Enzyme-immobilized clay nanotube–chitosan membranes with sustainable biocatalytic activities
Received
31st October 2016
, Accepted 22nd November 2016
First published on 22nd November 2016
Abstract
We have developed a simple and effective strategy to prepare an enzymatic membrane by the admixing of a halloysite clay nanotube–lipase complex and a chitosan solution. The loading of 3 wt% lipase into the lumen of halloysite clay nanotubes was enhanced with an electrostatic interaction. 10 wt% of lipase–nanotubes were dispersed in an aqueous chitosan solution and cast as a coating. The enzymatic chitosan–halloysite membranes displayed increased pH, temperature stability and long-term usability for the catalytic decomposition of lipids: 90% of the initial activity was preserved at 80 °C, 70% of the functionality remained at the high pH of 9, and 85% of the activity was sustained after ten continuous cycles of use. The placement of the second antimicrobial enzyme–lysozyme at the outer surface of the clay nanotubes allowed for a synergistic increase in the activity of the composite antibacterial formulation. The lipase/lysozyme-co-immobilized clay nanotube–chitosan membranes are promising in anti-biofouling applications.
Introduction
Enzymes are biomacromolecules that catalyze chemical transformations with excellent efficiency and selectivity.1,2 Carrier-based immobilization of enzymes has been shown to be an effective strategy to improve the robustness and reusability of enzymes, resulting in enhanced overall catalytic conversion.3–5 Polymeric membranes are good supports for enzyme immobilization, bioreactors, water treatment, and antifouling coatings.6–8 Covalent grafting is often used to immobilize enzymes on membrane surfaces.9–11 Generally, this strategy inevitably requires relatively complex manipulation and covalent binding which often disturbs the conformation of enzyme molecules. One-step mixing of enzymes with a polymer solution followed by casting composite membranes is a simple, economical and scalable strategy for producing bioreactors.12–14 However, a large part of enzymes can leak due to the weak intermolecular interactions with the polymeric matrix, which causes long-term instability of the enzymatic membranes.12,14 Therefore, using enzyme containing capsules bound into the polymeric matrix is promising for the fabrication of robust biocatalytic membranes.
Biocompatible halloysite clay nanotubes are a promising carrier for various guest molecules, e.g. enzymes and drugs, due to their unique hollow cylindrical structure and abundant availability.15–22 Halloysite has a tubular structure, with a length of 500–800 nm, an outer diameter of 50–60 nm, and a lumen diameter of 12–15 nm.23 Globular enzymes with a typical diameter of 3–6 nm can be loaded into the lumen of these tubes and slowly diffused out. Halloysite nanotubes have a positively charged inner lumen (zeta-potential ξ = +24 mV) which consists of aluminum hydroxide and a negatively charged silicon dioxide outer surface (ξ = −35 mV).23–25 Therefore, the selective immobilization of charged macromolecules on the tubes' inside/outside surfaces can be realized. Negatively charged enzymes will be attracted into the lumen and positively charged enzymes will be adsorbed on the tubes' outer surface.15,24–27
Chitosan, a natural biopolymer mainly composed of β (1-4) linked glucosamine units, is a desirable material for the membranes due to its physiological inertness, biocompatibility, hydrophilicity, good affinity to proteins, and high mechanical strength.28,29 Chitosan has good film-forming capabilities due to its solubility in organic acids.30 Cross-linkers are usually used for covalent binding of enzymes on chitosan carriers,30 but this method involves organic solvents and toxic reagents, and was not used in our membranes, enhancing enzyme immobilization with tubular containers.
We have developed an effective method to fabricate enzymatic membranes using easily accessible materials, halloysite clay nanotubes, chitosan and enzymes with antimicrobial activities – lipase and lysozyme. First, lipase loading into the lumen of the clay nanotube was optimized, and then the resulting enzyme–nanotube complex was mixed with chitosan to fabricate the enzymatic membrane (Scheme 1). The obtained clay nanotube/enzyme hybrid chitosan membranes showed good catalytic lipid decomposition, stability and reusability. Secondly, the cationic lysozyme was adsorbed onto the halloysite tubes' outer surface, which may synergistically increase the antifouling capability of the dual enzyme composite.
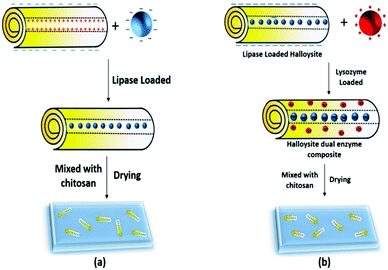 |
| Scheme 1 (a) Loading of anionic lipase into the tube lumen and membrane preparation, and (b) dual enzyme loading; anionic lipase goes into the positive lumen and cationic lysozyme goes onto the surface of the negative tubes. | |
Materials and methods
Halloysite clay nanotubes were supplied by Applied Minerals Inc., USA. Lipase and lysozyme were purchased from Sigma-Aldrich and used without further processing. Transmission electron microscopy (TEM) was performed using JEOL JEM-1011 at 100 kV. Scanning electron microscopy (SEM) was performed using S-4800 (HITACHI, Japan) with an accelerating voltage of 10 kV. UV-visible results were obtained using a Shimadzu UV-2600 spectrophotometer.
1. Enzyme loading and biocatalytic activity
The selected enzymes were loaded into halloysite (HNT): HNT/lysozyme (5
:
1) and HNT/lipase (2
:
1) by dissolving the enzyme in phosphate buffer and dispersing the halloysite in an appropriate ratio. The dispersion was placed in 3 cycles of vacuum for 20 minutes. The sample was washed thrice and the pellet obtained after centrifugation was re-dispersed and sonicated. The second enzyme was added to the dispersion in an appropriate ratio and the vacuum loading procedure was repeated. The sample was washed thrice with water, dried and powdered. Thermogravimetric analysis (TGA DuPont 1090B Thermal Analyzer) was also used for protein loading estimation.
The amount of immobilized enzyme on the halloysite clay nanotubes was determined by measuring the initial and final concentrations of protein in the enzyme and washing solutions using the micro bicinchoninic acid (Micro BCA) assay (Pierce Biotechnology, Rockford, IL). A standard calibration curve was constructed by using bovine serum albumin (BSA).
The enzymatic activity of lipase was studied as follows: 20 μL of hexadecanoic acid, 4-nitrophenyl ester (0.5 M) dissolved in acetone was added to the phosphate solution (100 mM, pH 7) containing halloysite clay nanotube/lipase. The reaction system was incubated at 40 °C for 30 min under shaking at 50 rpms. Afterwards, the absorbance of the supernatant was measured at 410 nm. One unit of the lipase was defined as the amount of enzyme required to liberate 1 μmoL p-nitrophenol per minute.
The antibacterial activities of lysozyme and the dual enzyme composites were determined using the bacteria Micrococcus lysodeikticus.32 A 0.01% (w/v) dispersion of the bacteria was prepared in phosphate buffer saline to obtain an initial absorption of 0.6–0.7 at 450 nm. 1 mL of the bacterial dispersion was added to a cuvette and its absorbance (A450) was measured for 60 seconds with readings taken every 10 s using a UV-Vis spectrophotometer. 50 μL of 1 mg mL−1 of halloysite–lysozyme (HNT–L) and halloysite–dual-enzyme composite dispersions was added to the bacteria and the kinetic measurement was continued for 600 s. Lysozyme activity was expressed as the rate of reduction in the absorbance of the bacteria.
2. Preparation of the halloysite clay nanotube/enzyme/chitosan membranes
One gram of chitosan was dissolved in 100 mL of acetic acid solution (0.8% w/v). Halloysite nanotube/lipase was mixed with the above chitosan solution for the formation of the halloysite–chitosan composite with a component ratio of 1
:
10, and the composite membrane contained 10 wt% of halloysite. Then 1 mL of the mixture was poured into a Teflon dish with a bottom diameter of 36 mm. The enzymatic chitosan was dried at room temperature for 7 days to form halloysite clay nanotube/enzyme/chitosan membranes. A dilute NaOH solution was used to neutralize the membrane. The membrane could be peeled off from the dish using forceps. To determine the amount of enzymes released from these membranes, the membranes were immersed in a phosphate solution (100 mM, pH 7) for 6 hours and the amount of enzymes in the solution was measured using a micro bicinchoninic acid (Micro BCA) assay (Pierce Biotechnology, Rockford, IL).
The catalytic activity of the nanotube/enzyme/chitosan membrane was determined via the hydrolysis of hexadecanoic acid, 4-nitrophenyl ester. In a typical process, the enzymatic membranes were cut into 1 cm2 squares and dipped into 2 mL of phosphate solution (100 mM, pH 7). Then 20 μL of hexadecanoic acid, 4-nitrophenyl ester (0.5 M) dissolved in acetone was added to the above buffer. The reaction system was incubated at 40 °C for 10 min under shaking at 50 rpms. Afterwards, the absorbance of the supernatant was measured at 410 nm. One unit of the WPU-L film was defined as the dimension of the membranes required to liberate 1 μmoL p-nitrophenol per minute.
3. Effects of pH and temperature on the biocatalytic activity
For pH stability, the halloysite clay nanotube/lipase, the halloysite clay nanotube/lipase/chitosan membrane, or free lipase was added to the appropriate buffer. The buffers used were 100 mM citrate for pH 3–6, 100 mM phosphate buffer for pH 7, and 100 mM carbonate buffer for pH 10. After incubating for 15 min, the enzyme's residual activity was measured using the relevant method presented in the Materials and methods section. For temperature stability, the samples were added to a phosphate solution (100 mM, pH 7), and then incubated at the desired temperature for 15 min, cooled to room temperature, and the residual activity was measured using the appropriate technique for the enzyme.
Results and discussion
1. Encapsulation of enzymes in the halloysite clay nanotubes
Lipase, widely used in catalytic conversion and fouling elimination, was used for immobilization into the clay nanotubes and it has an isoelectric point at pH 6.0, with a negative charge in phosphate buffer (pH 7.0). Therefore, lipase can be electrostatically driven into the positively charged lumen of halloysite clay nanotubes. The structures of halloysite clay nanotubes after immobilization of lipase are shown in Fig. 1.
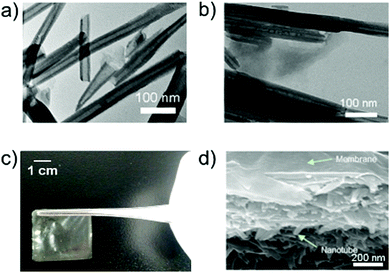 |
| Fig. 1 (a) TEM image of the halloysite clay nanotubes. (b) Halloysite tubes loaded with lipase are visible inside the tubes as darker spots. (c) Optical image of the clay nanotube–chitosan hybrid membrane. (d) SEM image of a section of the halloysite clay nanotube/lipase/chitosan hybrid membrane. | |
The loading of enzymes did not change the morphology of the halloysite clay nanotubes. In the BCA analysis, the content of lipase loaded into the halloysite clay nanotubes increases with an increase in the initial content of lipase, and reaches a maximum loading capability of ∼3 wt% (Fig. 2). The samples were carefully washed after enzyme loading, so we assume that most of the adsorbed enzymes are located in the inner lumen, as shown in Fig. 1b. The corresponding catalytic activity of halloysite/lipase also increases with the increase in the loaded enzyme (Fig. 2). These observations indicate that the substrate lipid molecules are accessible to the immobilized enzyme and the product can diffuse into the bulk phase from the tubes' openings. Lysozyme loading was higher, 7 wt%, reaching saturation in the same range of enzyme concentration of 2.5–3 wt%. The catalytic activities of both enzymes were increased in proportion to the loading and reached saturation in the same concentration region (Fig. 3).
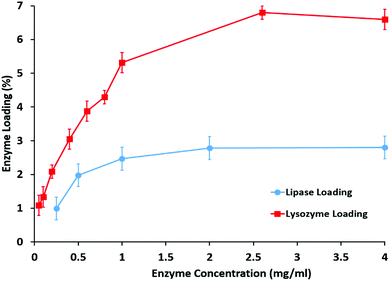 |
| Fig. 2 Adsorption isotherms with halloysite loading efficiency for lipase and lysozyme. | |
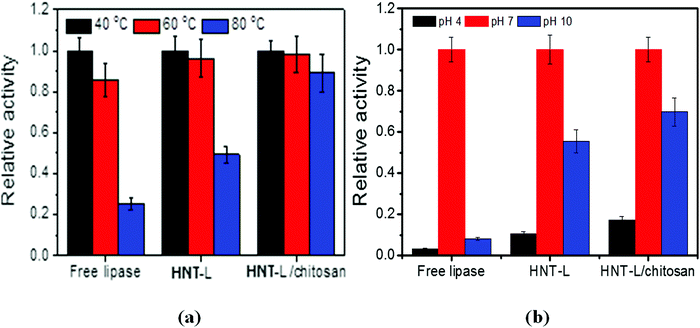 |
| Fig. 3 Effects of temperature (a) and pH (b) on the relative catalytic activity of free lipase, halloysite–lipase (HNT–L) and halloysite–lipase–chitosan (HNT–L–chitosan) formulations. | |
Lysozyme has an isoelectric point at pH 11 and it is positively charged at pH 6–7. This results in a larger amount of adsorbed protein of 7 wt%, which is probably located on the outer negative surface, while less loading of negative proteins occurs inside the tubes. Proteins adsorbed on the halloysite tube have a density of ca. 1.3 g cm−3 and the halloysite density is 2.53 ± 0.03 g cm−3, so the equal protein volume will give a mass twice as small. The ratio of the volume fraction of the protein layer ΔL on the outer tube surface (R) to the total tube volume will be 2ΔL/R, and for a protein monolayer of 5 nm thickness, this gives ca. 16 vol% or 8 wt%. Therefore, the experimental 7 wt% lysozyme coating on halloysite results in a monolayer of proteins adsorbed on the outer surface of the tube. While for lipase, the ratio of the volume fraction of the protein layer on the inner lumen tube (r) surface to the total tube volume will be 2ΔLr/R2 and the monolayer coverage is ca. 6 vol% or 3 wt%. So, the results presented in Fig. 2 for the anionic lipase and cationic lysozyme correspond to the tube coverage of the internal and external proteins, respectively.
2. Stability of the halloysite clay nanotube/lipase (HNT–L)
Thermostability is one of the most important properties for enzyme-based materials in practical applications and below we provide data on its improvement after halloysite immobilization. After immersing the loaded tubes in aqueous Tris-HCl buffer solution, about 20 wt% of lipase was released within 5 h, but the remaining 80 wt% of lipase is efficiently immobilized with an improved stability as compared with the free enzymes in the solution. The thermostability of lipase in the halloysite clay nanotubes was investigated by incubating the samples at 40, 60 and 80 °C for 15 min. At 40 °C, the optimum temperature for lipase catalysis, the free lipase shows a slightly higher catalytic capability than the immobilized one. This indicates that the contact of lipase with the clay nanotubes deteriorates some catalytic activity, possibly due to the disturbance of active site conformation. However, at a higher temperature of 80 °C, the clay nanotube/lipase shows a much higher preserved catalytic activity than the free ones (50 vs. 20%) (Fig. 3a). Such remarkable stability for the nanotube/lipase might be attributed to the incorporation of enzymes within the halloysite lumens, which stabilizes the enzyme conformation preventing unfolding of the protein amino acid chain. In contrast, the free enzymes suffered a rapid deactivation after 80 °C thermal stimulus.
The halloysite clay nanotube/lipase reveals a better preserved activity under acidic conditions, pH 4 (10 vs. 3%), and is spatially well stabilized under alkaline conditions, pH 10 (55 vs. 8% for the free enzymes) (Fig. 3b). The optimum pH values for the free and immobilized enzymes are the same, pH 7. Similar observations were made for the halloysite-immobilized lysozyme.
3. Catalytic capability of the HNT–L–chitosan hybrid membranes
After drying at room temperature, the mixture of HNT–L–chitosan and chitosan solution forms transparent and flat membranes (Fig. 1c). SEM images show that the enzymatic halloysite clay nanotubes are uniformly distributed in the chitosan membranes (Fig. 1d). These results suggest that the halloysite clay nanotubes have good compatibility with the chitosan matrix. The obtained HNT–L–chitosan membranes have the bioactivity to catalyze the hydrolysis of the hexadecanoic acid, 4-nitrophenyl ester, indicating that the enzyme molecules can still contact the substrate even after embedding in the chitosan membranes. The catalytic activity of the hybrid membrane increases with the increase in the amount of halloysite clay nanotube/lipase, and a saturated catalytic activity is obtained when the content of the enzyme in the membranes is 0.15 wt% (Fig. 4). A further increase in HNT–L does not increase the catalytic activity, possibly due to the diffusion limitation of mass transfer at a given surface of the chitosan membrane. In addition, the halloysite nanotube/lipase/chitosan hybrid membranes show further improved temperature and pH stabilities as compared to the non-embedded polymer matrix halloysite nanotube/lipase.
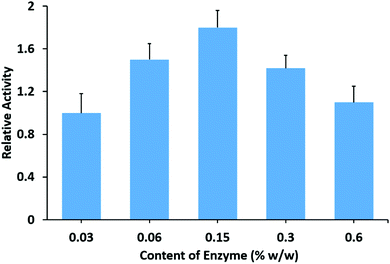 |
| Fig. 4 Relative catalytic activity of the halloysite clay nanotube/lipase/chitosan membranes with different amounts of lipase. | |
The HNT–L–chitosan membranes retained ca. 20 and 80% of their residual activity at pH 4 and 9, and 90% at 80 °C, which is a remarkable improvement in temperature stability (Fig. 3a and b). This higher stability is possibly due to the enhanced retention of the enzyme molecules in the nanotubes after admixing into the chitosan matrix. The immobilization of lipase onto the chitosan membrane did not change the optimum temperature or pH. In the BCA analysis, less than 1 wt% of the protein loaded into the chitosan was detected in the incubation solution, and no activity was observed, indicating that almost all of the lipase was irreversibly immobilized into the chitosan–halloysite membranes. We suggest that the cationic chitosan molecular chain binds to the halloysite and blocks the ends of the tubes, thereby decreasing the enzyme leakage.
4. Reusability of the halloysite clay nanotube/lipase/chitosan hybrid membranes
For consideration of coating applications, reusability and long-term use of the enzymatic membranes would be desirable. Therefore, the reusability of the halloysite clay nanotube/lipase/chitosan hybrid membranes was tested. After the measurement of initial activity, the film was incubated in phosphate buffer for 2 hours and then its activity was tested and this process was repeated. Results of ten cycles of reusability experiments, performed over five consecutive days, are presented here. The hydrolytic activity of the films after ten washes retained ca. 60% of the initial activity of the enzymatic membranes (Fig. 5). Furthermore, no activity was observed in the washed solution, suggesting that there was no loss of lipase from the films and the observed activity resulted from enzymes incorporated into the films. The initial loss in activity of the enzymatic coatings was usually found mainly due to leaching of unfixed enzymes.
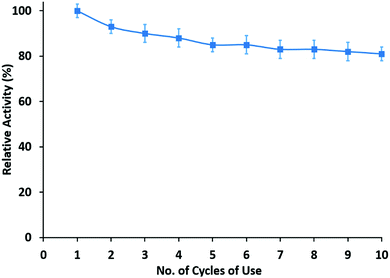 |
| Fig. 5 Reusability of the HNT–L chitosan membrane. | |
In this study, the film activity was almost unchanged in the first five cycles. Halloysite clay nanotube/lipase is efficiently entangled within the polymer matrix, thereby allowing enzymes to be retained and to possess activity after long-term reuse of these materials. This property of retention of the enzymatic activity can be compared to the microgel oral delivery system which contained halloysite loaded with bovine serum albumin (BSA).31 BSA immobilized in a pure microgel system retained only 5% of its activity in a digestive environment (PBS with trypsin), whereas BSA encapsulated halloysite incorporated in microgels retained 82% of its activity.
5. Sequential two enzyme adsorption on the clay nanotubes
Since halloysite has a negatively charged outer surface and a positively charged inner lumen, it can be used as a template for inside/outside loading of two different enzymes depending on their isoelectric points. Lysozyme (pKa = 11) is positively charged at pH 6.5–7 whereas lipase (pKa = 6) is negatively charged. For enhanced antimicrobial functionality we designed a nano-architecture where lysozyme is located on the outer tube surface and lipase in the lumen.
The loading efficiency of individual enzymes and lipase/lysozyme adsorbed at different combinations was determined using TGA, and is presented in Table 1. The loading efficiency of individual enzymes was lower when compared to the sequential enzyme loading, which is in agreement with our hypothesis that first we should load lipase inside the tubes and then load lysozyme outside the tube as defined by the negative–positive charge electrostatic interaction.
Table 1 Loading efficiency of dual enzyme and individual enzymes in/on to the halloysites
Protein charge |
Sample architecture |
Loading, ±0.1 (%) |
+ |
HNT–lysozyme |
7.0 |
− |
HNT–lipase |
3.5 |
± |
HNT–lipase/lysozyme |
6.9 |
Mixed |
HNT–lysozyme + lipase |
6.3 |
The lipase activity was highest in the sample where lipase was loaded next to lysozyme. It is twice the activity of the sample where lipase was loaded prior to lysozyme. This may be due to the difference in availability of the enzymes from the halloysite. The addition of lipase did not essentially change the overall protein loading because the tube-opening to the inside lumen is blocked by lysozyme. Similarly, starting with negative lipase did not increase the loading efficiency.
The antibacterial activity of a single enzyme and dual enzyme nanotube composites was measured as the rate of decrease in bacterial M. lysodeikticus turbidity in their dispersions.32 The rate of activity of the control sample with pristine halloysite was also measured in order to ensure that halloysite does not contribute to the overall turbidity. Samples which had lysozyme loaded halloysite later had the highest rate (−754.5 AU s−1), suggesting that it was adsorbed on the outside making it more available for the substrate. Halloysite lysozyme composites had a lower rate (−252 AU s−1) compared to the dual enzyme composites (−492.8 AU s−1), suggesting that the presence of another enzyme contributes synergistically to the activity.
Conclusions
A robust enzymatic membrane was prepared by casting a mixture of halloysite clay nanotube/lipase/lysozyme and aqueous chitosan. Such nanocomposite chitosan membranes are capable of hydrolysis of lipids without the loss of enzymes. The encapsulation of enzymes within clay nanotubes bound to a chitosan matrix resulted in their enhanced stability and reusability. Chitosan membranes embedded with a nanotube–enzyme complex represent a facile route to developing efficient antimicrobial formulations. Using dual antimicrobial enzyme–lipase and lysozyme with the sequential adsorption on halloysite allowed for doubling of the antimicrobial activity of the formulation as tested for M. Lysodeikticus. This approach may be extended to other functional enzymes and is promising in antifouling applications.
Acknowledgements
We acknowledge financial support from the National Natural Science Foundation of China (Project No. 21522307, 21473208 and 91434103), the Talent Fund of the Recruitment Program of Global Youth Experts, and the Chinese Academy of Sciences (CAS, No. QYZDB-SSW-JSC034). YL acknowledges the support from the Russian Science Foundation (project # 14-19-01045).
References
- A. Schmid, J. S. Dordick, B. Hauer, A. Kiener, M. Wubbolts and B. Witholt, Nature, 2001, 409, 258–268 CrossRef CAS PubMed
.
- T. Bornscheuer, G. W. Huisman, R. J. Kazlauskas, S. Lutz, J. C. Moore and K. Robins, Nature, 2012, 485, 185–194 CrossRef PubMed
.
- H. R. Luckarift, J. C. Spain, R. R. Naik and M. O. Stone, Nat. Biotechnol., 2004, 22, 211–213 CrossRef CAS PubMed
.
- R. A. Sheldon and S. van Pelt, Chem. Soc. Rev., 2013, 42, 6223–6235 RSC
.
- K. Ariga, Q. M. Ji, T. Mori, M. Naito, Y. Yamauchi, H. Abe and J. P. Hill, Chem. Soc. Rev., 2013, 42, 6322–6345 RSC
.
- P. Jochems, Y. Satyawali, L. Diels and W. Dejonghe, Green Chem., 2011, 13, 1609–1623 RSC
.
- N. Cicek, J. P. Franco, M. T. Suidan, V. Urbain and J. Manem, Water Environ. Res., 1999, 71, 64–70 CrossRef CAS
.
- I. Banerjee, R. C. Pangule and R. S. Kane, Adv. Mater., 2011, 23, 690–718 CrossRef CAS PubMed
.
- J. N. Talbert and J. M. Goddard, Colloids Surf., B, 2012, 93, 8–19 CrossRef CAS PubMed
.
- S. Yuan, D. Wan, B. Liang, S. Pehkonen, Y. Ting, K. Neoh and E. Kang, Langmuir, 2011, 27, 2761–2774 CrossRef CAS PubMed
.
- K. Muszanska, H. J. Busscher, A. Herrmann, H. C. van der Mei and W. Norde, Biomaterials, 2011, 32, 6333–6341 CrossRef PubMed
.
- K. Rege, N. R. Raravikar, D. Y. Kim, L. S. Schadler, P. M. Ajayan and J. S. Dordick, Nano Lett., 2003, 3, 829–832 CrossRef CAS
.
- R. C. Pangule, S. J. Brooks, C. Z. Dinu, S. S. Bale, S. L. Salmon, G. Y. Zhu, D. W. Metzger, R. S. Kane and J. S. Dordick, ACS Nano, 2010, 4, 3993–4000 CrossRef CAS PubMed
.
- S. J. Novick and J. S. Dordick, Biomaterials, 2002, 23, 441–448 CrossRef CAS PubMed
.
- J. Tully, R. Yendluri and Y. Lvov, Biomacromolecules, 2016, 17, 615–621 CrossRef CAS PubMed
.
- J. J. Xue, Y. Z. Niu, M. Gong, R. Shi, D. F. Chen, L. Q. Zhang and Y. Lvov, ACS Nano, 2015, 9, 1600–1612 CrossRef CAS PubMed
.
- Y. M. Lvov, M. M. DeVilliers and R. F. Fakhrullin, Expert Opin. Drug Delivery, 2016, 13, 977–986 CrossRef CAS PubMed
.
- D. G. Shchukin, G. B. Sukhorukov, R. R. Price and Y. M. Lvov, Small, 2005, 1, 510–513 CrossRef CAS PubMed
.
- C. Chao, J. D. Liu, J. T. Wang, Y. W. Zhang, B. Zhang, Y. T. Zhang, X. Xiang and R. F. Chen, ACS Appl. Mater. Interfaces, 2013, 5, 10559–10564 CAS
.
- R. Zhai, B. Zhang, Y. Z. Wan, C. C. Li, J. T. Wang and J. D. Liu, Chem. Eng. J., 2013, 214, 304–309 CrossRef CAS
.
- M. Kruchkova, A. Danilushkina, Y. Lvov and R. Fakhrullin, Environ. Sci.: Nano, 2016, 3, 442–452 RSC
.
- M. Dzamukova, E. Naumenko, A. Badrutdinov, Y. Lvov and R. Fakhrullin, Sci. Rep., 2015, 5, 10560 CrossRef CAS PubMed
.
- Y. Lvov, W. Wang, L. Zhang and R. Fakhrullin, Adv. Mater., 2016, 28, 1227–1250 CrossRef CAS PubMed
.
- N. G. Veerabadran, R. R. Price and Y. M. Lvov, Nano, 2007, 2, 115–120 CrossRef CAS
.
- Y. Lvov, A. Aerov and R. Fakhrullin, Adv. Colloid Interface Sci., 2014, 207, 189–198 CrossRef CAS PubMed
.
- D. Tan, P. Yuan, F. Annabi-Bergaya, H. Yu, D. Liu, H. Liu and H. He, Microporous Mesoporous Mater., 2013, 179, 89–98 CrossRef CAS
.
- P. Yuan, D. Tan and F. Annabi-Bergaya, Appl. Clay Sci., 2015, 112, 75–93 CrossRef
.
- M. Kumar, React. Funct. Polym., 2000, 46, 1–27 CrossRef CAS
.
- M. Rinaudo, Prog. Polym. Sci., 2006, 31, 603–632 CrossRef CAS
.
- M. Aider, LWT–Food Sci. Technol., 2010, 43, 837–842 CrossRef CAS
.
- J. Kruif, G. Ledergerber, C. Garofalo, E. Fasler-Kan and M. Kuentz, Eur. J. Pharm. Biopharm., 2016, 101, 90–102 CrossRef PubMed
.
- P. Mörsky, Anal. Biochem., 1983, 128, 77–85 CrossRef
.
|
This journal is © the Owner Societies 2017 |