DOI:
10.1039/C6FO00866F
(Paper)
Food Funct., 2017,
8, 220-231
Arabinoxylan hydrolyzates as immunomodulators in Caco-2 and HT-29 colon cancer cell lines
Received
10th June 2016
, Accepted 22nd November 2016
First published on 29th November 2016
Abstract
The use of plant derived polysaccharides as health promoters has gained immense interest in the past few years. Arabinoxylan (AX) is the predominant non-starch polysaccharide in cereals and grasses including wheat. The current research aimed to investigate the structure–function relationship of arabinoxylan hydrolyzates (AXH), obtained by the enzymatic hydrolysis of AX using xylanase and arabinofuranosidase as immunomodulators in two colon cancer cell lines: Caco-2 and HT-29. Fine structural details had a strong correlation with the immunological properties of the wheat AXH. As a general trend, as the presence of arabinose substitution increased in the AXH, the production of proinflammatory cytokines, IL-8 and TNF-α, decreased in both cell lines. Thus, AXH with a higher degree of arabinose substitution might be better adept in lowering inflammation in colon cancer cells.
Introduction
The immune system consists of molecules, cells, tissues and organs that function together to provide immunity to the body. It consists of innate immunity which provides the initial protection against infections and adaptive immunity which develops later in response to infections. Innate immunity is achieved through the components of the innate immune system which consists of epithelial barriers, phagocytic cells, natural killer cells, the complement system and cytokines.1 Thus, intestinal epithelial cells play an important role in the immune system as the single layer of cells that acts as the barrier between the luminal content and the host.2 As part of the innate immune system, intestinal epithelial cells exhibit the ability to recognize antigens through pattern recognition receptors such as Toll-like receptors (TLRs), produce cytokines and chemokines, and act as nonprofessional antigen-presenting cells.3 As they border the antigen-rich lumen and a lymphocyte-rich lamina propria, the intestinal epithelial cells contribute to maintain the mucosal immunoregulation in the gut.3 Thus, intestinal inflammation is a natural and protective function of the gastro-intestinal tract which helps maintain the health of the individual.4 However, deregulation of this inflammatory response can lead to detrimental effects. Inflammatory bowel disease (IBD), the collective name for Crohn's disease and ulcerative colitis, is one such outcome.5 The disease is characterized by strong and unpredictable inflammatory attacks in the intestine. It affects as many as 1.4 million persons in the United States and about 2.2 million persons in Europe.6 Patients with IBD have an increased risk of developing colitis-associated colorectal cancer due to the increased tumorigenesis resulting from chronic inflammation.7 Thus, patients with inflammatory bowel disease (IBD) have a greater risk of developing colon cancer than healthy patients.8–10 Dietary components that exhibit immunomodulatory properties could be beneficial in regulating the intestinal inflammation that leads to diseases such as IBD.
Arabinoxylan (AX) is the predominant polysaccharide in the cell wall of wheat grain.11 AX consists of a backbone of β-(1,4)-linked xylose residues, which is substituted with arabinose residues on the C(O)-2 and/or C(O)-3 position.12 Phenolic acids such as ferulic acid can be ester linked on the C(O)-5 position of arabinose. The immunomodulatory properties of AX have also been reported. Using the phagocytic activity of macrophage-like cells as an indicator of immune function activation, immunostimulating activities of wheat bran derived AX were found to be higher than the immunostimulating activities of AX derived from corn husk or rice bran.13 Arabinoxylans from wheat bran have also been shown to exert potent stimulating effects on innate and acquired immune responses on oral administration in female BALB/c mice. AX was shown to exert an effect on macrophage phagocytosis and delayed hypersensitivity reactions.14 Recently, Cao et al.15 investigated the anti-tumor activities of AX in mice and found that AX from wheat bran was effective in inhibiting the growth of transplanted tumors. Also, AX has been shown to have a possible effect on stimulating antibody mediated immune responses in chickens with Eimeria infection.16 However, the exact cellular mechanisms of how these immunomodulatory effects are brought about by the AX are still not well understood. More so, there is a scarcity of research on understanding how the fine chemical structure of these complex polysaccharides affects their immunomodulatory properties. Thus, we aimed to investigate how the fine chemical structure of enzymatically hydrolyzed AXH affects their immunomodulatory properties. In this study, we assessed the immunomodulatory effects of 12 selected AX hydrolyzates produced from wheat bran derived AX on two colon cancer cell lines, Caco-2 and HT-29 cells, following stimulation by lipopolysaccharides (LPS).
Materials and methods
Materials
The human colorectal adenocarcinoma cell line HT-29 was a generous gift from Dr Bin Guo (Department of Pharmaceutical Sciences, North Dakota State University). The human colorectal adenocarcinoma cell line, Caco-2, was purchased from American Type Culture Collection (ATCC) (Manassas, VA). Eagle's Minimum Essential Medium (EMEM) (ATCC® 30-2003™), Dulbecco's Modified Eagle's Medium (DMEM) (ATCC® 30-2002™) and Fetal Bovine Serum (FBS) (ATCC® 30-2020™) were from ATCC (Manassas, VA). Penicillin–streptomycin solution (10
000 units per mL penicillin/10
000 μg mL−1 streptomycin) was purchased from HyClone Laboratories, Inc. (Logan, UT). Trypsin (1×, 0.25% trypsin in HBSS without calcium and magnesium) was from Mediatech Inc. (Manassas, VA). Lipopolysaccharide (LPS) (Escherichia coli 0111:B4, L4391-1MG, lot no: 043M4089V) was from Sigma-Aldrich (St Louis, MO). The human IL-8 ELISA kit (KHC0081) was purchased from Invitrogen Corporation (Camarillo, CA) The detection limit of human IL-8 ELISA was <5.0 pg mL−1. The human IL-1β Quantikine ELISA kit (DLB50) (minimum detection limit of <1 pg mL−1), human IL-6 Quantikine ELISA kit (D6050) (minimum detection limit of <0.7 pg mL−1) and human TNF-α Quantikine ELISA kit (DTA00C) (minimum detection limit of 0.5–5.5 pg mL−1) were purchased from R&D Systems, Inc. (Minneapolis, MN). PARIS™ kit: the protein and RNA isolation system (AM1921) was purchased from Ambion® by Life Technologies (Carlsbad, CA). The reverse transcription system (A3500) used for the production of cDNA was from Promega Corporation (Madison, WI). The Brilliant II SYBR® Green QRT-PCR Master Mix with Low ROX kit (600835) was purchased from Agilent Technologies, USA.
Production and characterization of arabinoxylan hydrolyzates
Wheat AX (WAX) was extracted from bran using methods described by Mendis and Simsek.17 Briefly, wheat bran was defatted, destarched and deprotinized and extracted with alkaline hydrogen peroxide and separated out using ethanol precipitation. This procedure was repeated once again to further purify the sample and the resulting material was termed wheat arabinoxylan (WAX) which was used in the preparation of different arabinoxylan hydrolyzates (AXH).
Wheat arabinoxylan hydrolyzates were prepared according to the method described by Mendis and Simsek.17 Briefly, two xylanases (endo-1,4-β-xylanase (Cellvibrio japonicus) (CJX) and endo-1,4-β-xylanase M4 (Aspergillus niger) (ANX)) and two arabinofuranosidases (α-L-arabinofuranosidase (Clostridium thermocellum) (CAF) and α-L-arabinofuranosidase (novel specificity) (Bifidobacterium adolescentis) (BAF)) were used. Among the two arabinofuranosidases used, BAF hydrolyzes the C3-linked arabinose residues from double-substituted xylose residues18 while CAF hydrolyzes the C3-linked arabinosyl side chain of xylan.19 Thus, these enzymes were used in different combinations to produce twelve different arabinoxylan hydrolyzates with different structural details.
The determination of the arabinose substitution pattern and linkage analysis of arabinoxylan hydrolyzates were carried out according to the methods described by Mendis and Simsek17 using 1H-NMR and GC-MS techniques.
Determination of endotoxin contamination in AXH
Using the Pierce LAL Chromogenic Endotoxin Quantitation kit (Thermo Scientific, Rockford, IL) the amount of endotoxin present in the AXH samples was determined following the manufacturer's instructions.
Determination of the immunomodulatory properties of AXH
Enzymatically derived arabinoxylan hydrolyzates were evaluated for their immunomodulatory properties using LPS induced intestinal epithelial cell lines Caco-2 and HT-29. Caco-2 cells were grown in EMEM supplemented with 20% FBS and 1% penicillin–streptomycin solution. HT-29 cells were grown in DMEM media supplemented with 10% FBS and 1% penicillin–streptomycin solution. The cells were grown at 37 °C under a humidified atmosphere of 95% air and 5% CO2. The cells were cultured in the appropriate media until they reached 80–90% confluence before using in the assays. The number of viable cells was determined by counting manually on a haemocytometer after staining with 0.4% (w/v) trypan blue (Mediatech, Inc., Manassas, VA). The cells were plated and treated with AXH according to the methods described by Li et al.,20 with some modifications as described below.
Treatment of cells with AXH
Caco-2 cells were plated at 2 × 106 cells per mL cell density (400 μL) in 24 well plate wells and incubated overnight. Next day, AXH (2000 μg mL−1) dissolved in serum free-antibiotic free EMEM was added (200 μL) to each well. The negative control wells and control wells both received serum free-antibiotic free EMEM (200 μL). After 2 h incubation with each treatment, 200 μL of LPS dissolved in serum free-antibiotic free EMEM (4 μg mL−1) was added to each well to induce inflammation. 200 μL of serum free-antibiotic free EMEM was added to negative control wells. Thus, the final concentration of each compound in the wells was: AXH (500 μg mL−1) and LPS (1 μg mL−1). The choice of 500 μg mL−1 of the AXH concentration was based on previous research which employed similar concentrations of AX extracts in cell culture model systems.20–22 The cells were incubated for 24 h. The same procedure was carried out using HT-29 cells with the following modifications. The cells were plated at 1 × 106 cells per mL cell density and the medium used was DMEM. The EMEM used for dissolving the compounds were replaced with DMEM. Thus, negative control treatment consisted of no LPS (−LPS) and control treatment consisted of LPS (+LPS). AXH treated cells were identified as the corresponding AXH + LPS.
Determination of IL-8 and TNF-α by ELISA
The extracellular media of the cells treated under the conditions described above were collected and centrifuged at 13
000 rpm for 3 min to precipitate the cell debris. The supernatants were aliquoted out and stored at −20 °C until further analysis. The amount of each cytokine in the media was determined using the appropriate ELISA kit following the manufacturer's instructions. The results were quantified using the corresponding standard provided with each kit.
Quantification of human TLR4 and COX-2 mRNAs using quantitative reverse transcriptase real-time polymerase chain reaction (qRT-PCR)
The treated cells were detached using a cell scraper and washed with PBS according to the manufacturer's instructions for the total RNA isolation using the PARIS™ kit: the protein and RNA isolation system (Ambion® by Life Technologies, Carlsbad, CA). The reverse transcription system (A3500) (Promega Corporation, Madison, WI) was used for the production of cDNAs. The cDNA samples were stored at −20 °C until use. The qRT-PCR mixture system was set up as follows according to the manufacturer's instructions with some modifications: 10 μL of 2× Brilliant II SYBR® Green QRT-PCR Master Mix with Low ROX (Agilent Technologies, USA), 2 μL of the primer mix (5 μM of forward primer and 5 μM of reverse primer), 1 μL of cDNA (5 ng μL−1) and 7 μL of nuclease free PCR grade water. The primers purchased from The Midland Certified Reagent Company, Incorporated (Midland, TX) were as follows: actin (forward) CATGTACGTTGCTATCCAGGC (reverse) CTCCTTAATGTCACGCACGAT; COX-2 (forward) CAGCAAATCCTTGCTGTTCC (reverse) GTGCACTGTGTTTGGAGTGG; TLR4 (forward) ATATTGACAGGAAACCCCATCCA (reverse) TAGAACCCGCAAGTCTGTGC. The reaction protocol was as follows: 95 °C for 10 min; 40 cycles consisting of 95 °C for 30 s, 60 °C for 1 min, and 72 °C for 1 min; a melting step consisting of 95 °C for 1 min; 55 °C for 30 s; 95 °C for 30 s was added at the end of the cycles. ΔCt values were calculated using actin as the housekeeping gene. Δ(ΔCt) was calculated as ΔCt (AXH + LPS) − ΔCt (cells + LPS). The fold of change was calculated as 2Δ(ΔCt).
Statistical analysis
All the cell culture experiments were performed in duplicate. All statistical analyses were performed using the Statistical Analysis System software package version 9.4 (SAS Institute, Cary, NC).
The data are presented as the mean ± standard deviation (SD) values that were calculated from replications for individual AX treatments. The data were subjected to one way ANOVA and a least significant difference (LSD) was used to declare differences between the treatments. The differences were considered significant when the probability value p was lower than 0.05.
Pearson's correlation analysis was conducted to evaluate relationships between immunological outcomes and AXH composition/structural details.
Results and discussion
Chemical characteristics of the AXH
We obtained 12 AXH polysaccharides by the enzymatic hydrolysis of WAX using xylanase and arabinofuranosidase enzymes. endo-β-(1,4)-D-Xylanases (EC 3.2.1.8, xylanase) hydrolyze the WAX by internally hydrolyzing the 1,4-β-D-xylosidic linkage between the xylose residues in the xylan backbone.12,23 The enzymes α-L-arabinofuranosidases (EC 3.2.1.55) remove arabinose substituents from the xylan backbone.12 Thus, depending on the substrate specificity of the two xylanases and two arabinofuranosidases, AXH with different structural properties with respect to the molecular weight and arabinose substitution pattern were obtained. The AXH used in our study was obtained through alkaline-hydrogen peroxide extraction procedures. Upon reaction with alkaline solutions, ester linked coumaric and ferulic acids can be liberated from AX.24 Arabinoxylan that was extracted using alkaline-hydrogen peroxide extraction procedures has been shown to contain a negligible amount of ferulic acid.14 Since we employed similar extraction procedures to obtain our AXH, the amount of ferulic acid present in our AXH was assumed to be negligible as well. α-D-Glucuronic acid (and its methyl ether, 4-O-methyl-glucuronic acid) is another important substituent of AX11 which could have an impact on the immunomodulatory properties of AX. However, we have not discussed its effect on immunomodulation in the current research.
A detailed discussion on the structural characteristics of the AXH is presented in our previous work.17 In brief, the 1H-NMR results of the different hydrolyzates are summarized in Table 1. Each AXH has its own pattern of resonances corresponding to different anomeric protons in the arabinoxylan molecule. Resonances 1–3 correspond to the anomeric protons of arabinose, while resonances 4–6 correspond to the anomeric proton in xylose. Resonance 6 corresponds to the anomeric proton of unsubstituted xylose. All the hydrolyzates had a high proportion of resonance integration for resonance 6 (37.6–46.6%) indicating that the AXH being tested had a high amount of unsubstituted xylose in the molecule. To further understand the structural details of the AXH, linkage analysis was carried out using GC-MS techniques. As expected, structural heterogeneity was observed with respect to the linkage types present in each arabinoxylan hydrolyzate. This linkage information about each AXH was useful in deducing the effect of structural details on the immunomodulatory properties of the AXH.
Table 1 Amount of different substitutions and linkage types in the arabinoxylan hydrolyzates (AXH)
AXHa |
1H-NMR resonance integrations (% total resonance)b |
GC-MS peak amounts as % of total peak areac |
R1 |
R2 |
R3 |
R4 |
R5 |
R6 |
GCP1 |
GCP2 |
GCP3 |
GCP4 |
GCP5 |
GCP6 |
GCP7 |
Enzymatic treatments were carried out using the following enzymes: ANX, endo-1,4-β-xylanase M4 (Aspergillus niger); CJX, endo-1,4-β-xylanase (Cellvibrio japonicus); BAF, α-L-arabinofuranosidase (novel specificity) (Bifidobacterium adolescentis); CAF, α-L-arabinofuranosidase (Clostridium thermocellum); each sample name starting with the corresponding enzyme abbreviation received that specific enzyme treatment first.
Each resonance corresponds to the following anomeric protons: R1, anomeric proton of O-3 linked arabinose linked to monosubstituted xylose: R2, anomeric proton of O-3 linked arabinose linked to disubstituted xylose: R3, anomeric proton of O-2 linked arabinose linked to disubstituted xylose: R4, anomeric proton of disubstituted xylose: R5, anomeric proton of monosubstituted xylose: R6, anomeric proton of unsubstituted xylose.
GCP1, terminal arabinose: GCP2, terminal xylose: GCP3, 2-substituted 1,4-linked xylose: GCP4, 1,4-linked xylose: GCP5, 1,3-linked arabinose: GCP6, 3-substituted 1,4-linked xylose: GCP7, 2,3-substituted 1,4-linked xylose.
|
ANX-3 |
10.6 |
10.1 |
10.4 |
9.8 |
14.7 |
44.4 |
5.7 |
7.8 |
0.5 |
64.8 |
3.7 |
13.3 |
3.8 |
ANX-4 |
13.2 |
13.6 |
9.4 |
14.1 |
11.7 |
37.8 |
4.5 |
1.3 |
1.1 |
73.3 |
4.4 |
12.2 |
3.2 |
ANX-6 |
14.3 |
13.3 |
10.2 |
11.2 |
13.5 |
37.6 |
13.1 |
3.3 |
0.0 |
52.6 |
4.2 |
10.3 |
10.3 |
ANX-7 |
15.2 |
11.5 |
9.9 |
9.2 |
14.1 |
40.2 |
17.3 |
4.7 |
1.0 |
51.0 |
2.1 |
9.2 |
11.8 |
ANX-8 |
10.0 |
12.7 |
11.1 |
10.9 |
13.5 |
41.7 |
16.4 |
4.8 |
0.7 |
52.0 |
2.2 |
8.5 |
8.9 |
CJX-6 |
12.7 |
12.0 |
11.0 |
6.4 |
13.1 |
44.8 |
13.1 |
2.5 |
0.9 |
50.2 |
4.1 |
8.8 |
8.2 |
CAF-2 |
13.2 |
11.1 |
8.6 |
8.5 |
12.6 |
46.0 |
8.9 |
1.4 |
0.0 |
43.8 |
2.7 |
8.5 |
20.8 |
CAF-3 |
14.1 |
12.3 |
9.6 |
10.7 |
11.6 |
41.7 |
3.6 |
0.7 |
0.0 |
38.0 |
1.4 |
8.5 |
31.1 |
CAF-5 |
14.3 |
10.8 |
10.0 |
9.4 |
11.8 |
43.7 |
11.0 |
1.9 |
0.0 |
52.7 |
3.0 |
8.7 |
11.8 |
CAF-6 |
13.1 |
9.3 |
8.9 |
11.5 |
13.2 |
44.0 |
10.7 |
2.6 |
1.0 |
39.9 |
5.8 |
9.0 |
19.3 |
BAF-5 |
12.6 |
10.6 |
9.2 |
7.5 |
13.5 |
46.6 |
8.6 |
2.5 |
0.0 |
40.2 |
3.2 |
7.2 |
26.6 |
BAF-6 |
14.5 |
11.0 |
10.1 |
8.6 |
13.1 |
42.6 |
4.6 |
1.2 |
0.0 |
0.0 |
2.9 |
8.5 |
17.3 |
Effect of endotoxin contamination of AXH on the immunological activity
The Pierce LAL Chromogenic Endotoxin Quantitation Kit detects as little as 0.1 endotoxin unit per mL (EU per mL), where one EU per mL is equivalent to 0.1 ng endotoxin per mL of solution.25,26 Out of the 12 AXH only four contained detectable levels of endotoxin. The endotoxin levels in these four AXH samples (500 μg mL−1 sample) were 0.016, 0.018, 0.031 and 0.036 ng mL−1 for ANX-3, ANX-8, BAF-6 and ANX-7, respectively. However, there was no apparent relationship between the amount of endotoxin and the biological responses measured for each AXH sample. Several studies have reported 0.5–5000 ng mL−1 endotoxin concentrations to be the lowest concentration of endotoxin to induce significant effects on different cell types.27 In another study wheat germ agglutinin (WGA) contaminated with 1 ng mL−1 LPS was tested on CD33+ cells to appreciate the effect of endotoxin on the observed biological effects.28 They concluded that endotoxin contamination at 1 ng mL−1 did not significantly influence the effect rendered by WGA. The low levels of endotoxins detected in our AXH samples support our suggested conclusion that the immunomodulatory effects observed in our research can be attributed to the effect of the AXH molecules rather than the trace amounts of endotoxins present in the samples.
Immunological activity of AXH
We chose here to investigate the effect of AX hydrolyzates on LPS-stimulated colon cancer cells. In particular we determined how AXH modulated the levels of IL-8, TNF-α and TLR4 transcripts and COX-2 transcripts produced by the LPS stimulated Caco-2 and HT-29 cancer cells. The Caco-2 cell line has been previously used as a model system to study the immunomodulatory activity of AX.20,29 Both Caco-2 and HT-29 cell lines have also been used to study the immunomodulatory properties of dietary fiber previously too.29,30 Thus, we also employed these two cell lines in our experiments to investigate the immunomodulatory activity of AXH.
In order to test the immunomodulatory properties of these AXH, we stimulated the cells with LPS. To verify that there was no significant LPS contamination in the AXH, the AXH was tested on RAW264.7 cells for the induction of NO production, an indicator of inflammation. The AXH did not significantly increase the NO production in RAW264.7 cells (data not shown) indicating that the AXH used in this research was free of significant levels of LPS contamination.
Due to the general structural similarity between AX and LPS,20 the cellular pathways that are triggered by LPS could also be affected by AX. Thus, we used LPS-stimulated cancer cell lines to explore the effect of AXH as immunomodulators. Although LPS-stimulated monocytes and macrophages are most frequently used to evaluate the anti-inflammatory properties of drugs and dietary agents,31,32 LPS-stimulated cancer cells have also been used to study inflammation in these cells in order to study the role of inflammation in cancer.33–35 Thus, we employed a similar model in our research.
Effect of AXH on IL-8 production
IL-8 is an important cytokine involved in the initiation and amplification of inflammatory responses resulting in tissue damage and injury36 and is a primary target for the treatment of intestinal inflammation.37 Elevated mucosal IL-8 levels have been observed in patients with acute ulcerative colitis.38 IL-8 has also been shown to play important roles in cancer progression.39,40 Tumor associated IL-8 is postulated to play a role in leukocyte recruitment into tumors, modulation of angiogenesis and tumor immune response, mediation of tumor growth and motion of tumor cells.40 In colorectal cancer cells, IL-8 increases cell migration through the ERK/Ets-1 pathway.41
We investigated here the effect of each AXH on LPS induced IL-8 production on Caco-2 and HT-29 cells. The levels of IL-8 were 10–20 fold higher in the HT-29 cells than in the Caco-2 cells (Fig. 1). At the present seeding density, the Caco-2 cells produced a small amount of IL-8 compared to the HT-29 cells. Similar observations regarding the differences in IL-8 production between these two cell lines have also been reported previously.29,30 Eight of the 12 AXH (ANX-6, ANX-7, ANX-8, CJX-6, CAF-3, CAF-5, CAF-6 and BAF-5) caused a significant decrease in LPS induced IL-8 production in Caco-2 cells compared to the +LPS treatment. However, in the case of HT-29, none of the AXH significantly lowered the LPS induced IL-8 production. On the contrary, three of the AXH (ANX-3, ANX-8 and CAF-3) significantly increased LPS induced IL-8 production in HT-29 cells. ANX-8 caused the highest increase in IL-8 followed by CAF-3 and ANX-3.
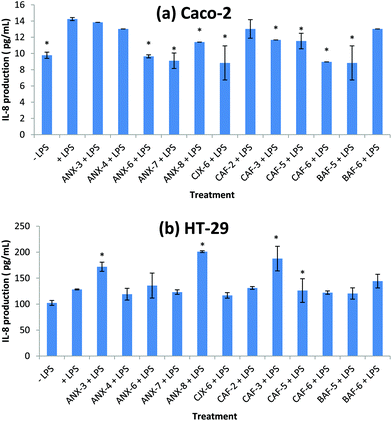 |
| Fig. 1 Immunomodulatory effect of AXH on LPS induced IL-8 production in (a) Caco-2 cells and (b) HT-29 cells. − LPS, cells not induced with LPS; +LPS, cells with LPS; ANX-3 + LPS to BAF-6 + LPS, cells treated with the corresponding AXH and induced with LPS. Data are presented as mean ± SD. Differences with +LPS are marked with an asterisk (*) (p < 0.05). | |
Previous study has shown a barley fiber fraction consisting of 96.4% AX to have no significant effect on the IL-8 production in Caco-2 and HT-29 cells.29 However, they reported these observations based on Caco-2 and HT-29 cells in which there was no induction of inflammation using inducers such as LPS. In our research, being subjected to LPS induced inflammation, some of the AXH were able to significantly decrease (ANX-6, ANX-7, ANX-8, CJX-6, CAF-3, CAF-5, CAF-6 and BAF-5 in Caco-2 cells) or increase (ANX-3, ANX-8, CAF-3 in HT-29 cells) IL-8 production compared to the cells induced with LPS (+LPS). These results suggest that depending on their structural properties AXH could either increase or decrease the production of pro-inflammatory cytokines in LPS induced intestinal epithelial cells. Also, the same AXH that exhibited anti-inflammatory properties in one cell line could exert anti-inflammatory effects on another cell line.
Effect of AXH on TNF-α production
TNF-α is a pro-inflammatory cytokine produced during acute inflammation leading to a wide range of intracellular signaling events.42 It is involved in inducing anti-microbial effects in response to bacterial infection in cells.43–45 It also leads to the production of other related chemokines and cytokines that directs the progression of disease.43 In Caco-2, CAF-5, BAF-5 and ANX-8 were able to reduce TNF-α production significantly compared to the control (+LPS) (Fig. 2). The rest of the AXH did not exert a significant effect on the TNF-α production in Caco-2. In the case of HT-29, there was a significant increase in TNF-α production in cells treated with CJX-6, ANX-4 and CAF-3 compared to the control (+LPS).
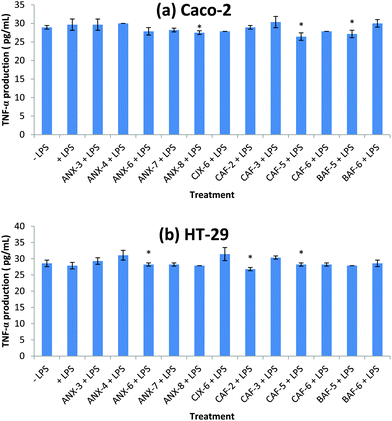 |
| Fig. 2 Immunomodulatory effect of AXH on LPS induced TNF-α production in (a) Caco-2 cells and (b) HT-29 cells. −LPS, cells not induced with LPS; +LPS, cells with LPS; ANX-3 + LPS to BAF-6 + LPS, cells treated with the corresponding AXH and induced with LPS. Data are presented as mean ± SD. Differences with +LPS are marked with an asterisk (*) (p < 0.05). | |
Structure–function relationship of arabinoxylan hydrolyzates
Previous research studies have conducted correlation analysis between the chemical characteristics and biological properties of plant components. A Pearson's correlation analysis was conducted by Sato et al.46 to calculate the correlation between the chemical characteristics (HPLC chromatogram peaks) and antiallergic activity of different onion samples. We employed a similar approach. Pearson's correlation analysis was conducted to evaluate the relationships between immunological outcomes and AXH composition/structural details in our research.
To understand the structure–function relationship among the AXH structure and its immunological properties, correlation was performed among the composition/structural properties of AXH with their effect on IL-8 production and TNF-α production in Caco-2 and HT-29 cells (Table 2).
Table 2 Correlation coefficient between the structural properties and the immunomodulatory properties of AXH in Caco-2 and HT-29 cells
Structural component |
Chemical structure |
Correlation coefficient |
Structural data obtained through 1H-NMR techniques.
Structural data obtained through GC-MS techniques. **P-Value <0.01. *P-Value <0.05. |
IL-8 production in Caco-2 cells
|
Terminal arabinoseb× 1,3-linked arabinoseb |
|
−0.720** |
Amount of terminal arabinoseb× amount of disubstituted xyloseb |
|
−0.690* |
Amount of terminal xyloseb× amount of disubstituted xyloseb |
|
−0.639* |
IL-8 production in HT-29 cells
|
Amount of arabinose substituted to monosubstituted xylose at the O-3 positiona |
|
−0.700* |
TNF-α production in Caco-2 cells
|
Amount of terminal arabinoseb× unsubstituted xylosea |
|
−0.763** |
Amount of terminal arabinoseb× amount of disubstituted xyloseb |
|
−0.606* |
TNF-α production in HT-29 cells
|
Amount of terminal arabinoseb× amount of disubstituted xyloseb |
|
−0.651* |
There was no significant correlation between the IL-8 production in the Caco-2 cells and each single structural characteristic of the AXH identified in Table 1, when these characteristics were considered individually. However, when these structural characteristics were considered in combination (taking two structural characteristics together), in the Caco-2 cells, a significant negative correlation (−0.720) (p < 0.01) between IL-8 and the product of the amount of terminal arabinose and the amount of 1,3-linked arabinose (terminal arabinose × 1,3-linked arabinose) was observed. Also, a significant negative correlation (−0.690) (p < 0.05) between IL-8 and the terminal arabinose × disubstituted xylose was observed in the Caco-2 cells. In HT-29 cells, there was a significant negative correlation (−0.700) between IL-8 and NMR resonance 1 (i.e. arabinose substituted to monosubstituted xylose at the O-3 position) (p < 0.05). As a general trend, the increasing amount of arabinose substitution in the AXH was negatively correlated with the production of IL-8. This suggests that as the arabinose substitution in AXH increases, such AXH might be better adept in lowering the production of proinflammatory cytokines such as IL-8.
Furthermore, TNF-α production was negatively correlated with the product of NMR resonance 6 (i.e. anomeric proton of unsubstituted xylose) × terminal arabinose (−0.763) (p < 0.01) in the Caco-2 cells. These results might suggest that as the structural complexity of the AXH increases, their potential to lower the production of pro-inflammatory cytokines such as TNF-α in the colon cancer cell lines increases.
Effect of AXH on TLR4 transcript levels
Based on the general structural similarity between AX and LPS,20 the cellular pathways that are triggered by LPS could also be affected by AX. TLR4 is the receptor involved in the recognition of LPS on the cell surface.47 Thus, we investigated the effect of AXH on the TLR4 receptor. Both Caco-2 and HT-29 cells have been shown to express TLR4 at the transcript and protein levels; however Caco-2 cells have been shown to express lower levels of the key molecular partners (MD2, CD14 and MyD88) involved in TLR4 signaling than HT-29.33–35 Moreover, contradictory data regarding the functional relevance of TLR4 signaling in Caco-2 cells have been reported. Indeed, the study of Hsu et al.35 showed that under their experimental conditions, Caco-2 cells did not express TLR4 at the cell surface, although the protein was found in the cell extract, suggesting that TLR4 could not transmit signals from extracellular ligands, such as LPS, in these cells.35 This observation is supported by studies where Caco-2 cells were found to be unresponsive to LPS stimulation.48–51 However, under certain experimental conditions, Caco-2 cells have been shown to respond to stimulation by LPS.52–57 Based on these conflicting data, we decided to test the effects of our AXH after LPS stimulation in both Caco-2 and HT-29 cells.
We analyzed here how AXH modulated the expression of TLR4 transcripts following stimulation by LPS. We observed significant (P < 0.05) changes in the TLR4 transcript levels in Caco-2 cells treated with ANX-6 with an ∼52 fold increase in TLR4 transcripts compared to the +LPS treatment group (Fig. 3). This was the only treatment group with significant differences versus the +LPS treatment group in Caco-2 cells.
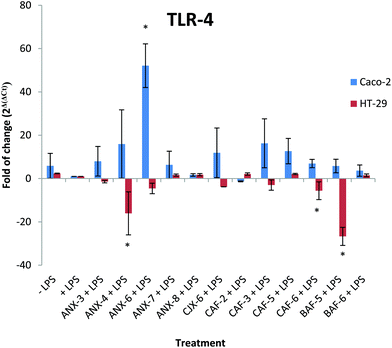 |
| Fig. 3 Immunomodulatory effect of AXH on LPS induced TLR-4 mRNA production in Caco-2 cells and HT-29 cells. −LPS, cells not induced with LPS; +LPS, cells with LPS; ANX-3 + LPS to BAF-6 + LPS, cells treated with the corresponding AXH and induced with LPS. Data are presented as mean ± SD. Differences with +LPS are marked with an asterisk (*) (p < 0.05). | |
However, in HT-29 cells, there was a significant reduction (p < 0.05) in TLR-4 transcripts in treatments that received ANX-4, CAF-6 and BAF-5 (∼16, 5 and 26 fold reduction) compared to the +LPS treatment group (Fig. 3).
Between the two cell lines, the AXH did not yield similar outcomes with respect to their effect on the TLR-4 transcript levels. This suggests that the effect of these AXH on TLR-4 transcripts might be cell type dependent.
Changes in the TLR4 transcript levels suggest that the AXH modulates gene expression in the cancer cell lines. One hypothesis is that AHX could stimulate either positive or negative feedback loops resulting in up-regulation or suppression of TLR4. Although we have not shown whether the changes in TLR4 transcripts are associated with changes in TLR4 at the protein levels, our data suggest that AXH could modulate TLR4 signaling by increasing the amount of TLR4 available for signaling.
TLR4 is a pattern recognition receptor that is involved in the recognition of bacterial membrane components such as LPS.43 In monocytes, TLR4 along with its intracellular adaptor molecule MyD88 leads to the activation of the MAPK pathways,58 specifically p38 and ultimately resulting in the activation of NF-κB.43,59,60 NF-κB is an important transcription factor involved in various immunological processes including cytokine production, phagocytosis and respiratory burst.61 Eventually NF-κB triggers the expression of inflammatory cytokines, such as TNF-α.43,62 Several studies have demonstrated the involvement of NF-κB in cellular pathways triggered by botanical polysaccharides.61,63,64 Wang et al.42 demonstrated that sulfated Astragalus polysaccharide (SAPS) lowered the expression of LPS induced TLR4 mRNA transcripts compared to the control of only LPS induced cells in Caco-2 cells. Thus, SAPS has been indicated as a potential anti-inflammatory agent. On the other hand, sulfated polysaccharides from marine red algae have been shown to possess pro-inflammatory properties.65 Our data indicate that different AXH can behave differently either as pro- or anti-inflammatory compounds, with respect to their effect on TLR4 transcripts, depending on the structure of the AXH and the cell type being tested on.
Besides the important role of TLR4 in inflammation, TLR4 signaling has also been shown to contribute significantly to cancer progression with both pro- and anti-cancer effects.66–68 AXH could thus also modulate colon cancer progression through the regulation of TLR4.
A recent study has demonstrated that AX interacts with dectin-1 receptors and not TLR4.69 Our findings on TLR4 expression upon AXH do not provide adequate evidence to relate to this claim. However, if AXH does not interact with TLR4, the observed regulation of TLR4 transcripts in the two colon cancer cell lines could be linked to dectin-1 receptor signaling.
Effect of AXH on COX-2 transcript levels
In phagocytic cells, the engagement of TLR4 by LPS leads to the downstream activation of MAP kinases, and of the transcription factor NF-κB, controlling the expression of pro-inflammatory cytokines (IL-1, IL-6 and TNF-α), the expression of inducible NO synthase (iNOS) and of COX. Thus, to further understand the cellular responses involved in the immunomodulatory properties of AXH in LPS induced intestinal cells, the expression of COX-2 at the mRNA level was investigated using qRT-PCR.
Two isoforms of enzyme COX: COX-1 and COX-2 are responsible for prostaglandin synthesis.70 While high levels of COX-1 are expressed under physiological conditions, COX-2 remains at low levels.71 On the contrary, COX-2 is considered an inducible form of COX and elevated levels are observed during inflammation.72 The up-regulation of COX-2 induces the production of PG-E2 which is associated with pathological conditions such as inflammatory bowel disease71 and slow transit constipation.73 In the presence of endotoxins such as LPS, mitogen-activated protein kinase (MAPK) (specifically p38 MAPK) is involved in the regulation of COX-2 mRNA transcription.74,75 Also, the anti-inflammatory properties of many phytochemicals have been predicted to be achieved by the down regulation of COX-2 (via suppression of NF-κB activation).76
The effect of different AXH on LPS induced COX-2 gene expression was evaluated by assessing the COX-2 mRNA levels in Caco-2 and HT-29 cells (Fig. 4). In Caco-2 cells, seven treatment groups showed significant increases in COX-2 transcripts compared to the control (+LPS). Treatment with ANX-6 resulted in the largest increase (∼30 fold increase). Treatment with ANX-4, CJX-6, CAF-3, CAF-5, CAF-6 and BAF-5 resulted in fold increases ranging from 31 to 9.5. However, the AXH exerted noticeably different effects on the HT-29 cells with respect to the COX-2 mRNA transcript levels compared to those on the Caco-2 cells. Treatment with ANX-4, CAF-6 and BAF-5 resulted in significantly reduced levels (∼13, 5 and 22 fold respectively) compared to the +LPS treatment. Interestingly, while most of the AXH lead to the increased levels of COX-2 mRNA transcripts in Caco-2, the trend was reversed in HT-29 cells. This might indicate that the effect of each AXH is highly cell specific.
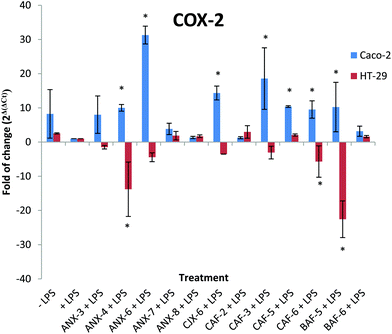 |
| Fig. 4 Immunomodulatory effect of AXH on LPS induced COX-2 mRNA production in Caco-2 cells and HT-29 cells. −LPS, cells not induced with LPS; +LPS, cells with LPS; ANX-3 + LPS to BAF-6 + LPS, cells treated with the corresponding AXH and induced with LPS. Data are presented as mean ± SD. Differences with +LPS are marked with an asterisk (*) (p < 0.05). | |
Relationship between TLR4 transcript and COX-2 transcript levels in the presence of AXH
Similar to the analysis by Sato et al.,46 we also conducted Pearson's correlation analysis to evaluate the relationships between the TLR4 transcripts and COX-2 transcripts in each cell line.
We observed an interesting connection between the TLR4 transcript and COX-2 transcript levels in the presence of each AXH. The expression pattern of the TLR-4 transcript levels and COX-2 transcript levels in each cell line in the presence of each AXH was almost superimposable (Fig. 3 and 4). There was a significant positive correlation (0.931) between the TLR4 transcripts and COX-2 transcripts in Caco-2 cells (p < 0.001). Also, there was a significant positive correlation (0.997) between the TLR4 transcripts and COX-2 transcripts in HT-29 cells (p < 0.001). This indicates that in Caco-2 and HT-29 cells the transcriptions of TLR4 and COX-2 are closely related at the transcriptional level. Previous research has shown that intestinal epithelial cell lines up-regulate COX-2 expression in a TLR4- and MyD88-dependent fashion.77 Similarly, we observed a close correlation between TLR4 expression and COX-2. An overview of the TLR4 triggered signaling pathways leading to the production of COX-2 and other inflammatory cytokines is shown in Fig. 5.47,74,75,78
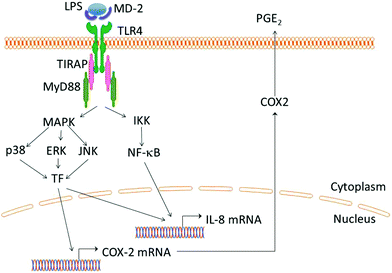 |
| Fig. 5 LPS are recognized on the cell surface by the TLR-4/MD-2 complex (Takeuchi & Akira, 2010).47 Ligand stimulation recruits TIRAP and MyD88 to the TLR leading to the activation of IKK complex. Subsequently, NF-κB is activated and translocated to the nucleus, driving the expression of cytokine genes such as IL-8. Simultaneously, MAPK cascades are activated leading to the activation of transcription factors such as AP-1 which are important in the induction of cytokine genes (Takeuchi & Akira, 2010)47 (Kawasaki T, 2013).78 In the presence of endotoxins such as LPS, mitogen-activated protein kinase (MAPK) (specifically p38 MAPK) is involved in the regulation of COX-2 mRNA transcription (Grishin et al., 2004; Grishin et al., 2006).74,75 | |
Conclusions
The use of plant derived polysaccharides as health promoters has gained immense interest in the past few years. The current research aimed to investigate the structure–function relationship of enzymatically tailored AXH as immunomodulators using LPS induced colon cancer cell lines: Caco-2 and HT-29. Twelve structurally different AXH were prepared from wheat AX, using different enzymatic conditions. The 12 different AXH being tested showed different immunological properties with respect to the cell type and the production of IL-8 and TNF-α.
The importance of polymer side branching in the immunological properties of polymers has also been emphasized by others.79,80 Zhou et al.14 also suggested that the immunological effects of wheat bran AX depend on the molecular weight, chemical composition and the substituted degree or branch of arabinose. Similarly, we demonstrated that the structural details had a strong correlation with the immunological properties of the wheat AXH. We observed strong correlations between the production of IL-8 and TNF-α, and the structural features of the AXH. Strong correlations were observed when the structural features were considered in combination rather than individually. The presence of arabinose substitution along the xylan backbone was negatively correlated with the production of pro-inflammatory cytokines, IL-8 and TNF-α in both cell lines. Thus, AXH with a higher degree of arabinose substitution could be better adept in lowering inflammation in colon cancer cells.
The current research indicates that there are structure driven immunological properties for wheat bran derived AX polysaccharides. However, to date, the cellular mechanisms involved in the recognition of AXH and the downstream cellular pathways involved in the process have not been well defined. Our study provides new insights into the mechanisms of AXH in colon cancer cells and strongly suggests that AXH can stimulate COX2 through TLR-4 engagement and up-regulation. Thus, future research exploring the cellular mechanisms pertaining to the recognition of AX by colon cancer cells is encouraged.
Acknowledgements
We thank Kristin Whitney for the help with the SEC-MALS equipment running and data processing and other laboratory equipment use and her valuable input. James Gillespie is kindly appreciated for his time and expertise on the GC-MS equipment running and data processing. We also thank Dr Jae Ohm for his help with the statistical analysis of the data. We acknowledge the North Dakota Wheat Commission and the NDSU Agriculture Experiments Station for funding this research.
Notes and references
-
Basic immunology: functions and disorders of the immune system, ed. A. K. Abbas and A. H. Lichtman, Saunders Elsevier, Philadelphia, PA, USA, 2011 Search PubMed.
- J. J. Deuring, C. de Haar, E. J. Kuipers, M. P. Peppelenbosch and C. J. van der Woude, The cell biology of the intestinal epithelium and its relation to inflammatory bowel disease, Int. J. Biochem. Cell Biol., 2013, 45, 798–806 CrossRef CAS PubMed.
- S. Dahan, F. Roth-Walter, P. Arnaboldi, S. Agarwal and L. Mayer, Epithelia: lymphocyte interactions in the gut, Immunol. Rev., 2007, 215, 243–253 CrossRef CAS PubMed.
- G. R. Martin and J. L. Wallace, Gastrointestinal inflammation: a central component of mucosal defense and repair, Exp. Biol. Med., 2006, 231, 130–137 CAS.
- M. G. Neuman, Immune dysfunction in inflammatory bowel disease, Transl. Res., 2007, 149, 173–186 CrossRef CAS PubMed.
- E. V. Loftus Jr., Clinical epidemiology of inflammatory bowel disease: incidence, prevalence, and environmental influences, Gastroenterology, 2004, 126, 1504–1517 CrossRef.
- M. Saleh and G. Trinchieri, Innate immune mechanisms of colitis and colitis-associated colorectal cancer, Nat. Rev. Immunol., 2011, 11, 9–20 CrossRef CAS PubMed.
- S. H. Itzkowitz and X. Yio, Inflammation and cancer IV. Colorectal cancer in inflammatory bowel disease: the role of inflammation, Am. J. Physiol.: Gastrointest. Liver Physiol., 2004, 287, G7–G17 CrossRef CAS PubMed.
- S. Soderlund, L. Brandt, A. Lapidus, P. Karlen, O. Brostrom, R. Lofberg, A. Ekbom and J. Askling, Decreasing time-trends of colorectal cancer in a large cohort of patients with inflammatory bowel disease, Gastroenterology, 2009, 136, 1561–1567 CrossRef ; quiz 1818–1569.
- J. Terzić, S. Grivennikov, E. Karin and M. Karin, Inflammation and Colon Cancer, Gastroenterology, 2010, 138, 2101–2114 CrossRef PubMed , e2105.
- L. Saulnier, P. E. Sado, G. Branlard, G. Charmet and F. Guillon, Wheat arabinoxylans: Exploiting variation in amount and composition to develop enhanced varieties, J. Cereal Sci., 2007, 46, 261–281 CrossRef CAS.
- E. Dornez, K. Gebruers, J. Delcour and C. Courtin, Grain-associated xylanases: occurrence, variability, and implications for cereal processing, Trends Food Sci. Technol., 2009, 20, 495–510 CrossRef CAS.
- M. Monobe, M. Maeda-Yamamoto, Y. Matsuoka, A. Kaneko and S. Hiramoto, Immunostimulating activity and molecular weight dependence of an arabinoxylan derived from wheat bran, J. Jpn. Soc. Food Sci. Technol., 2008, 55, 245–249 CrossRef CAS.
- S. Zhou, X. Liu, Y. Guo, Q. Wang, D. Peng and L. Cao, Comparison of the immunological activities of arabinoxylans from wheat bran with alkali and xylanase-aided extraction, Carbohydr. Polym., 2010, 81, 784–789 CrossRef CAS.
- L. Cao, X. Liu, T. Qian, G. Sun, Y. Guo, F. Chang, S. Zhou and X. Sun, Antitumor and immunomodulatory activity of arabinoxylans: A major constituent of wheat bran, Int. J. Biol. Macromol., 2011, 48, 160–164 CrossRef CAS PubMed.
- M. Akhtar, A. F. Tariq, M. M. Awais, Z. Iqbal, F. Muhammad, M. Shahid and E. Hiszczynska-Sawicka, Studies on wheat bran Arabinoxylan for its immunostimulatory and protective effects against avian coccidiosis, Carbohydr. Polym., 2012, 90, 333–339 CrossRef CAS PubMed.
- M. Mendis and S. Simsek, Production of structurally diverse wheat arabinoxylan hydrolyzates using combinations of xylanase and arabinofuranosidase, Carbohydr. Polym., 2015, 132, 452–459 CrossRef CAS PubMed.
- L. Van den Broek, R. Lloyd, G. Beldman, J. Verdoes, B. McCleary and A. Voragen, Cloning and characterization of arabinoxylan arabinofuranohydrolase-D3 (AXHd3) from Bifidobacterium adolescentis DSM20083, Appl. Microbiol. Biotechnol., 2005, 67, 641–647 CrossRef CAS PubMed.
- E. Taylor, N. Smith, J. Turkenburg, S. D'souza, H. Gilbert and G. Davies, Structural insight into the ligand specificity of a thermostable family 51 arabinofuranosidase, Araf51, from Clostridium thermocellum, Biochem. J., 2006, 395, 31–37 CrossRef CAS PubMed.
- W. Li, S. Zhang and C. Smith, The molecular structure features-immune stimulatory activity of arabinoxylans derived from the pentosan faction of wheat flour, J. Cereal Sci., 2015, 62, 81–86 CrossRef CAS.
- S. Gollapudi and M. Ghoneum, MGN-3/Biobran, modified arabinoxylan from rice bran, sensitizes human breast cancer cells to chemotherapeutic agent, daunorubicin, Cancer Detect. Prev., 2008, 32, 1–6 CrossRef CAS PubMed.
- M. Ghoneum and S. Gollapudi, Modified arabinoxylan rice bran (MGN-3/Biobran) sensitizes human T cell leukemia cells to death receptor (CD95)-induced apoptosis, Cancer Lett., 2003, 201, 41–49 CrossRef CAS PubMed.
- T. Collins, C. Gerday and G. Feller, Xylanases, xylanase families and extremophilic xylanases, FEMS Microbiol. Rev., 2005, 29, 3–23 CrossRef CAS PubMed.
- L. W. Doner and K. B. Hicks, Isolation of hemicellulose from corn fiber by alkaline hydrogen peroxide extraction, Cereal Chem., 1997, 74, 176–181 CrossRef CAS.
- H. Schwarz, M. Schmittner, A. Duschl and J. Horejs-Hoeck, Residual endotoxin contaminations in recombinant proteins are sufficient to activate human CD1c+ dendritic cells, PLoS One, 2014, 9, e113840 Search PubMed.
- S. I. ThermoFisher, Pierce™ LAL Chromogenic Endotoxin Quantitation Kit, https://www.thermofisher.com/order/catalog/product/88282, (accessed 11/04/2016).
-
J. Ryan, Endotoxins and cell culture, http://www.gongyingshi.com/item/doc/20141221/cc_endotoxins_tc_305_rev1.pdf.
- C. D. Pellegrina, O. Perbellini, M. T. Scupoli, C. Tomelleri, C. Zanetti, G. Zoccatelli, M. Fusi, A. Peruffo, C. Rizzi and R. Chignola, Effects of wheat germ agglutinin on human gastrointestinal epithelium: Insights from an experimental model of immune/epithelial cell interaction, Toxicol. Appl. Pharmacol., 2009, 237, 146–153 CrossRef PubMed.
- A. B. Samuelsen, A. Rieder, S. Grimmer, T. E. Michaelsen and S. H. Knutsen, Immunomodulatory activity of dietary fiber: arabinoxylan and mixed-linked beta-glucan isolated from barley show modest activities in vitro, Int. J. Mol. Sci., 2011, 12, 570–587 CrossRef CAS PubMed.
- A. Rieder, S. Grimmer, S. O. Kolset, T. E. Michaelsen and S. H. Knutsen, Cereal β-glucan preparations of different weight average molecular weights induce variable cytokine secretion in human intestinal epithelial cell lines, Food Chem., 2011, 128, 1037–1043 CrossRef CAS.
- E. O. Kim, K. J. Min, T. K. Kwon, B. H. Um, R. A. Moreau and S. W. Choi, Anti-inflammatory activity of hydroxycinnamic acid derivatives isolated from corn bran in lipopolysaccharide-stimulated Raw 264.7 macrophages, Food Chem. Toxicol., 2012, 50, 1309–1316 CrossRef CAS PubMed.
- M. G. Vernaza, V. P. Dia, E. Gonzalez de Mejia and Y. K. Chang, Antioxidant and antiinflammatory properties of germinated and hydrolysed Brazilian soybean flours, Food Chem., 2012, 134, 2217–2225 CrossRef CAS PubMed.
- E. Cario, I. M. Rosenberg, S. L. Brandwein, P. L. Beck, H. C. Reinecker and D. K. Podolsky, Lipopolysaccharide activates distinct signaling pathways in intestinal epithelial cell lines expressing Toll-like receptors, J. Immunol., 2000, 164, 966–972 CrossRef CAS.
- H. Q. Doan, K. A. Bowen, L. A. Jackson and B. M. Evers, Toll-like receptor 4 activation increases Akt phosphorylation in colon cancer cells, Anticancer Res., 2009, 29, 2473–2478 CAS.
- R. Y. Hsu, C. H. Chan, J. D. Spicer, M. C. Rousseau, B. Giannias, S. Rousseau and L. E. Ferri, LPS-induced TLR4 signaling in human colorectal cancer cells increases beta1 integrin-mediated cell adhesion and liver metastasis, Cancer Res., 2011, 71, 1989–1998 CrossRef CAS PubMed.
- R. M. Al-Sadi and T. Y. Ma, IL-1beta causes an increase in intestinal epithelial tight junction permeability, J. Immunol., 2007, 178, 4641–4649 CrossRef CAS.
- R. B. Sartor, Cytokines in intestinal inflammation: pathophysiological and clinical considerations, Gastroenterology, 1994, 106, 533–539 CrossRef CAS.
- Y. Murata, Y. Ishiguro, J. Itoh, A. Munakata and Y. Yoshida, The role of proinflammatory and immunoregulatory cytokines in the pathogenesis of ulcerative colitis, J. Gastroenterol., 1995, 30(Suppl 8), 56–60 Search PubMed.
- D. J. Waugh and C. Wilson, The interleukin-8 pathway in cancer, Clin. Cancer Res., 2008, 14, 6735–6741 CrossRef CAS PubMed.
- A. Yuan, J. J. Chen, P. L. Yao and P. C. Yang, The role of interleukin-8 in cancer cells and microenvironment interaction, Front. Biosci., 2005, 10, 853–865 CrossRef CAS.
- Q. Sun, F. Sun, B. Wang, S. Liu, W. Niu, E. Liu, C. Peng, J. Wang, H. Gao, B. Liang, Z. Niu, X. Zou and J. Niu, Interleukin-8 promotes cell migration through integrin αvβ6 upregulation in colorectal cancer, Cancer Lett., 2014, 354, 245–253 CrossRef CAS PubMed.
- X. Wang, S. Wang, Y. Li, F. Wang, X. Yang and J. Yao, Sulfated Astragalus polysaccharide can regulate the inflammatory reaction induced by LPS in Caco2 cells, Int. J. Biol. Macromol., 2013, 60, 248–252 CrossRef CAS PubMed.
- F. Liu, Y. Liu, V. C. H. Lui, J. R. Lamb, P. K. H. Tam and Y. Chen, Hypoxia modulates lipopolysaccharide induced TNF-α expression in murine macrophages, Exp. Cell Res., 2008, 314, 1327–1336 CrossRef CAS PubMed.
- R. M. Andrade, M. Wessendarp, J. A. Portillo, J. Q. Yang, F. J. Gomez, J. E. Durbin, G. A. Bishop and C. S. Subauste, TNF receptor-associated factor 6-dependent CD40 signaling primes macrophages to acquire antimicrobial activity in response to TNF-alpha, J. Immunol., 2005, 175, 6014–6021 CrossRef CAS.
- M. Zakharova and H. K. Ziegler, Paradoxical anti-inflammatory actions of TNF-alpha: inhibition of IL-12 and IL-23 via TNF receptor 1 in macrophages and dendritic cells, J. Immunol., 2005, 175, 5024–5033 CrossRef CAS.
- A. Sato, T. Zhang, L. Yonekura and H. Tamura, Antiallergic activities of eleven onions (Allium cepa) were attributed to quercetin 4′-glucoside using QuEChERS method and Pearson's correlation coefficient, J. Funct. Foods, 2015, 14, 581–589 CrossRef CAS.
- O. Takeuchi and S. Akira, Pattern Recognition Receptors and Inflammation, Cell, 2010, 140, 805–820 CrossRef CAS PubMed.
- U. Bocker, O. Yezerskyy, P. Feick, T. Manigold, A. Panja, U. Kalina, F. Herweck, S. Rossol and M. V. Singer, Responsiveness of intestinal epithelial cell lines to lipopolysaccharide is correlated with Toll-like receptor 4 but not Toll-like receptor 2 or CD14 expression, Int. J. Colorectal Dis., 2003, 18, 25–32 CrossRef PubMed.
- M. T. Abreu, E. T. Arnold, L. S. Thomas, R. Gonsky, Y. Zhou, B. Hu and M. Arditi, TLR4 and MD-2 expression is regulated by immune-mediated signals in human intestinal epithelial cells, J. Biol. Chem., 2002, 277, 20431–20437 CrossRef CAS PubMed.
- M. T. Abreu, P. Vora, E. Faure, L. S. Thomas, E. T. Arnold and M. Arditi, Decreased expression of Toll-like receptor-4 and MD-2 correlates with intestinal epithelial cell protection against dysregulated proinflammatory gene expression in response to bacterial lipopolysaccharide, J. Immunol., 2001, 167, 1609–1616 CrossRef CAS.
- J. Van De Walle, A. Hendrickx, B. Romier, Y. Larondelle and Y.-J. Schneider, Inflammatory parameters in Caco-2 cells: Effect of stimuli nature, concentration, combination and cell differentiation, Toxicol. in Vitro, 2010, 24, 1441–1449 CrossRef CAS PubMed.
- Y. Huang, N. Li, K. Liboni and J. Neu, Glutamine decreases
lipopolysaccharide-induced IL-8 production in Caco-2 cells through a non-NF-kappaB p50 mechanism, Cytokine, 2003, 22, 77–83 CrossRef CAS PubMed.
- K. L. Puig, G. D. Manocha and C. K. Combs, Amyloid precursor protein mediated changes in intestinal epithelial phenotype in vitro, PLoS One, 2015, 10, e0119534 Search PubMed.
- E. Muto, M. Dell'Agli, E. Sangiovanni, N. Mitro, M. Fumagalli, M. Crestani, E. De Fabiani and D. Caruso, Olive oil phenolic extract regulates interleukin-8 expression by transcriptional and post-transcriptional mechanisms in Caco-2 cells, Mol. Nutr. Food Res., 2015, 59, 1217–1221 CAS.
- X. Ke, G. Hu, W. Fang, J. Chen, X. Zhang, C. Yang, J. Peng, Y. Chen and T. J. Sferra, Qing Hua Chang Yin inhibits the LPS-induced activation of the IL-6/STAT3 signaling pathway in human intestinal Caco-2 cells, Int. J. Mol. Med., 2015, 35, 1133–1137 Search PubMed.
- A. Cianciulli, R. Calvello, P. Cavallo, T. Dragone, V. Carofiglio and M. A. Panaro, Modulation of NF-kappaB activation by resveratrol in LPS treated human intestinal cells results in downregulation of PGE2 production and COX-2 expression, Toxicol. In Vitro, 2012, 26, 1122–1128 CrossRef CAS PubMed.
- M. A. Panaro, V. Carofiglio, A. Acquafredda, P. Cavallo and A. Cianciulli, Anti-inflammatory effects of resveratrol occur via inhibition of lipopolysaccharide-induced NF-kappaB activation in Caco-2 and SW480 human colon cancer cells, Br. J. Nutr., 2012, 108, 1623–1632 CrossRef CAS PubMed.
- S. Akira and K. Takeda, Toll-like receptor signalling, Nat. Rev. Immunol., 2004, 4, 499–511 CrossRef CAS PubMed.
- M. Fujihara, M. Muroi, K. Tanamoto, T. Suzuki, H. Azuma and H. Ikeda, Molecular mechanisms of macrophage activation and deactivation by lipopolysaccharide: roles of the receptor complex, Pharmacol. Ther., 2003, 100, 171–194 CrossRef CAS PubMed.
- M. Guha and N. Mackman, LPS induction of gene expression in human monocytes, Cell. Signalling, 2001, 13, 85–94 CrossRef CAS PubMed.
- J. J. Volman, J. D. Ramakers and J. Plat, Dietary modulation of immune function by beta-glucans, Physiol. Behav., 2008, 94, 276–284 CrossRef CAS PubMed.
- M. Brook, G. Sully, A. R. Clark and J. Saklatvala, Regulation of tumour necrosis factor α mRNA stability by the mitogen-activated protein kinase p38 signalling cascade, FEBS Lett., 2000, 483, 57–61 CrossRef CAS PubMed.
- S. J. Lee, H. K. Rim, J. Y. Jung, H. J. An, J. S. Shin, C. W. Cho, Y. K. Rhee, H. D. Hong and K. T. Lee, Immunostimulatory activity of polysaccharides from Cheonggukjang, Food Chem. Toxicol., 2013, 59, 476–484 CrossRef CAS PubMed.
- I. A. Schepetkin and M. T. Quinn, Botanical polysaccharides: Macrophage immunomodulation and therapeutic potential, Int. Immunopharmacol., 2006, 6, 317–333 CrossRef CAS PubMed.
- A. M. S. Assreuy, D. M. Gomes, M. S. J. da Silva, V. M. Torres, R. C. L. Siqueira, A. d. F. Pires, D. N. Criddle, N. M. N. de Alencar, B. S. Cavada and A. H. Sampaio, Biological effects of a sulfated-polysaccharide isolated from the marine red algae Champia feldmannii, Biol. Pharm. Bull., 2008, 31, 691–695 CAS.
- T. M. Earl, I. B. Nicoud, J. M. Pierce, J. P. Wright, N. E. Majoras, J. E. Rubin, K. P. Pierre, D. L. Gorden and R. S. Chari, Silencing of TLR4 decreases liver tumor burden in a murine model of colorectal metastasis and hepatic steatosis, Ann. Surg. Oncol., 2009, 16, 1043–1050 CrossRef CAS PubMed.
- A. Oblak and R. Jerala, Toll-like receptor 4 activation in cancer progression and therapy, Clin. Dev. Immunol., 2011, 2011, 609579 Search PubMed.
- S. Awasthi, Toll-like receptor-4 modulation for cancer immunotherapy, Front. Immunol., 2014, 5, 328 Search PubMed.
- N. M. Sahasrabudhe, H. A. Schols, M. M. Faas and P. de Vos, Arabinoxylan activates Dectin-1 and modulates particulate beta-glucan-induced Dectin-1 activation, Mol. Nutr. Food Res., 2016, 60, 458–467 CAS.
- M. Martinez-Cutillas, N. Mañé, D. Gallego, M. Jimenez and M. T. Martin, EP2 and EP4 receptors mediate PGE2 induced relaxation in murine colonic circular muscle: Pharmacological characterization, Pharmacol. Res., 2014, 90, 76–86 CrossRef CAS PubMed.
- I. Dey, M. Lejeune and K. Chadee, Prostaglandin E2 receptor distribution and function in the gastrointestinal tract, Br. J. Pharmacol., 2006, 149, 611–623 CrossRef CAS PubMed.
- C. Porcher, B. Horowitz, S. M. Ward and K. M. Sanders, Constitutive and functional expression of cyclooxygenase 2 in the murine proximal colon, Neurogastroenterol. Motil., 2004, 16, 785–799 CrossRef CAS PubMed.
- P. Cong, V. Pricolo, P. Biancani and J. Behar, Abnormalities of Prostaglandins and Cyclooxygenase Enzymes in Female Patients With Slow-Transit Constipation, Gastroenterology, 2007, 133, 445–453 CrossRef CAS PubMed.
- A. Grishin, J. Wang, D. Hackam, F. Qureshi, J. Upperman, R. Zamora and H. R. Ford, p38 MAP kinase mediates endotoxin-induced expression of cyclooxygenase-2 in enterocytes, Surgery, 2004, 136, 329–335 CrossRef PubMed.
- A. V. Grishin, J. Wang, D. A. Potoka, D. J. Hackam, J. S. Upperman, P. Boyle, R. Zamora and H. R. Ford, Lipopolysaccharide induces cyclooxygenase-2 in intestinal epithelium via a noncanonical p38 MAPK pathway, J. Immunol., 2006, 176, 580–588 CrossRef CAS.
- Y. J. Surh, K. S. Chun, H. H. Cha, S. S. Han, Y. S. Keum, K. K. Park and S. S. Lee, Molecular mechanisms underlying chemopreventive activities of anti-inflammatory phytochemicals: down-regulation of COX-2 and iNOS through suppression of NF-kappa B activation, Mutat. Res., 2001, 480–481, 243–268 CrossRef CAS PubMed.
- M. Fukata, A. Chen, A. Klepper, S. Krishnareddy, A. S. Vamadevan, L. S. Thomas, R. Xu, H. Inoue, M. Arditi, A. J. Dannenberg and M. T. Abreu, Cox-2 is regulated by Toll-like receptor-4 (TLR4) signaling: Role in proliferation and apoptosis in the intestine, Gastroenterology, 2006, 131, 862–877 CrossRef CAS PubMed.
- T. Kawasaki and T. Sata, Trauma-hemorrhage and dendritic cell functions: A critical review of splenic dendritic cell dysfunction following trauma-hemorrhage and therapeutic approach., OA Anaesth., 2013, 1(2), 15 Search PubMed.
- E. L. Adams, P. J. Rice, B. Graves, H. E. Ensley, H. Yu, G. D. Brown, S. Gordon, M. A. Monteiro, E. Papp-Szabo and D. W. Lowman, Differential high-affinity interaction of dectin-1 with natural or synthetic glucans is dependent upon primary structure and is influenced by polymer chain length and side-chain branching, J. Pharmacol. Exp. Ther., 2008, 325, 115–123 CrossRef CAS PubMed.
- M. S. Mikkelsen, B. M. Jespersen, A. Mehlsen, S. B. Engelsen and H. Frokiaer, Cereal bets-glucan immune modulating activity depends on the polymer fine structure, Food Res. Int., 2014, 62, 829–836 CrossRef CAS.
|
This journal is © The Royal Society of Chemistry 2017 |