DOI:
10.1039/C6FO01225F
(Paper)
Food Funct., 2017,
8, 232-240
A comparison of RS4-type resistant starch to RS2-type resistant starch in suppressing oxidative stress in high-fat-diet-induced obese rats†
Received
17th August 2016
, Accepted 19th November 2016
First published on 22nd November 2016
Abstract
The anti-obesity effects of two types of resistant starch (RS) in high-fat-diet-induced obese rats were investigated. The serum triglycerides, total cholesterol and malondialdehyde concentrations were significantly reduced, and the total antioxidant capacity, superoxide dismutase levels and glutathione peroxidase activity were increased by RS2 and RS4 consumption compared to the obesity group. A significant reduction in the serum glucose level and elevations in hepatic lipid metabolic enzyme activities were observed only for RS4 administration. Moreover, the expression levels of the fatty acid synthesis associated genes ACC and Fads1, the triglyceride synthesis and metabolism-related gene SREBP-1, the adipocyte differentiation gene PPARγ, the cholesterol synthesis associated gene HMGCR, and the gluconeogenesis associated gene GAPDH were all significantly down-regulated, whilst the lipid oxidation gene Acox1 and the liver function genes Gsta2, Nqo1, and Gclm were up-regulated in both administered groups. Additionally, RS4 performed well in up-regulating the expressions of Gsta2, Gsta3, Nqo1, and Egfr, and down-regulating LXRα, Igfbp1, and Pml. RS4 exhibited great advantages in reducing oxidative stress compared with RS2.
1. Introduction
Obesity is defined as a chronic metabolic disease caused by an energy imbalance which results in abnormal or excessive body fat accumulation.1 It is reported that obesity is closely related to glucose and lipid metabolism disorders.2 Exposure to a high-fat and high-energy diet over a long period of time could result in the abnormal accumulation of fat, together with increased lipid levels. As a consequence, a large amount of free fatty acid is created by lipid degradation sometimes associated with a reduction in hepatic glycogen synthesis and an increase in glucose output could occur, further contributing to dysfunctional lipid metabolism. Obesity is a worldwide epidemic and according to the World Health Organization (WHO), approximately 13% of the world's adult population (11% of men and 15% of women) was obese in 2014. Obesity is a major risk factor for a number of chronic diseases, including diabetes, cardiovascular diseases and cancer,3–5 and affects virtually all age and socioeconomic groups and is thus rapidly becoming a health burden in both developed and developing countries (Kelaher et al., 2008).6,7
Studies have shown that obesity is coupled with an altered redox state and an increased metabolic risk.8–10 Oxidative stress can be a consequence of and also a trigger for obesity. Oxidative stress is generally associated with an imbalance in net levels of reactive oxygen species (ROS) relative to the antioxidant capacity of the body and reduced antioxidant defenses, which results in an accumulation of oxidative products. ROS include free radicals like superoxide (O2−), hydroxyl radicals (˙OH), hydrogen peroxide (H2O2) and hydroperoxyl radicals
.11 Increased ROS production in accumulated fat leads to an elevation of systemic oxidative stress, further leading to dysregulated production of adipocytokines.12
Resistant starch is a fermentable carbohydrate that has been shown to have similar properties as dietary fiber and may contribute to satiety and exert a beneficial role in weight regulation.13,14 RS passes through the upper digestive tract to the colon, where it is fermented by bacteria, producing important metabolites, such as short chain fatty acids (SCFAs).15,16 These metabolites have been shown to have important biological functions associated with colon cancer prevention, hypoglycemic effects, inhibition of fat accumulation and regulation of macronutrient metabolism.17–20 RS includes an extremely broad and diverse range of materials and a number of different types exist (RS1–5), according to origin and digestion resistibility and are classified as physically inaccessible starch (RS1), high-amylose starch (RS2), retrograded starch (RS3), chemically modified starch (RS4) and starch–lipid complex (RS5).21–23 Acetylated starch (with a lower substitution degree) has been widely used in the food industry, and studies have proposed its potential functional role as an emulgator, a plastic agent and other additives in instant food and functional food.24,25 However, little research has been reported regarding the question whether RS4 shows any advantage over RS2 in terms of anti-obesity effects. This study comprehensively compares RS2 and RS4 in terms of their ability to suppress oxidative stress, and whether esterification contributes to a more efficient and positive regulation of obesity.
2. Experimental section
2.1. Sample preparation
High amylose starch (HAS, referred to as RS2) was purchased from Ingredion Ltd (NSW, Australia). Esterified high amylose starch (EHAS, referred to as RS4) was prepared as follows: 10 g of HAS was mixed thoroughly in 10 mL acetic acid and incubated at room temperature for 5 min, and then 30 mL acetic anhydride was added and incubated for a further 10 min. The catalytic agent, which was obtained by diluting 0.38 mL of 98% concentrated sulfuric acid in 10 mL acetic acid, was slowly added to the above solution, and the reaction was allowed to proceed for 150 min at a constant temperature of 70 °C. The esterification reaction was completed by adding distilled water (1
:
10, reaction solution). The prepared starch was collected in a Buchner funnel and washed using distilled water. When a neutral pH was reached, the filtrate was transferred onto glass plates followed by vacuum drying at 50 °C. The degree of substitution of EHAS was 2.08, determined by the BaCl2–gelatin turbidity method.26
2.2. Animals and treatment
Healthy male Wistar rats (non-obese) of 110 ± 10 g body weight were purchased from the animal house, the Chinese Military Medical Science Academy and fed in the clean-grade facility of the laboratory animal center in Tianjin University of Science and Technology. After one week's adaptive feeding with a basal diet, thirty-two rats were divided into four groups randomly: NC: normal control (normal rats fed with basal diet); MC: model control (basal diet with a high fat component to induce obesity); HAS: high amylose starch (interventional group: obese rats induced by a high fat diet, and fed with a dietary intervention of HAS, 2 g per day); EHAS: esterified high amylose starch (interventional group: obese rats induced by a high fat diet, and fed with an intervention of EHAS, 2 g per day). The basal diet consisted of the AIN-76 diet (American Institute of Nutrition, 1980).27
The rats were housed in plastic cages (4 rats per cage) with free access to drinking water, under controlled conditions of humidity (40%–60%), light (12 h/12 h light/dark cycle) and temperature (20–25 °C). After one week's adaptive feeding, the gavage method was adopted, in which resistant starch powder was mixed with water and delivered by gavage to the rats in interventional groups twice a day at the rate of 2 g d−1. For NC and MC groups, an equal amount of normal saline was gavaged to the control rats. During the experimental course of 6 weeks, body mass was recorded once a week. At the end of the experimental period, the rats were fasted overnight, and blood samples were taken from the arteria femoralis. The serum was separated from whole blood by centrifugation at 4 °C and 3000 rpm for 10 min. Liver, epididymal white adipose tissue and perirenal white adipose tissue were quickly removed after sacrifice and weighed. Samples of serum and liver tissue were collected and stored at −80 °C until analysis of biological parameters and liver tissue gene expression. There was no casual or obvious signs of toxicity throughout the course of the experiments and all rats involved survived.
2.3. Morphological analysis
Scanning electron microscopy (SEM) observation.
The freeze-dried RS samples were mounted on circular aluminum stubs with carbon electroconductive adhesive tape and sputtered with gold to create electric conductivity. The morphologies of un-esterified and esterified samples were observed under vacuum using an SU-1510 Scanning Electron Microscope (Hitachi, Japan). The electron images were acquired at an accelerating voltage of 40 kV.
Fourier transform infrared spectroscopy (FTIR) analysis.
Following a tableting process using KBr, starch samples were scanned using a Fourier transform infrared spectrometer (Vector22, Bruker, German) within a spectrum span of 400–4000 cm−1, a distinguishability of 4 cm−1, and a scanning time of 16 s.
2.4. Analysis of blood serum
Blood lipid indices evaluated in the current study included triglyceride (TG), total cholesterol (TCH), high density lipoprotein cholesterol (HDL-c) and low-density lipoprotein cholesterol (LDL-c). The specific measurement procedures and final calculations were performed according to TG, TCH, HDL-c and LDL-c assay kit (Jian Cheng Biotechnology Co., Ltd, Nanjing, China) specifications.
2.5. Malondialdehyde (MDA)
Malondialdehyde (MDA) is a final product of the lipid oxidation process, which can directly reflect the degree of lipid peroxidation and indirectly reflect the degree of cellular damage. The thiobarbituric acid (TBA) method was used to measure the MDA content.28 A red end product is produced during the condensation reaction between MDA and thiobarbituric acid, which results in absorption at 532 nm. The specific measurement procedure was performed according to MDA assay kit (Jian Cheng Biotechnology Co., Ltd, Nanjing, China) specifications. The MDA content was calculated using the following equation: |  | (1) |
where OD is the absorption value of the test sample, ODc is the absorption value of the control, ODs is the absorption value of the standard sample, ODb is the absorption value of the blank, c is the concentration of the standard sample (10 nmol mL−1), and f is the dilution factor.
2.6. Total superoxide dismutase (T-SOD)
Total superoxide dismutase (T-SOD) was determined using an SOD assay kit (Jian Cheng Biotechnology Co., Ltd, Nanjing, China). A superoxide anion free radical produced systemically in the body can oxidize hydroxylamine, creating nitrite that presents as an amaranthine color following color development. SOD in the serum of test rats can suppress superoxide anion free radical formation, further reducing the nitrite concentration. The reduction of nitrite can be measured using a colorimetric method, as well as the SOD activity in the serum. |  | (2) |
where OD is the absorption value of the test sample; ODc is the absorption value of the control; f1 is the dilution factor of the reaction system; and f2 is the dilution factor of the sample.
2.7. Glutathione peroxidase (GSH-PX)
The method is based on the reaction of 5,5-dithiobis(2-nitrobenzoic acid) (DTNB) with reduced glutathione peroxidase (GSH-PX) to produce a stable yellow compound, and its absorbance can be measured at 412 nm (ref. 29) using an assay kit (Jian Cheng Biotechnology Co., Ltd, Nanjing, China). GSH-PX activity can be calculated (eqn (3)) according to the reduced chromogen, which is directly proportional to GSH-PX concentration. |  | (3) |
where OD is the absorption value of samples with enzyme; OD0 is the absorption value of samples without enzyme; ODs is the absorption value of the standard sample; ODb is the absorption value of the blank; c is the concentration of the standard sample (20 μmol mL−1); f1 is the dilution factor of the reaction system; and f2 is the dilution factor of the sample.
2.8. Total antioxidant capacity (T-AOC)
The T-AOC was determined by the ferric reducing-antioxidant power (FRAP) assay method. In the experiment, the ferric ion (Fe3+) can be reduced to the ferrous (Fe2+) form by antioxidants and the color that develops can be detected at 520 nm with a spectrophotometer and is proportional to the concentration of antioxidative substances. The specific measurement procedure was performed based on a T-AOC assay kit (Jian Cheng Biotechnology Co., Ltd, Nanjing, China). The T-AOC content was calculated using the following equation: |  | (4) |
where OD is the absorption value of test samples; ODc is the absorption value of the control; V is the total reacting solution; V1 is the volume of the test sample; f is the dilution factor of the sample.
2.9. Determination of hepatic lipid metabolism enzymes
Gutamic oxaloacetic transaminase (GOT).
The interchange of the amido group and the ketonic group between α-oxoglutarate and asparaginic acid with the existence of AST/GOT leads to the formation of glutamic acid and oxaloacetic acid, and the latter is automatically decarboxylated to pyruvic acid. 2,4-Dinitrophenylhydrazone pyruvic acid, the product of pyruvic acid and 2,4-dinitrophenylhydrazine, presents as a reddish brown color in basic solution. GOT activity was measured by colorimetric analysis using an AST/GOT assay kit (Jian Cheng Biotechnology Co., Ltd, Nanjing, China).
Glutamic-pyruvic transaminase (GPT).
Glutamic-pyruvic transaminase (GPT) reacts with alanine and α-oxoglutarate at 37 °C, pH 7.4, forming pyruvic acid and glutamic acid. This reaction is completed by adding DNPH and incubation for 30 min, producing pyruvate phenylhydrazone that results in a reddish brown color under basic conditions and the absorbance was monitored at 510 nm. GPT activity was measured by colorimetric analysis using an ALT/GPT assay kit (Jian Cheng Biotechnology Co., Ltd, Nanjing, China).
Alkaline phosphatase (AKP).
Alkaline phosphatase (AKP) decomposes disodium phenyl phosphate to produce free phenol and phosphoric acid. The action of phenol and 4-aminoantipyrine in a basic solution coupled with oxidation by potassium ferricyanide produces perylenequinonoid derivatives with an associated red color that reflects enzyme activity.
2.10. Expression analysis of genes involved in lipid metabolism, oxidative stress and liver function
The rats were dissected immediately after sacrifice. The liver was weighed, immediately frozen in liquid nitrogen and stored at −80 °C until RNA extraction. The total RNA was extracted from tissues using Trizol reagent (Takara), treated with RNase-free DNase to remove any contaminating genomic DNA and transcribed to cDNA with a PrimeScript™ RT reagent kit with gDNA Erase (TaKaRa) according to the manufacturer's instructions. Real-time quantitative PCR reactions were performed using a SYBR® Premix Ex Taq™ II (TaKaRa) on a CFX96 Real-Time PCR Detection System (Mx-3000p™, Jitai Biological Technology Co., Shanghai, China). Real-time PCR was performed with the following parameters: initial denaturation at 95 °C for 5 min, and then 40 cycles of 95 °C for 30 s, 58–60 °C for 30 s, 72 °C for 30 s, and 72 °C for 10 min. The 18S rRNA gene was used as an internal control to normalize target gene expression. Three replicates of each reaction were carried out, and the relative transcript quantity was calculated according to the method of 2−ΔΔCT.30 The primer sequences of ACC (acetyl-CoA carboxylase glycogen), FAS (fatty acid synthase), Acox1 (acyl-CoA oxidase 1), SREBP-1 (sterol regulatory element binding protein-1), Acly (ATP citrate lyase), Fads1 (fatty acid desaturase 1), Gpam (glycerol-3-phosphate acyltransferase mitochondrial), Dgat1 (diacylglycerol acyltransferase 1), PPARγ (peroxisome proliferator-activated receptor γ), LXRα (liver X receptor α), HMGCR (3-hydroxy-3-methylglutaryl coenzyme A reductase), FATP1 (fatty acid transport protein 1), FABP4 (fatty acid binding protein 4), Gsta2 (glutathione S-transferase, alpha 2), Gsta3 (glutathione S-transferase, alpha 3), Gstm2 (glutathione S-transferase Mu2), Nqo1 (NAD(P)H quinone oxidoreductase 1), Gclc (glutamate-cysteine ligase catalytic subunit), Gclm (glutamate-cysteine ligase modifier subunit), VEGF (vascular endothelial growth factor), Egfr (epidermal growth factor receptor), Igfbp1 (insulin-like growth factor binding protein 1), Pml (promyelocytic leukemia), GAPDH (glyceraldehyde-3-phosphate dehydrogenase) and 18S are shown in the ESI.†
2.11. Ethics statement
All animal procedures were approved by the Ethical Committee for the Experimental Use of Animals at the Center for Drug Safety Evaluation, Tianjin University of Science & Technology (approval no. 13/051/MIS).
2.12. Statistical analysis
All analyses were conducted in triplicate and statistical analyses were performed using the Statistical Package for Social Science (SPSS) program. Results were expressed as the mean ± standard deviation (SD). The analysis of variance was performed at the 5% level of significance.
3. Results
3.1. SEM and FTIR analyses
It can be seen from SEM images (Fig. 1a) that the HAS particles are similar in size, mostly round in shape with a smooth surface, without holes and fissures. However, EHAS particles aggregate together as shown in Fig. 1(b). The initial reaction on the starch particle surface first causes HAS particles to lose their scattered and integrated characteristics. As the reaction progresses, esterification also occurs inside and a loose and porous structure is formed, and further accelerated esterified reaction leads to fracture and fragmentation.
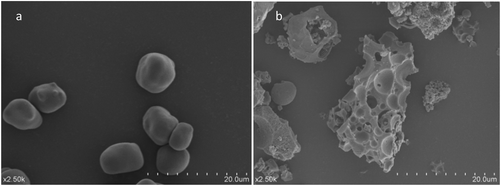 |
| Fig. 1 Effect of esterification on the morphology of starch granules: (a) native starch; (b) starch acetate. | |
The changes in the FTIR spectrum before and after the esterification reaction were recorded and are shown in Fig. 2. It was noted that the stretching vibration of C–O in HAS led to the formation of absorption peaks at 1200 cm−1, 1086 cm−1 and 1026 cm−1. The characteristic absorption peak at 2924 cm−1 was caused by the stretching vibration of C–H, and the wider absorption peak at 3450 cm−1 by O–H.
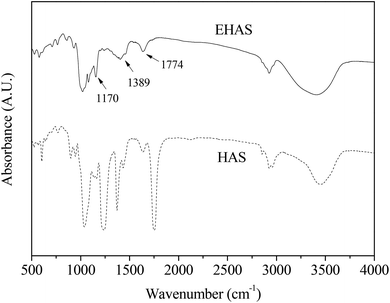 |
| Fig. 2 Difference in the FTIR spectrum of native starch and starch acetate. HAS: high amylose starch group, EHAS: esterified high amylose starch group. | |
Three new absorption peaks were found in EHAS at 1170 cm−1, 1389 cm−1 and 1774 cm−1, which were a C–O–C stretching vibrational absorption, a C–H bending vibrational absorption, and a C
O anti-symmetric stretching vibrational absorption, respectively. An average reduction in absorption peaks of EHAS was noted compared with raw starch, resulting from the substitution of hydroxy and decreases in the number of hydroxy groups, which indicated that hydroxyl hydrogen in glucose units was increasingly substituted and that the acetyl number increased with substitution degree increasing.
3.2. Blood glucose and lipid levels
At the end of the animal experiment, the administration of EHAS in the high fat diet was found to reduce the body weight and body fat ratio, although there was no significant difference between EHAS and HAS administration (in TS2 and TS3). The blood glucose data obtained from the rats during the treatment period is shown in Table 1. By the end of the 6th week, there was a significant increase in the blood glucose of high fat diet fed rats compared to the control group. During 6 weeks’ treatment with HAS and EHAS, the blood glucose levels of obese rats were shown to be reduced when compared with initial values. Moreover, a significant reduction in the blood glucose level was observed at the end of 6 weeks of treatment with EHAS in the obese rats compared to the MC group, which reflects the more efficient regulation of EHAS in lowering the blood glucose level.
Table 1 Effect of different treatments on the blood glucose level of rats in different groups (mmol L−1)
Groups |
0 W |
1 W |
2 W |
3 W |
4 W |
5 W |
6 W |
NC: normal control, MC: model control, HAS: high amylose starch group, EHAS: esterified high amylose starch group. Different lowercase letters above the same column indicate a significant difference (p ≤ 0.05); the results are the average values of three replicates ± standard deviation. |
NC |
6.73 ± 0.60a |
6.08 ± 0.50a |
6.23 ± 1.30a |
6.11 ± 0.50a |
5.83 ± 0.80a |
5.63 ± 0.80a |
5.61 ± 0.80b |
MC |
7.05 ± 0.50a |
6.46 ± 0.60a |
6.55 ± 0.60a |
6.38 ± 0.40a |
6.32 ± 0.30a |
6.28 ± 0.80a |
6.76 ± 0.60a |
HAS |
6.84 ± 0.40a |
6.26 ± 0.40a |
6.34 ± 0.30a |
6.00 ± 0.60a |
6.01 ± 0.70a |
5.91 ± 0.90a |
5.86 ± 0.40ab |
EHAS |
6.49 ± 0.64a |
6.34 ± 0.64a |
6.34 ± 0.74a |
5.51 ± 0.47a |
5.47 ± 0.53a |
5.47 ± 0.53a |
5.70 ± 0.19b |
At the end of the 6th week, the TG, TCH, HDL-c and LDL-c content in serum was measured. Table 2 summarizes the blood lipid levels of tested rats during the experimental period. Compared with the MC group, the HAS and EHAS groups had significantly decreased TG and TCH levels, and slightly decreased LDL-c. Additionally, higher values of HDL-c were seen in the HAS and EHAS groups when compared with the high fat-diet fed rats. Furthermore the EHAS group showed the most significant positive effect in lowering the TCH level when compared to the HAS group.
Table 2 Effect of different treatments on blood lipid compositions of rats in different groups (mmol L−1)
Groups |
Triglyceride (TG) |
Total cholesterol (TCH) |
High density lipoprotein cholesterol (HDL-c) |
Low density lipoprotein cholesterol (LDL-c) |
NC: normal control, MC: model control, HAS: high amylose starch group, EHAS: esterified high amylose starch group. Different lowercase letters above the same column indicate a significant difference (p ≤ 0.05); the results are the average values of three replicates ± standard deviation. |
NC |
1.34 ± 0.35b |
2.46 ± 0.46bc |
1.07 ± 0.27a |
1.18 ± 0.26a |
MC |
2.43 ± 0.26a |
3.85 ± 0.36a |
0.42 ± 0.09b |
1.99 ± 0.97a |
HAS |
1.77 ± 0.18b |
2.98 ± 0.28b |
0.60 ± 0.13b |
1.90 ± 0.49a |
EHAS |
1.51 ± 0.32b |
2.17 ± 0.26c |
0.66 ± 0.08b |
1.75 ± 0.87a |
3.3. T-AOC, T-SOD, MDA and GSH-PX levels
Our results (Fig. 3) showed a statistically significant increase in the serum levels of MDA and also a decrease in GSH-PX levels in the obese rats when compared to the control group (P < 0.05). T-AOC and T-SOD levels of obese rats were lower than the normal rats, but these differences were not significant. The HAS group showed significantly higher levels of T-AOC compared to the MC group and both HAS and EHAS groups showed higher T-SOD levels, compared to the MC group, with EHAS being the highest. Significant decreases in MDA content were seen in both HAS and EHAS groups, but no significant difference was seen between the two groups. There were significant decreases in GSH-PX levels in the HAS and EHAS treated rats compared with that of MC, and the HAS group showed a 15.25% higher level of GSH-Px compared to the EHAS group.
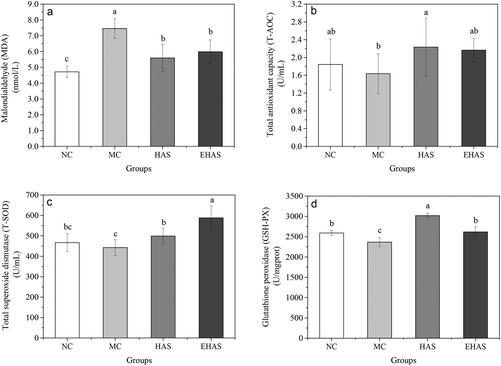 |
| Fig. 3 Changes in malonaldehyde (MDA) (a), total antioxidant capacity (T-AOC) (b), total superoxide dismutase (T-SOD) (c) and glutathione peroxidase (GSH-PX) (d) levels in rats of four groups after six weeks’ experiment. NC: normal control, MC: model control, HAS: high amylose starch group, EHAS: esterified high amylose starch group. Different lowercase letters above the bar indicate a significant difference (p < 0.05). | |
3.4. GPT, GOT and AKP levels
Table 3 shows significantly increased serum levels of GPT, GOT and AKP in the obese rats compared to the control group. There was also a significant decrease in the activities of GPT, GOT and AKP enzymes in EHAS administered rats. In contrast, the decrease seen in enzyme activities induced by HAS was not significant. GSH levels were significantly decreased in the obese rats compared to the control.
Table 3 Changes in GPT, GOT and AKP levels in rats following six weeks’ treatment
Groups |
Glutamic-pyruvic transaminase (GPT) (mU L−1) |
Gutamic oxaloacetic transaminase (GOT) (mU L−1) |
Alkaline phosphatase (AKP) (mU L−1) |
NC: normal control, MC: model control, HAS: high amylose starch, EHAS: esterified high amylose starch. Different lowercase letters above the same column indicate a significant difference (p ≤ 0.05); the results are the average values of three replicates ± standard deviation. |
NC |
30.66 ± 5.59c |
51.91 ± 14.57ab |
251.69 ± 48.31c |
MC |
41.28 ± 6.29a |
64.93 ± 21.50a |
409.86 ± 53.86a |
HAS |
39.33 ± 7.63ab |
57.56 ± 8.13a |
409.88 ± 48.86a |
EHAS |
33.16 ± 5.29bc |
41.77 ± 5.10b |
349.29 ± 27.36b |
3.5. Expression analysis of genes involved in metabolism and oxidative stress
We investigated the expression of lipid metabolic genes and oxidative stress related genes. The relative expression of genes involved in lipid metabolism, liver metabolism and oxidative stress can be seen in Fig. 4. Significant down-regulations of some genes involved in lipid metabolism, such as ACC, Fads1, SREBP-1, PPARγ, LXRα and HMGCR, were observed in the experimental groups compared with the obesity control group. However, no significant differences were observed in the expression levels of Acly, FAS, Gpam, Dgat1 and FATP1 for both HAS and EHAS treatment groups compared with the MC group. In addition, the relative expression of Acox was significantly up-regulated in the interventional groups, with the maximum increase seen in the HAS group. The relative expression of genes involved in liver function (Gsta2, Gsta3, Nqo1, Gclc and Gclm) was up-regulated in the experimental groups compared with the high fat-diet fed group, except for the Gstm2 gene. Significant down-regulations of the relative expression of Igfbp1 and Pml were seen in the EHAS group, VEGF and GAPDH for both intervention groups.
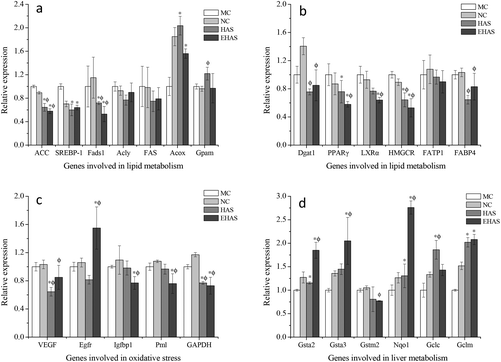 |
| Fig. 4 Changes in the expression of genes involved in lipid metabolism (a & b), oxidative stress (c) and liver metabolism (d) following the treatment. NC: normal control, MC: model control, HAS: high amylose starch group, EHAS: esterified high amylose starch group. “*” indicates p < 0.05 vs. NC and “ϕ” indicates p < 0.05 vs. MC. | |
4. Discussion
Obesity represents a significant threat to human health, and is a strong risk factor for developing dyslipidemia, diabetes mellitus, fatty liver (an inducing factor for nonalcoholic fatty liver disease) and cardiovascular diseases.31–34
In this study, a model of obesity in rats was induced by using a high fat diet formula. The high fat-diet resulted in increased serum levels of glucose, TG, TCH, and LDL-c and lower levels of HDL-c when compared to the normal control. Dyslipidemia is in direct proportion to the degree of obesity. The rising blood glucose levels may be attributed to the large accumulation of fat in the liver and the enhancement of gluconeogenesis induced by hepatic cells, reducing the transformation of glucose into fat caused by lipodystrophy. The remarkably increased triglyceride content of the liver has been shown to be due to the increased influx of excess non-esterified fatty acids (NEFAs) into the liver.35 High serum TG reduces HDL-c concentrations through the exchange of very low density lipoprotein triglycerides with HDL cholesterol esters.36 The intervention of resistant starch lowered the blood glucose, TG, TCH and LDL-c concentrations compared with obesity induced control animals; the effect was most significant in the EHAS group. This could be associated with increased levels of the bacterial populations in the gut. It has been reported that resistant starch administration greatly increased the short-chain fatty acids via gut microbiota fermentation, in particular, propionate and butyrate, which were associated with inhibitory effects on cholesterol synthesis.37
Obesity is a chronic inflammatory disease characterized by higher than normal oxidative stress. Lipid peroxidation, a free radical-generating process, occurs on every cellular membranous structure. Free radicals in high concentrations damage DNA, proteins, carbohydrates and lipid constituents and compromise cell function.38,39 A great many studies in rats have demonstrated that obesity is connected with increased oxidative stress and increased lipid peroxidation.40,41 Antioxidant enzymes play a central role in the body antioxidant system, the activity of which is closely linked with antioxidant status in the body. The major antioxidant enzymes include superoxide dismutase, glutathione peroxidase and catalase. Our results showed that a high fat-diet led to the increased production of MDA, as well as a reduction in T-AOC, T-SOD and GSH-PX levels compared with the normal control. Decreased activity of antioxidant enzymes may be attributed to their rapid consumption and depletion of their storage in the body when fighting free radicals generated during the development of obesity.42 The MDA content of the RS treated groups significantly decreased, whilst the T-AOC, T-SOD and GSH-PX levels significantly increased when compared with the obesity control group. These results reflect the strong anti-lipid peroxidation effects of these two types of resistant starch, indicating that HAS and EHAS can contribute to improving the activity of antioxidant enzymes in animal models of obesity and diminish the obesity-induced lipid peroxidation via scavenging of free radicals. Furthermore, amelioration of oxidative stress by resistant starch was demonstrated to be associated with the alteration of gut microbiota43 and the improved gut epithelial barrier structure and gastrointestinal tract function.44
In order to investigate the effect of RS2 and RS4 on the regulation of lipid metabolism, we also measured serum GPT, GOT and AKP levels. These three enzymes mainly exist in hepatic cells, and are released into the blood when liver cell necrosis occurs or degeneration occurs due to cell membrane rupturing, leading to increased serum activity.45 The results of this study show that the serum levels of GPT, GOT and AKP were elevated under the high fat-diet, and were significantly reduced following administration of HAS and EHAS compared to the obesity control, suggesting that HAS and EHAS can improve liver function by protecting the liver cell membrane and reducing the release of GPT, GOT and AKP.
To further understand the molecular mechanism of HAS and EHAS on lipid metabolism regulation, we examined the expression of lipid metabolic genes, liver functional genes and oxidative stress related genes. Significant down-regulations of the relative expression of ACC were observed in the treated groups, which may lead to a reduced opportunity for acetyl-CoA carboxylase to participate in fatty acid synthesis, which as a result reduces adipose tissue formation. A significant reduction in the expression of the SREBP-1 gene in HAS and EHAS groups supported the observation of maintaining cholesterol homeostasis in these groups. Fads1 is a key enzyme responsible for the synthesis of polyunsaturated fatty acids. A significant decrease in relative gene expression was noted in both the HAS group and the EHAS group, which may also contribute to reduced oxidative stress due to the reaction between polyunsaturated fatty acid and ROS productions. LXRα has been regarded as a central regulator of cholesterol homeostasis and lipid and glucose metabolism.46 It was also noted that the expression levels of LXRα in the EHAS group were significantly lower than those in the MC group, suggesting the cholesterol-lowering effect of EHAS, which may be attributed to its promotion of cholesterol conversion into the bile acid by down-regulating the gene expression of LXRα. The expression of Acox1, the lipid-oxidation enzyme, was increased significantly, indicating an intensive lipid oxidation in the rats following the RS administration. The PPARγ gene could be activated when fatty acid is accumulated, and could regulate fatty acid metabolism via up-regulation of downstream genes like ACOX1, and catalyze hydroxy cholesterol synthesis by the regulation of downstream genes and activate LXRα to regulate cellular cholesterol metabolism. The significant reduction of PPARγ expression in the RS treated groups indicated that triglyceride synthesis was efficiently suppressed by both HAS and EHAS groups. Both the intervention groups were found to show a significant inhibition in HMGCR relative expression compared with the MC group, which blocks the conversion of HMG-CoA to mevalonate, thereby reducing cholesterol levels.47 Moreover, our study showed that the expression of liver function related genes (except Gstm2) was significantly increased by HAS and/or EHAS consumption. It can be seen from Fig. 4(c) that HAS was mainly associated with regulation of oxidative stress in the obese rats by down-regulating gene expression of VEGF and GAPDH. EHAS suppressed oxidative stress in the high fat-diet by up-regulating Egfr gene expression and down-regulating Igfbp1 and Pml expression, as well as VEGF and GAPDH.
Taken together, the two types of resistant starch, RS2 and RS4, were observed to exert a positive regulatory effect on high fat diet induced obesity in rats. In contrast, RS4 supplementation of the high fat diet attenuated diet-induced obesity more efficiently. Adding chemical derivatives of acyl groups to high amylose starch changes the structure of the starch (seen in Fig. 1 and 2) and partially restricts the enzymatic hydrolysis of the starch molecule,16 resulting in RS4-type resistant starch. RS4-type resistant starch showed a reduced susceptibility to enzymes in vitro and in vivo compared with the corresponding unmodified starch,48,49 consequently reducing the energy density50 and attenuating the degradation of starch polymers into glucose.16 Moreover, the more efficient effect of RS4-type resistant starch on reducing lipid peroxidation and elevating antioxidant status in our study appeared to be associated with its excellent performance in elevating antioxidant enzymes activity, significantly up-regulating gene expression of Gsta2, Gsta3, Nqo1, and Egfr, and significantly down-regulating gene expression of Igfbp1 and Pml, compared with RS2-type resistant starch. Shimotoyodome et al. reported that the increased oxidation of fatty acids in the liver drained fatty acids from the body, reducing VLDL formation and exerting anti-obesity effects, which may be similar to those of RS4-type resistant starch, contributing to the superior performance of RS4-type resistant starch compared to RS2-type resistant starch.51
5. Conclusion
The present study compares RS2 and RS4 resistant starches through the measurement of their abilities to attenuate oxidative stress induced by a high fat diet. This study found that the administration of both types of resistant starches improved the antioxidant status in obese rats by maintaining antioxidant enzyme activity. Furthermore, the current data also indicate that RS4-type resistant starch seems to be superior to RS2-type resistant starch in terms of efficiently lowering serum glucose, decreasing serum lipid levels, and protecting liver cells by enhancing the hepatic function, which was confirmed by the changes in the expression of oxidative stress related genes. To the best of our knowledge, this is the first study to compare and analyse the oxidative stress regulation between RS2-type and RS4-type resistant starches using a high fat diet induced model.
Acknowledgements
This work was financially supported by the Agricultural Science and Technology Achievements Transformation Fund (2014GB2A100527), the National Key Research and Development Program (2016YFD0400100), the NSFC (no. 31471701), the NSFC (U1501214), the Tianjin Research Program of Application Foundation and Advanced Technology (15JCZDJC34300), and the China–European research collaboration programme (SQ2013ZOA100001).
References
- L. Huang, J. Chen, P. Cao, H. Pan, C. Ding and T. Xiao, Mar. Drugs, 2015, 13(5), 2732–2756 CrossRef CAS PubMed.
- K. G. Alberti and P. Z. Zimmet, Diabetic Med., 1998, 15, 539 CrossRef CAS PubMed.
- N. Z. Baquer, P. Kumar, A. Taha, R. K. Kale, S. M. Cowsik and P. McLean, J. Biosci., 2011, 36(2), 383–396 CrossRef CAS PubMed.
- J. Zhou, L. Chan and S. Zhou, Curr. Med. Chem., 2012, 19(21), 3523–3531 CrossRef CAS PubMed.
- E. Al-Khateeb, S. A. Hamadi, A. A. N. Al-Hakeemi, M. Abu-Taha and N. Al-Rawi, Eur. J. Sci., 2012, 8, 16–24 Search PubMed.
- L. Trasande, C. Cronk, M. Durkin, M. Weiss, D. A. Schoeller, E. A. Gall, J. Hewitt, A. Carrel, P. Landrigan and M. W. Gillman, Environ. Health Perspect., 2009, 117(2), 159 CrossRef PubMed.
- M. Kelaher, S. Paul, H. Lambert, W. Ahmad and G. D. Smith, Ann. Epidemiol., 2008, 18(5), 351–356 CrossRef PubMed.
- P. Codoñer, Nutr., Metab. Cardiovasc. Dis., 2012, 22(3), 237–243 CrossRef PubMed.
- S. Isabella, C. Maria Valeria, E. Daniela, G. Valeria and A. Luciana, Int. J. Mol. Sci., 2013, 14(5), 10497–10538 CrossRef PubMed.
- W. Jian, S. Dan, Z. M. Qi, Z. H. Yan, Q. W. Ping and P. Nan, Pediatr. Obes., 2014, 9(1), 43–52 CrossRef PubMed.
- C. F. Pilar, V. B. Victoria, A. C. Angela and A. I. Eulalia, Transl. Res., 2011, 158(6), 369–384 CrossRef PubMed.
- S. Furukawa, T. Fujita, M. Shimabukuro, M. Iwaki, Y. Yamada, Y. Nakajima, O. Nakayama, M. Makishima, M. Matsuda and I. Shimomura, J. Clin. Invest., 2004, 114(12), 1752–1761 CAS.
- M. M. J. Champ, J. AOAC Int., 2004, 87(3), 749–755 CAS.
- E. E. J. G. Aller, A. Itziar, A. Arne, M. J. Alfredo and M. A. V. Baak, Nutrients, 2011, 3(3), 341–369 CrossRef CAS PubMed.
- G. S. Ranhotra, J. A. Gelroth and B. K. Glaser, Cereal Chem., 1996, 73(2), 176–178 CAS.
- D. F. Birt, T. Boylston, S. Hendrich, J. L. Jane, J. Hollis, L. Li, J. McClelland, S. Moore, G. J. Phillips, M. Rowling, K. Schalinske, M. P. Scott and E. M. Whitley, Adv. Nutr., 2013, 4(6), 587–601 CrossRef CAS PubMed.
- R. N. Tharanathan and S. Mahadevamma, Trends Food Sci. Technol., 2003, 14, 507–518 CrossRef CAS.
- A. Sharma, B. S. Yadav and Ritika, Food Rev. Int., 2008, 24, 193–234 CrossRef CAS.
- H. W. Lopez, M. A. Levrat-Verny, C. Coudray, C. Besson, V. Krespine, A. Messager, C. Demigné and C. Rémésy, J. Nutr., 2011, 131(4), 1283–1289 Search PubMed.
- H. Younes, M. A. Levrat, C. Demige and C. Remesy, Lipids, 1995, 30, 847–853 CrossRef CAS.
- S. Rashmi and A. Urooj, Int. J. Food Sci. Nutr., 2003, 54(1), 27–36 CrossRef CAS PubMed.
- F. Brouns, B. Kettlitz and E. Arrigoni, Trends Food Sci. Technol., 2002, 13(8), 251–261 CrossRef CAS.
- D. L. Topping and P. M. Clifton, Physiol. Rev., 2001, 81(3), 1031–1064 CAS.
- M. Elomaa, T. Asplund, P. Soininen, R. Laatikainen, S. Peltonen, S. Hyvärinen and A. Urtti, Carbohydr. Polym., 2004, 57(3), 261–267 CrossRef CAS.
- B. Cui, Y. M. Lu, C. P. Tan, G. Q. Wang and G. H. Li, Food Hydrocolloids, 2014, 35(1), 576–582 CrossRef CAS.
- A. Chaidedgumjorn, H. Toyoda, E. R. Woo, K. B. Lee, Y. S. Kim, T. Toida and T. Imanari, Carbohydr. Res., 2002, 337, 925–933 CrossRef CAS PubMed.
- J. G. Bieri, J. Nutr., 1980, 110(8), 1726 Search PubMed.
- K. M. Wilbur, Arch. Biochem., 1949, 24, 305–313 CAS.
- F. Tietez, Anal. Biochem., 1969, 27, 502–522 CrossRef.
- K. J. Livak and T. D. Schmittgen, Methods, 2001, 25(4), 402–408 CrossRef CAS PubMed.
- M. Fried, V. Hainer, A. Basdevant, H. Buchwald, M. Deitel, N. Finer, J. W. M. Greve, F. Horber, E. Mathus-Vliegen, N. Scopinaro, R. Steffen, C. Tsigos, R. Weiner and K. Widhalm, Obes. Facts, 2008, 1(1), 52–59 CrossRef PubMed.
- M. G. Andreassi, R. Barale, P. Iozzo and E. Picano, Mutagenesis, 2011, 26(1), 77–83 CrossRef CAS PubMed.
- D. Marović, Srp. Arh. Celok. Lek., 2008, 136(3–4), 122–125 CrossRef.
- C. J. Lavie, P. A. Mcauley, T. S. Church, R. V. Milani and S. N. Blair, J. Am. Coll. Cardiol., 2014, 63(14), 1345–1354 CrossRef PubMed.
- S. M. Grundy, Endocrine, 2002, 13(2), 155–165 CrossRef.
- S. M. Grundy, J. Clin. Endocrinol. Metab., 2004, 89(6), 2595–2600 CrossRef CAS PubMed.
- Z. K. Zhou, W. Fang, X. C. Ren, Y. Wang and C. Blanchard, Int. J. Biol. Macromol., 2015, 75, 316–321 CrossRef CAS PubMed.
- B. P. Yu, Physiol. Rev., 1994, 74(1), 139–162 CAS.
- H. K. Vincent and A. G. Taylor, Int. J. Obes., 2006, 30(3), 400–418 CrossRef CAS PubMed.
- K. Lahbib, I. Aouani, J. F. Cavalier and S. Touil, Chem. Biol. Drug Des., 2015, 86(3), 291–301 CAS.
- M. P. Mattson, Exp. Gerontol., 2009, 44(10), 625–633 CrossRef CAS PubMed.
- S. A. Noeman, H. E. Hamooda and A. A. Baalash, Diabetol. Metab. Syndr., 2011, 3(1), 1–8, DOI:10.1186/1758-5996-3-17.
- D. A. Kieffer, B. D. Piccolo, N. D. Vaziri, S. Liu, W. L. Lau, M. Khazaeli, S. Nazertehrani, M. E. Moore, M. L. Marco, R. J. Martin and S. H. Adams, Am. J. Physiol.: Renal Physiol., 2016, 310(9), 857–871 CrossRef PubMed.
- N. D. Vaziri, S. M. Liu, L. L. Wei, M. Khazaeli, S. Nazertehrani, S. H. Farzaneh, D. A. Kieffer, S. H. Adams and R. J. Martin, PLoS One, 2014, 9(12), 751–763 Search PubMed.
- F. S. Miao, W. Q. Yu, Y. G. Wang, M. J. Wang, X. Y. Liu and F. L. Li, Afr. J. Pharm. Pharmacol., 2010, 4, 178–183 CAS.
- M. V. Cannon, H. H. Silljé, J. W. Sijbesma, M. A. Khan, K. R. Steffensen, W. H. van Gilst and R. A. de Boer, Diabetologia, 2016, 59(3), 634–643 CrossRef CAS PubMed.
- J. L. Figarola, P. Singhal, S. Rahbar, B. G. Gugiu, S. Awasthi and S. S. Singhal, PLoS One, 2013, 8(12), 1524–1528 Search PubMed.
- M. Wootton and M. A. Chaudhry, Starch/Staerke, 1979, 31(7), 224–228 CrossRef CAS.
- K. Ebihara, Nipponyo Shokuryo Gakkaishi, 1992, 45, 551–553 CrossRef CAS.
- J. Zhou, R. J. Martin, R. T. Tulley, A. M. Raggio, K. L. McCutcheon, L. Shen, S. C. Danna, S. Tripathy, M. Hegsted and M. J. Keenan, Am. J. Physiol.: Endocrinol. Metab., 2008, 295(5), 1160–1166 CrossRef PubMed.
- A. Shimotoyodome, J. Suzuki, D. Fukuoka, I. Tokimitsu and T. Hase, Am. J. Physiol.: Endocrinol. Metab., 2010, 298(3), 652–662 CrossRef PubMed.
Footnote |
† Electronic supplementary information (ESI) available. See DOI: 10.1039/c6fo01225f |
|
This journal is © The Royal Society of Chemistry 2017 |