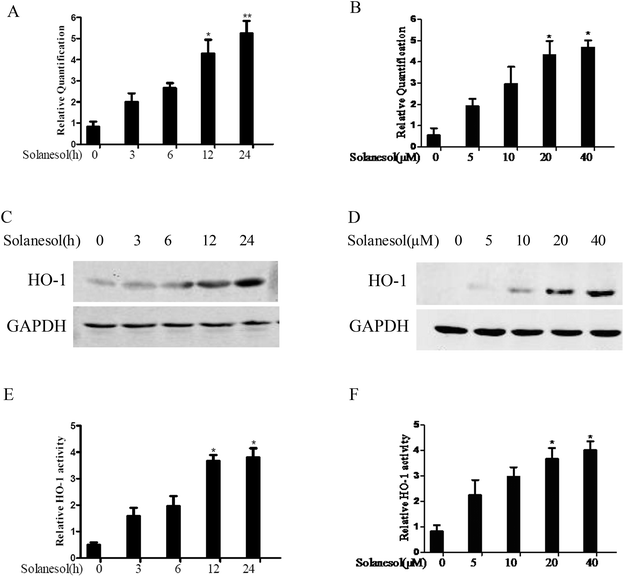 |
| Fig. 1 The effect of solanesol on the expression and enzyme activity in RAW264.7 cells. (A) Cells were cultured with 40 μM solanesol for the indicated times or (B) different concentrations of solanesol for 12 h. Relative HO-1 mRNA expression was determined using real-time PCR and calculated by subtracting the Ct value for GAPDH from the Ct value for HO-1. (C) Cells were cultured for various times with solanesol (40 μM) or (D) incubated with the indicated concentration of solanesol. Total cellular extracts were prepared and detected by western blotting. (E) HO-1 activity was measured for a variety of time courses of solanesol with 40 μM, and (F) with various concentrations of solanesol at 12 h. Each bar represents the mean ± SEM in triplicate experiments. *P < 0.05; **P < 0.01 compared with the control group. | |
3.2. Solanesol promotes Nrf2 nuclear translocation and transactivity in RAW264.7 cells
Nrf2 has been suggested to play a central role in HO-1 induction in response to numerous stimuli.16 To determine whether solanesol induced the nuclear translocation of Nrf2 in association with HO-1 upregulation in macrophages, RAW264.7 cells were exposed to 40 μM solanesol for different durations, and then western blot analysis was performed using cytosolic and nuclear extracts. As shown in Fig. 2A and B, solanesol treatment increased Nrf2 nuclear accumulation in a time- or dose-dependent manner. Furthermore, the cytosolic protein levels of Nrf2 induced by solanesol were reduced (Fig. 2C and D). To confirm the requirement of Nrf2 for solanesol-induced HO-1 expression, cells were transfected with siNrf2 for 24 h prior to solanesol treatment. As shown in Fig. 2E and F, both the constitutive and solanesol-induced expressions of HO-1 were markedly inhibited by siRNA knock down of the Nrf2 gene. To test Nrf2 phosphorylation, the nuclear lysates were immunoprecipitated with the anti-Nrf2 antibody and immunoblotted with the phosphoserine/phosphothreonine antibody. The phosphorylated Nrf2 was clearly increased in the cells treated with solanesol (Fig. 2G), and resulted in a translocation of the Nrf2 protein from the cytosol to the nucleus.
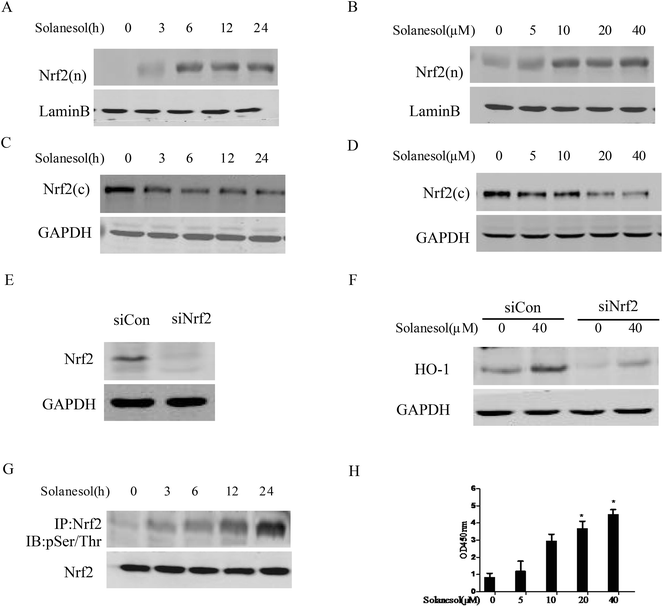 |
| Fig. 2 Effects of solanesol on Nrf2 activation in RAW264.7 cells. (A)–(D) Cells were pretreated with solanesol (40 μM) for the indicated times or pretreated with the indicated concentration of solanesol for 12 h. Cytosolic (c) and nuclear (n) extracts were isolated, and the levels of Nrf2 in each fraction were determined by western blotting. Lamin B and GAPDH were used as internal controls. The experiment was repeated three times, and similar results were obtained. (E)–(F) Cells were transfected in 100 nM siControl and siNrf2. At 24 h after transfection, the cells were treated with solanesol (40 μM) for 12 h and the expression of the HO-1 protein was examined by western blot analysis. (G) The cell lysates were immunoprecipitated with the Nrf2 antibody, and precipitates were western blotted with antibodies against phosphoserine/phosphothreonine and Nrf2. (H) Nuclear extracts prepared from cells were treated with the indicated concentration of solanesol for 12 h. TransAM Nrf2 kit was used for the detection of the ARE-binding activity of Nrf2. *P < 0.05; compared with the control group. | |
To examine the effect of solanesol on Nrf2 transactivity, we performed the analysis of the ARE-binding activity of Nrf2 using the TransAM Nrf2 kit. AREs are transcriptional control elements that mediate the expression of a set of antioxidant proteins. As shown in Fig. 2H, treatment with 40 μM solanesol significantly increased ARE promoter activity in RAW264.7 cells. These results suggest that solanesol activates Nrf2 nuclear translocation and transactivation that mediate HO-1 expression.
3.3. Solanesol activates Nrf2 by phosphorylation of p38 and Akt
To explore the upstream signaling pathway involved in solanesol-mediated Nrf2 activation and induction of HO-1, we tested the effects of p38 and Akt pathways on the upregulation of HO-1 gene expression by solanesol. As shown in Fig. 3A and D, exposure to solanesol caused an increase in the phosphorylation of p38 and Akt in the RAW264.7 cells. However, the phosphorylated forms of ERK and JNK were not affected by solanesol treatment in RAW264.7 cells (Fig. 3B and C).
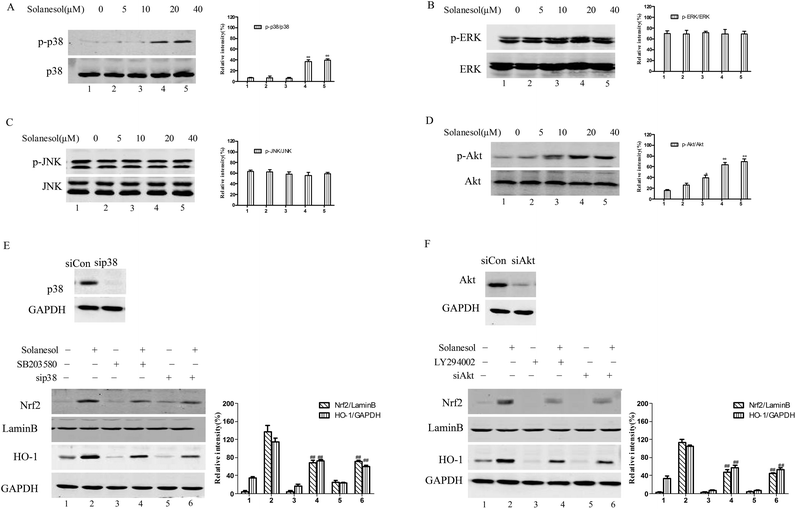 |
| Fig. 3 Induction of HO-1 and activation of Nrf2 by solanesol via phosphorylation of p38 and Akt. Cells were treated with solanesol for 12 h: (A) phospho-p38, p38, (B) phospho-Erk, ERK, (C) phospho-JNK, JNK and (D) phospho-Akt, Akt were detected using their respective specific antibodies. (E) Preincubated with SB203580 (10 μM) for 30 min or transfected in 100 nM siControl, sip38 for 24 h and then treated with solanesol for an additional 12 h, and the expression of nuclear Nrf2 and HO-1 protein was examined by western blot analysis. (F) Preincubated with LY294002 (25 μM) for 30 min or transfected in 100 nM siControl, siAkt for 24 h and then treated with solanesol for an additional 12 h, and the expression of nuclear Nrf2 and HO-1 protein was examined by western blot analysis. The results are expressed as mean ± SD of three independent experiments. *P < 0.05; **P < 0.01 compared with the control group. ##P < 0.01 compared with control cells treated with solanesol. | |
To further confirm the effect of solanesol on Nrf2 and HO-1 expression via the p38 and Akt signaling pathways, we used pharmacological inhibitors (p38, SB203580; PI3K/Akt, LY294002), sip38 and siAkt. Cells were treated with the indicated inhibitor for 30 min or 100 nM siRNA before exposure to 40 μM solanesol. As shown in Fig. 3E and F, cells pretreated with inhibitors or siRNA suppressed Nrf2 and HO-1 expression induced by solanesol. Our data suggest that inhibition of the p38 and Akt pathways dramatically reduced the capacity of solanesol to increase Nrf2 and HO-1 protein levels.
3.4. Solanesol enhances LPS-induced autophagic protein LC3B-II expression in RAW264.7 cells
HO-1 has been reported to be involved in LPS-induced autophagic signaling in macrophages.17 Thus, the effect of solanesol on the autophagic protein LC3B was assessed. Solanesol enhanced the LPS-induced increase of the LC3B-II level in a dose-and time-dependent manner, indicating an enhancement of the autophagic response (Fig. 4A and B). The enhanced autophagy by solanesol in LPS-stimulated macrophages was markedly attenuated in the presence of 3-MA, the specific inhibitor of the autophagic process, and confirmed by the further increase of LC3B-II levels in the presence of Baf-A1(a proton pump inhibitor), which interferes with the fusion between autophagosomes and lysosomes, leading to inhibition of LC3B-II degradation (Fig. 4C).
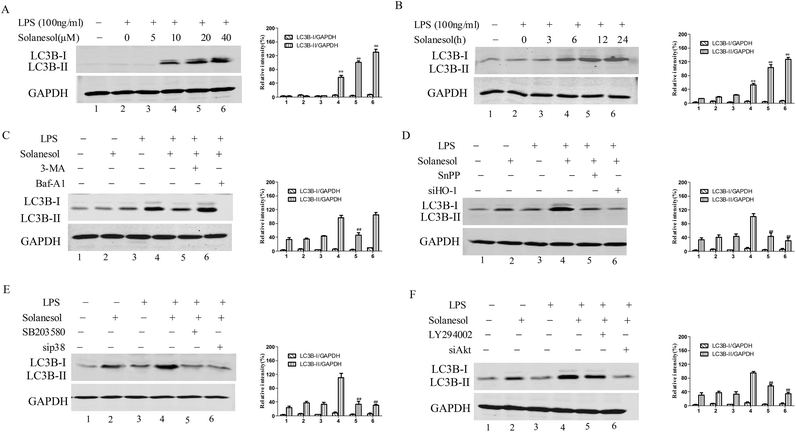 |
| Fig. 4 Effects of solanesol on the LPS-induced LC3B in RAW 264.7 macrophages. (A) Cells were pretreated with solanesol for 12 h with indicated concentrations and (B) treated with 40 μM solanesol for indicated times in the presence of LPS (100 ng ml−1). (C) Cells were pretreated with solanesol (40 μM), 3-MA (1 mM) or Baf-A1 (10 nM) for 12 h in the presence of LPS (100 ng ml−1). (D–F) Cells were pretreated or transiently transfected with indicated siRNA or inhibitors, and then followed by solanesol for 12 h in the presence of LPS (100 ng ml−1). Whole cell lysates were separated by SDS-PAGE and then immunoblotting was performed with anti-LC3B and GAPDH antibodies. The results are expressed as mean ± SD of three independent experiments. **P < 0.01 compared with control cells. ##P < 0.01 compared with control cells treated with solanesol/LPS. | |
To address the relevance of LC3B to HO-1, p38 and Akt, the inhibitors (SnPP, SB203580 and LY294002) and siRNA (siHO-1, sip38 and siAkt) were added or transfected to evaluate the effect of LC3B. Fig. 4D–F clearly show that this pharmacological or genetic blockade downregulated the LPS-induced LC3B-II level. Altogether, these data indicated that solanesol significantly enhanced autophagy in LPS-activated cells.
3.5. HO-1 mediates the inhibitory effect of solanesol on the production of proinflammatory cytokines in RAW264.7 cells
To elucidate whether the inhibitory effects of solanesol on the production of pro-inflammatory cytokines are mediated by the HO-1 signaling pathway, we utilized SnPP or HO-1 siRNA as HO-1 enzyme inhibitors. LPS stimulation of RAW264.7 increased the levels of IL-6, IL-1β and TNF-α. Inhibition of HO-1 impeded the solanesol-mediated suppression of IL-6, IL-1β and TNF-α production, indicating that solanesol-dependent HO-1 activation plays an important role in the downregulation of inflammatory responses (Fig. 5A and B). In accordance with the findings, the blockage with the inhibitors or siRNA of p38 and Akt also reversed pro-inflammatory cytokine production (Fig. 5C–F). Moreover, the addition of 3-MA or Baf-A significantly increased the production of cytokines following LPS stimulation in the solanesol-treated cells, confirming that solanesol can indeed stimulate autophagy (Fig. 5G and H). These data suggest that solanesol-induced HO-1 may limit LPS-stimulated cytokine production through autophagic signaling via p38 and Akt.
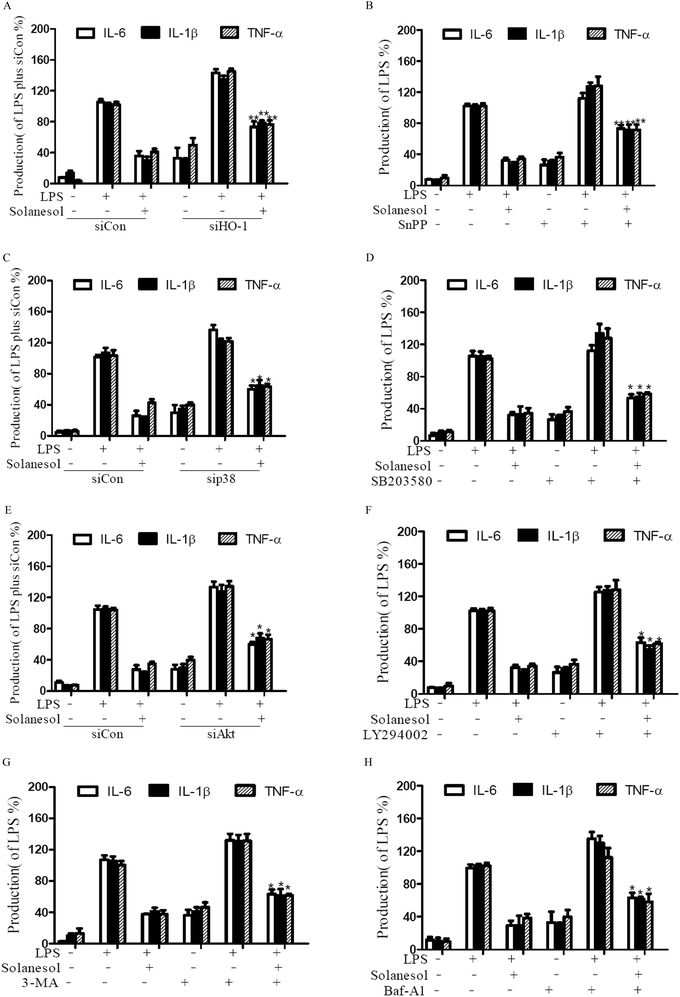 |
| Fig. 5 Effects of solanesol on IL-6, IL-1β, and TNF-α production in LPS-induced RAW264.7 cells. (A) Cells were transfected in 100 nM siControl and siHO-1 or (C) sip38 or (E) siAkt, after 24 h transfection, followed by solanesol in the presence of LPS (100 ng ml−1) for 24 h. (B) Cells were preincubated with SnPP, (D) SB203580, (F) LY294002, (G) 3-MA, and (H) Baf-A1 for 6 h, followed by solanesol in the presence of LPS (100 ng ml−1) for 24 h. The production of IL-6, IL-1β and TNF-α was determined. The results are expressed as mean ± SD of three independent experiments. *P < 0.05; **P < 0.01 compared with control cells treated with solanesol/LPS. | |
4. Discussion
In the current study, we showed that solanesol possesses anti-inflammatory properties through the activation of the p38 and Akt pathways, which appears to have contributed to the nuclear translocation of Nrf2, its subsequent binding to ARE, and the upregulation of HO-1 gene expression. Moreover, solanesol has also been demonstrated to elevate autophagic protein LC3B-II levels. Thus, it is quite plausible that HO-1 induction by solanesol could trigger anti-inflammatory pathways including limiting LPS-stimulated cytokine production through autophagic signaling via p38 and Akt.
Nrf2 plays an essential role in the expression of HO-1 in response to oxidative stress and inflammation.16 There have been several studies suggesting that phosphorylation of Nrf2 may contribute to its nuclear translocation and transcriptional activity.18–20 Our results demonstrated that the increase in the nuclear levels of Nrf2 including phosphorylation of Nrf2 induced by solanesol was dependent on the activation of p38 and Akt. Little is known about the interplay between the two kinase pathways and how they may coordinate to contribute to the regulation of the Nrf2 pathway. Since GSK3β is a common downstream target of Akt and MAPK, it may act as a key regulator of the activation of Nrf2 by phosphorylation.21 Furthermore, we found that p38 inhibitors (SB203580 or sip38) or Akt inhibitors (LY294002 or siAkt) only partially prevented the inhibitory effects of solanesol on LPS-induced pro-inflammatory cytokine production, indicating that other pathways may be involved in HO-1 induction such as protein kinase C (PKC). Therefore, relative contributions of Akt and p38 pathways to HO-1 regulation may result in discrepancy. The intimate connections between p38 and Akt in solanesol-induced HO-1 upregulation are currently under intense investigation. It is not clear whether solanesol crosses the cellular membrane and binds to specific intracellular receptors, especially the receptors associated with inflammation, such as LXR,22,23 PPARγ,24–26 and SHP,27 which in turn activate p38 and Akt.
The activation of autophagic pathways in macrophages may serve to limit the inflammatory response and restore cellular homeostasis.28,29 Our observations indicated that solanesol decreased the production of inflammatory cytokines, which is associated with the induction of autophagy by the HO-1 pathway. Several studies indicated that a variety of signaling pathways including p38, Akt, Nrf2 and HO-1 are also implicated in the regulation of autophagy in various cells.30–34 In addition, these signaling molecules may have multiple entry points into the autophagic pathway in a cell-and compound-specific fashion depending upon a combination of these factors. Thus, whether solanesol-stimulated autophagy is involved in these crosstalking pathways remains elusive. We also showed that siHO-1, Snpp or 3-MA, and Baf-A only partially prevented the inhibitory effects of solanesol on LPS-induced pro-inflammatory cytokine production, suggesting that solanesol seems to regulate inflammatory responses through HO-1 or autophagy-independent pathways, such as the STAT3 pathway. STAT3 has been identified as the key mediator of the anti-inflammatory effects of IL-10, which controls the expression of proinflammatory cytokines.35
In the present study, we emphasize that HO-1 and HO-1-dependent autophagy plays the dominant role in solanesol-induced anti-inflammatory effects in RAW264.7 cells. The current study using the murine macrophage-like cell model needs to be confirmed as being relevant to human cells such as THP-1 cells and peripheral blood mononuclear cells (PBMCs). Further in vivo studies using solanesol in combination with conventional anti-inflammatory agents remain to be fully determined. Autophagy has been shown to participate in chronic inflammatory diseases and autoimmune diseases and in combating against infections.36,37 Defective autophagy increases the possibility of viral and bacterial infection.38 Hence, promotion of HO-1 and HO-1-dependent autophagy by solanesol may play a role in the treatment of sepsis, Crohn's disease and gastrointestinal inflammation, which needs further investigation.
We have shown recently that solanesol protects human hepatic L02 cells from ethanol-induced oxidative injury via upregulation of HO-1 and Hsp70, suggesting that HO-1 induction is a universal mechanism of solanesol in different cells.39 The regulatory effect of HO-1-mediated autophagy during liver injury remains unclear. Carchman et al. reported that sepsis or LPS-induced autophagy protects against hepatocellular death, in part via an HO-1-dependent signaling.40 Liu et al. demonstrated that baicalein prevents hepatocellular injury via induction of HO-1-mediated autophagy.41 Therefore, it is possible that the protective action of solanesol could result from its enhancement of HO-1-dependent autophagy in hepatocytes. Additionally, little is known regarding the interaction of Hsp70 and autophagy. A study has shown that Hsp70 activation and autophagy induction are opposing processes,42 while autophagy and Hsp70 can coordinate and undergo sequential activation and downregulation.43 These data support the view that the interaction between autophagy and Hsp70 is complex. Thus, further studies are necessary to identify the interplay between HO-1, Hsp70 and autophagy in the solanesol-induced cytoprotective role under stress conditions.
Collectively, the present study demonstrated that solanesol restrained LPS-mediated inflammation depending on HO-1 through activation of p38 and Akt, and promotion of autophagy. Our findings suggest the therapeutic potential of solanesol in the prevention or treatment of inflammatory diseases.
Acknowledgements
This work was supported by the National Nature Science Foundation of China (no. 31270112), the Major Program of the Natural Science Foundation of the Anhui Higher Education Institutions of China (no. KJ2014A149, KJ2012ZD10) and the Natural Science Foundation of Bengbu University (no. 2011ZR02zd).
References
- A. Paine, B. Eiz-Vesper, R. Blasczyk and S. Immenschuh, Signaling to heme oxygenase-1 and its anti-inflammatory therapeutic potential, Biochem. Pharmacol., 2010, 80, 1895–1903 CrossRef CAS PubMed.
- J. A. Araujo, M. Zhang and F. Yin, Heme oxygenase-1, oxidation, inflammation, and atherosclerosis, Front. Pharmacol., 2012, 3, 1–17 Search PubMed.
- L. D. Orozco, M. H. Kapturczak, B. Barajas, X. Wang, M. M. Weinstein and J. Wong,
et al., Heme oxygenase-1 expression in macrophages plays a beneficial role in atherosclerosis, Circ. Res., 2007, 100, 1703–1711 CrossRef CAS PubMed.
- R. Gozzelino, V. Jeney and M. P. Soares, Mechanisms of cell protection by heme oxygenase-1, Annu. Rev. Pharmacol. Toxicol., 2010, 50, 323–354 CrossRef CAS PubMed.
- A. A. Chora, P. Fontoura, A. Cunha, T. F. Pais, S. Cardoso and P. P. Ho,
et al., Heme oxygenase-1 and carbon monoxide suppress autoimmune neuroinflammation, J. Clin. Invest., 2007, 117, 438–447 CAS.
- R. A. Hegazi, K. N. Rao, A. Mayle, A. R. Sepulveda, L. E. Otterbein and S. E. Plevy, Carbon monoxide ameliorates chronic murine colitis through a heme oxygenase 1-dependent pathway, J. Exp. Med., 2005, 202, 1703–1713 CrossRef CAS PubMed.
- A. R. Kinderlerer, I. Pombo Gregoire, S. S. Hamdulay, F. Ali, R. Steinberg and G. Silva,
et al., Heme oxygenase-1 expression enhances vascular endothelial resistance to complement-mediated injury through induction of decay-accelerating factor: a role for increased bilirubin and ferritin, Blood, 2009, 113, 1598–1607 CrossRef CAS PubMed.
- C. Cai, L. Teng, D. Vu, J. Q. He, Y. Guo and Q. Li,
et al., The Heme Oxygenase 1 Inducer (CoPP) Protects Human Cardiac Stem Cells against Apoptosis through Activation of the Extracellular Signal-regulated Kinase (ERK)/NRF2 Signaling Pathway and Cytokine Release, J. Biol. Chem., 2012, 287, 33720–33732 CrossRef CAS PubMed.
- M. Bonelli, A. Savitskaya, C. W. Steiner, E. Rath, M. Bilban and O. Wagner,
et al., Heme oxygenase-1 end-products carbon monoxide and biliverdin ameliorate murine collagen induced arthritis, Clin. Exp. Rheumatol., 2012, 30, 73–78 CAS.
- S. Bortscher, J. Chang, T. O. Vilz, N. Schafer, N. Sommer and S. Wehner,
et al., Hemin induction of HO-1 protects against LPS-induced septic ileus, J. Surg. Res., 2012, 178, 866–873 CrossRef CAS PubMed.
- S. W. Chung, X. Liu, A. A. Macias, R. M. Baron and M. A. Perrella, Heme oxygenase-1-derived carbon monoxide enhances the host defense response to microbial sepsis in mice, J. Clin. Invest., 2008, 118, 239–247 CAS.
- J. Alam and J. L. Cook, How many transcription factors does it take to turn on the heme oxygenase-1 gene?, Am. J. Respir. Cell Mol. Biol., 2007, 36, 166–174 CrossRef CAS PubMed.
- K. P. Kotipalli, N. C. V. Rao and K. Raj, Estimation of solanesol in tobacco and non-tobacco plants from Solanaceae family, J. Med. Aromat. Plant Sci., 2008, 30, 65–68 Search PubMed.
- M. A. Taylor and P. D. Fraser, Solanesol: Added value from Solanaceous waste, Phytochemistry, 2011, 72, 1323–1327 CrossRef CAS PubMed.
- H. Y. Hsu, L. C. Chu, K. F. Hua and L. K. Chao, Heme oxygenase-1 mediates the anti-inflammatory effect of Curcumin within LPS-stimulated human monocytes, J. Cell Physiol., 2008, 215, 603–612 CrossRef CAS PubMed.
- Q. Ma, Role of nrf2 in oxidative stress and toxicity, Annu. Rev. Pharmacol. Toxicol., 2013, 53, 401–426 CrossRef CAS PubMed.
- P. Waltz, E. H. Carchman, A. C. Young, J. Rao, M. R. Rosengart and D. Kaczorowski,
et al., Lipopolysaccaride induces autophagic signaling in macrophages via a TLR4, heme oxygenase-1 dependent pathway, Autophagy, 2011, 7, 315–320 CrossRef CAS PubMed.
- M. Kobayashi and M. Yamamoto, Molecular mechanisms activating
the Nrf2-Keap1 pathway of antioxidant gene regulation, Antioxid. Redox Signaling, 2005, 7, 385–394 CrossRef CAS PubMed.
- T. Nguyen, P. J. Sherratt, H. C. Huang, C. S. Yang and C. B. Pickett, Increased protein stability as a mechanism that enhances Nrf2-mediated transcriptional activation of the antioxidant response element, Degradation of Nrf2 by the 26 S proteasome, J. Biol. Chem., 2003, 278, 4536–4541 CrossRef CAS PubMed.
- J. Pi, Y. Bai, J. M. Reece, J. Williams, D. Liu and M. L. Freeman,
et al., Molecular mechanism of human Nrf2 activation and degradation: role of sequential phosphorylation by protein kinase CK2, Free Radicals Biol. Med., 2007, 42, 1797–1806 CrossRef CAS PubMed.
- A. I. Rojo, O. N. Medina-Campos, P. Rada, A. Zuniga-Toala, A. Lopez-Gazcon and S. Espada,
et al., Signaling pathways activated by the phytochemical nordihydroguaiaretic acid contribute to a Keap1-independent regulation of Nrf2 stability: Role of glycogen synthase kinase-3, Free Radicals Biol. Med., 2012, 52, 473–487 CrossRef CAS PubMed.
- M. Pascual-Garcia, L. Rue, T. Leon, J. Julve, J. M. Carbo and J. Matalonga,
et al., Reciprocal negative cross-talk between liver X receptors (LXRs) and STAT1: effects on IFN-gamma-induced inflammatory responses and LXR-dependent gene expression, J. Immunol., 2013, 190, 6520–6532 CrossRef CAS PubMed.
- S. B. Joseph, M. N. Bradley, A. Castrillo, K. W. Bruhn, P. A. Mak and L. Pei,
et al., LXR-dependent gene expression is important for macrophage survival and the innate immune response, Cell, 2004, 119, 299–309 CrossRef CAS PubMed.
- C. Jiang, A. T. Ting and B. Seed, PPAR-gamma agonists inhibit production of monocyte inflammatory cytokines, Nature, 1998, 391, 82–86 CrossRef CAS PubMed.
- J. S. Welch, M. Ricote, T. E. Akiyama, F. J. Gonzalez and C. K. Glass, PPARγ and PPARδ negatively regulate specific subsets of lipopolysaccharide and IFN-γ target genes in macrophages, Proc. Natl. Acad. Sci. U. S. A., 2003, 100, 6712–6717 CrossRef CAS PubMed.
- S. Ogawa, J. Lozach, C. Benner, G. Pascual, R. K. Tangirala and S. Westin,
et al., Molecular Determinants of Crosstalk between Nuclear Receptors and Toll-like Receptors, Cell, 2005, 122, 707–721 CrossRef CAS PubMed.
- J. M. Yuk, D. M. Shin, H. M. Lee, J. J. Kim, S. W. Kim and H. S. Jin,
et al., The orphan nuclear receptor SHP acts as a negative regulator in inflammatory signaling triggered by Toll-like receptors, Nat. Immunol., 2011, 12, 742–751 CrossRef CAS PubMed.
- B. Levine, N. Mizushima and H. W. Virgin, Autophagy in immunity and inflammation, Nature, 2011, 469, 323–335 CrossRef CAS PubMed.
- L. Fesus, M. A. Demeny and G. Petrovski, Autophagy shapes inflammation, Antioxid. Redox Signaling, 2011, 14, 2233–2243 CrossRef PubMed.
- M. Komatsu, H. Kurokawa, S. Waguri, K. Taguchi, A. Kobayashi and Y. Ichimura,
et al., The selective autophagy substrate p62 activates the stress responsive transcription factor Nrf2 through inactivation of Keap1, Nat. Cell Biol., 2010, 12, 213–223 CAS.
- T. M. Stepkowski and M. K. Kruszewski, Molecular cross-talk between the NRF2/KEAP1 signaling pathway, autophagy, and apoptosis, Free Radicals Biol. Med., 2011, 50, 1186–1195 CrossRef CAS PubMed.
- K. Fujita and S. M. Srinivasula, TLR4-mediated autophagy in macrophages is a p62-dependent type of selective autophagy of aggresome-like induced structures (ALIS), Autophagy, 2011, 7, 552–554 CrossRef CAS PubMed.
- B. E. Riley, S. E. Kaiser and R. R. Kopito, Autophagy inhibition engages Nrf2-p62 Ub-associated signaling, Autophagy, 2011, 7, 338–340 CrossRef PubMed.
- K.-i. Fujita, D. Maeda, Q. Xiao and S. M. Srinivasula, Nrf2-mediated induction of p62 controls Toll-like receptor-4–driven aggresome-like induced structure formation and autophagic degradation, Proc. Natl. Acad. Sci. U. S. A., 2011, 108, 1427–1432 CrossRef CAS PubMed.
- J. G. Bode, C. Ehlting and D. Haussinger, The macrophage response towards LPS and its control through the p38(MAPK)-STAT3 axis, Cell Signal, 2012, 24, 1185–1194 CrossRef CAS PubMed.
- R. T. Netea-Maier, T. S. Plantinga, F. L. van de Veerdonk, J. W. Smit and M. G. Netea, Modulation of inflammation by autophagy: Consequences for human disease, Autophagy, 2016, 12, 245–260 CrossRef CAS PubMed.
- V. Deretic, T. Saitoh and S. Akira, Autophagy in infection, inflammation and immunity, Nat. Rev. Immunol., 2013, 13, 722–737 CrossRef CAS PubMed.
- A. Choy and C. R. Roy, Autophagy and bacterial infection: an evolving arms race, Trends Microbiol., 2013, 21, 451–456 CrossRef CAS PubMed.
- X. Yao, Q. Bai, D. Yan, G. Li, C. Lü and H. Xu, Solanesol protects human hepatic L02 cells from ethanol-induced oxidative injury via upregulation of HO-1 and Hsp70, Toxicol. in Vitro, 2015, 29, 600–608 CrossRef CAS PubMed.
- E. H. Carchman, J. Rao, P. A. Loughran, M. R. Rosengart and B. S. Zuckerbraun, Heme oxygenase-1-mediated autophagy protects against hepatocyte cell death and hepatic injury from infection/sepsis in mice, Hepatology, 2011, 53, 2053–2062 CrossRef CAS PubMed.
- A. Liu, L. Huang, E. Guo, R. Li, J. Yang and A. Li,
et al., Baicalein pretreatment reduces liver ischemia/reperfusion injury via induction of autophagy in rats, Sci. Rep., 2016, 6, 25042 CrossRef CAS PubMed.
- K. Dokladny, M. N. Zuhl, M. Mandell, D. Bhattacharya, S. Schneider and V. Deretic,
et al., Regulatory coordination between two major intracellular homeostatic systems: heat shock response and autophagy, J. Biol. Chem., 2013, 288, 14959–14972 CrossRef CAS PubMed.
- K. Dokladny, O. B. Myers and P. L. Moseley, Heat shock response and autophagy–cooperation and control, Autophagy, 2015, 11, 200–213 CrossRef PubMed.
|
This journal is © The Royal Society of Chemistry 2017 |
Click here to see how this site uses Cookies. View our privacy policy here.