Atmospheric chemistry of Z- and E-CF3CH
CHCF3†
Received
21st October 2016
, Accepted 27th November 2016
First published on 29th November 2016
Abstract
The atmospheric fates of Z- and E-CF3CH
CHCF3 have been studied, investigating the kinetics and the products of the reactions of the two compounds with Cl atoms, OH radicals, OD radicals, and O3. FTIR smog chamber experiments measured: k(Cl + Z-CF3CH
CHCF3) = (2.59 ± 0.47) × 10−11, k(Cl + E-CF3CH
CHCF3) = (1.36 ± 0.27) × 10−11, k(OH + Z-CF3CH
CHCF3) = (4.21 ± 0.62) × 10−13, k(OH + E-CF3CH
CHCF3) = (1.72 ± 0.42) × 10−13, k(OD + Z-CF3CH
CHCF3) = (6.94 ± 1.25) × 10−13, k(OD + E-CF3CH
CHCF3) = (5.61 ± 0.98) × 10−13, k(O3 + Z-CF3CH
CHCF3) = (6.25 ± 0.70) × 10−22, and k(O3 + E-CF3CH
CHCF3) = (4.14 ± 0.42) × 10−22 cm3 molecule−1 s−1 in 700 Torr of air/N2/O2 diluents at 296 ± 2 K. E-CF3CH
CHCF3 reacts with Cl atoms to give CF3CHClC(O)CF3 in a yield indistinguishable from 100%. Z-CF3CH
CHCF3 reacts with Cl atoms to give (95 ± 10)% CF3CHClC(O)CF3 and (7 ± 1)% E-CF3CH
CHCF3. CF3CHClC(O)CF3 reacts with Cl atoms to give the secondary product CF3C(O)Cl in a yield indistinguishable from 100%, with the observed co-products C(O)F2 and CF3O3CF3. The main atmospheric fate for Z- and E-CF3CH
CHCF3 is reaction with OH radicals. The atmospheric lifetimes of Z- and E-CF3CH
CHCF3 are estimated as 27 and 67 days, respectively. IR absorption cross sections are reported and the global warming potentials (GWPs) of Z- and E-CF3CH
CHCF3 for the 100 year time horizon are calculated to be GWP100 = 2 and 7, respectively. This study provides a comprehensive description of the atmospheric fate and impact of Z- and E-CF3CH
CHCF3.
1. Introduction
Chlorofluorocarbons (CFCs) are well-known for their ability to destroy stratospheric ozone and their potency as greenhouse gases.1–3 They have had several uses, for instance as refrigerants, solvents, in foam blowing and in electronic cleaning.4 Having recognized their environmental and atmospheric impacts they were phased out and replaced by hydrochlorofluorocarbons (HCFCs) and hydrofluorocarbons (HFCs). Generally, the HCFCs and HFCs are more environmentally benign in that the HCFCs and HFCs have shorter atmospheric lifetimes than the CFCs, and that the HFCs do not contain chlorine substituents. However, they are both still long-lived greenhouse gases.
Hydrofluoroolefins (HFOs) constitutes a recent class of alternative replacement compounds to the CFCs, HCFCs, and HFCs. They are more reactive in the atmosphere and thereby have a smaller impact on the environment. It is important to know the fate of these compounds before they enter large-scale production and are potentially released to the environment. Z- and E-CF3CH
CHCF3 (1,1,1,4,4,4-hexafluoro-2-butene, HFO-1336mzzm) belong to this class of proposed HFOs. CF3CH
CHCF3 has been proposed to be used for foam blowing with an improvement of the energy efficiency of the process and the Z-isomer has been shown to have beneficial properties as a substitute for CF3CH2CHF2 (1,1,1,3,3-pentafluoropropane, HFC-245fa) as a refrigerant in organic Rankine cycles.5,6 The photochemical reactor setup at the Copenhagen Center for Atmospheric Research (CCAR) was used to study the atmospheric chemistry of the Z- and E-isomers of CF3CH
CHCF3. The reactions of the two isomers with Cl atoms, OH radicals, OD radicals, and O3 were studied, investigating the kinetics and the products of the reactions with Cl atoms in order to assess the fates of the compounds in the atmosphere. Only one previous study of the Z-isomer by Baasandorj et al.7 exists in the literature. OH radicals, and to a lesser degree, Cl atoms, and O3 are atmospheric oxidants that initiates the atmospheric removal of Z- and E-CF3CH
CHCF3. The kinetics of the reaction of Z-CF3CH
CHCF3 with OD radicals was also investigated in the aforementioned study by Baasandorj et al., so OD radical experiments have been included here to be able to compare the present study to their work.7 We present here the first determination of the Cl atom and O3 initiated chemistry of Z-CF3CH
CHCF3 and the first determination of the atmospheric chemistry of E-CF3CH
CHCF3. The findings from the present study are discussed with respect to the atmospheric chemistry of HFOs.
2. Methodology
2.1 Experimental methods
The CCAR photoreactor is a 101.4 L quartz reactor surrounded by 8 UVA (Osram Eversun L100/79 with the main emission peak at 368 nm) or UVB (Waldmann F85/100 UV6, with a wavelength range of 280–360 nm) lamps, and 16 UVC lamps that are used to initiate the experiments. The reactor is interfaced with a Bruker IFS 66 v/s FTIR spectrometer.8 All spectra were obtained using 32 co-added interferograms with a spectral resolution of 0.25 cm−1 and optical pathlengths of 45.10, 52.67, and 55.55 meters. All experiments were performed at 296 ± 2 K and at a total pressure of 700 Torr air/N2/O2 diluent. The experiments were performed with Cl atoms, OH radicals, OD radicals or O3. Cl atoms were produced by photolysis of Cl2: | Cl2 + hν(UVA or UVB) → 2Cl | (1) |
OH radicals were produced by the photolysis of (CH3)2CHONO (isopropyl nitrite) or CH3ONO (methyl nitrite) followed by reaction with O2 forming HO2: | (CH3)2CHONO + hν(UVC) → (CH3)2CHO˙ + NO | (2) |
| (CH3)2CHO˙ + O2 → (CH3)2CO + HO2 | (3) |
| CH3ONO + hν(UVC) → CH3O˙ + NO | (4) |
| CH3O˙ + O2 → H2CO + HO2 | (5) |
The HO2 formed from (CH3)2CHONO or CH3ONO reacts with NO to give OH radicals:OD radicals were produced by photolysis of CD3ONO (deuterated methyl nitrite): | CD3ONO + hν(UVC) → CD3O + NO | (7) |
| CD3O + O2 → CD2O + DO2 | (8) |
O3 was produced using a commercial ozone generator from O3-Technology (Dielectric barrier discharge; model AC-20). The O3 was pre-concentrated on a silica gel trap to reduce the amount of O2 introduced to the chamber.
The relative rate method is a well-known method for measuring rate coefficients of gas phase reactions. The reactions of the reactants (Z- and E-CF3CH
CHCF3) with the oxidants are measured relative to the reactions of reference compounds with the oxidants:
| Cl/OH/OD + Reactant → Products | (10) |
| Cl/OH/OD + Reference → Products | (11) |
Plotting the loss of reactant
versus the loss of reference the following equation is used:
|  | (I) |
where [Reactant]
t0, [Reactant]
t, [Reference]
t0 and [Reference]
t are the concentrations of the reactant and the reference at times
t0 and
t.
kReactant and
kReference are the rate coefficients of the reactant and the reference reactions, respectively. The slope of the fit of the experimental data to
eqn (I) provides the rate coefficient ratio
kReactant/
kReference. For the experiments with Cl atoms C
2H
2, C
2H
4, C
2H
6, and CH
3C(O)CH
3 were used as references. For the experiments with OH radicals C
3H
8 and C
2H
6 were used as references. For the experiments with OD radicals C
2H
6 and
n-C
4H
10 were used as references.
An absolute rate method was used in the O3 kinetic experiments. Here the loss of the reactant is monitored over time with an excess of O3:
|  | (II) |
The pseudo first order rate coefficients (
kpseudo
1st) obtained from the individual experiments are plotted against the varying O
3 concentrations giving the rate coefficient
k12 of the reaction as the slope of the line fitted to the data:
| O3 + Reactant → Products | (12) |
All reagents except CD3ONO, CH3ONO, and (CH3)2CHONO were obtained from commercial sources. Z- and E-CF3CH
CHCF3 were produced by SynQuest Laboratories at quoted purities of ≤100%. Z-CF3CH
CHCF3 was supplied to us by Honeywell. Ultrapure N2 (≥99.999%), ultrapure O2 (≥99.995%), synthetic air, Z-CF3CH
CHCF3, and E-CF3CH
CHCF3 were used as received. The Z-CF3CH
CHCF3 sample was devoid of any impurities as analyzed by FTIR. The sample of E-CF3CH
CHCF3 contained an impurity of 1.8% Z-CF3CH
CHCF3, which was taken into account in the analysis of the IR spectra. CD3ONO, CH3ONO, and (CH3)2CHONO were produced by the dropwise addition of cold H2SO4 (sulfuric acid) to a mixture of NaNO2 (sodium nitrite) and CD3OH (deuterated methanol), CH3OH (methanol) or (CH3)2CHOH (isopropanol), respectively. The produced nitrites were trapped using either an ice bath or an isopropanol/dry ice bath. They were stored cold and in the dark. CD3ONO, CH3ONO, and (CH3)2CHONO were subjected to freeze–pump–thaw cycling before use. The CD3ONO, CH3ONO, and (CH3)2CHONO samples were devoid of impurities as analyzed by FTIR.
To test for unwanted loss of reagents due to photolysis, dark reactions and heterogeneous reactions, control experiments were performed to investigate these processes. Samples of Z- and E-CF3CH
CHCF3 in the chamber were irradiated with UV with no observable loss. Reactant/product mixtures obtained after UV irradiation were allowed to stand in the dark for 30 minutes. No loss or changes were observed, indicating that loss processes due to dark reactions or heterogeneous reactions are not a complication in these experiments. Unless otherwise stated, the quoted uncertainties are two standard deviations from the least-squares fits and our estimated uncertainty of the analysis (typically ±1% of initial concentrations).
2.2 Computational methods
The calculations were carried out with a fourth generation composite method referred to as G4MP29 with the GAUSSIAN 09, suite of programs.10 G4MP2 theory is approximating a large basis set CCSD(T) single point calculation on a B3LYP/6-31G(2df,p) geometry and is incorporating a so-called higher level correction that is derived by a fit to the experimental values in the G3/05 test set with 454 experimental values.11 The average absolute derivation from the experimental test set values is 1.04 kcal mol−1, which places the G4MP2 results well within chemical accuracy of 10 kJ mol−1. The transition structures for the reactions reported in this work have been confirmed in each case by the calculation of vibrational frequencies (one imaginary frequency) and an intrinsic reaction coordinate analysis. Relative free energies stated within the text correspond to G4MP2 values at 298.15 K. The calculated total energies are available as ESI† (Table S1), which also includes the B3LYP/6-31G(2df,p) optimized geometries in the form of GAUSSIAN archive entries (Table S2, ESI†).
The energies of deuterated species were evaluated using the Freq=readiso keyword with an input from the B3LYP/6-31G(2df,p) frequency calculations. The frequencies were also scaled by 0.9854 as for the G4MP2 calculations. The free energy contributions were obtained in this manner together with the relevant partition functions for the evaluation of relative rate coefficients.
Calculations of theoretical IR spectra used in the product studies were performed with the GAUSSIAN 09, suite of programs,12 using B3LYP/6-31+G(d,p) and ωB97XD/cc-pVTZ frequency calculations. Optimized geometries and IR spectra can be found in the ESI† (Tables S3–S11 and Fig. S1–S9).
3. Results and discussion
3.1 Relative rate study of Z- and E-CF3CH
CHCF3 + Cl
The rates of reactions (13) and (14) were measured relative to reactions (15–17) and (15), (17), and (18), respectively. The initial reaction mixtures were 1.56–2.61 mTorr Z- or E-CF3CH
CHCF3, 72.1–83.3 mTorr Cl2, and 10.4–10.5 mTorr C2H2, 5.21 mTorr C2H4, 7.82 mTorr C2H6 or 10.0–10.4 mTorr CH3C(O)CH3 in 700 Torr air. The mixtures were subjected to a total of 25–76 seconds of UV irradiation. | Cl + Z-CF3CH CHCF3 → CF3CHClCHCF3 | (13) |
| Cl + E-CF3CH CHCF3 → CF3CHClCHCF3 | (14) |
| Cl + CH3C(O)CH3 → Products | (17) |
Fig. 1 shows the loss of Z- and E-CF3CH
CHCF3versus the loss of the reference compounds. Linear least squares analyses of the data for k13 in Fig. 1 gives the rate coefficient ratios k13/k15 = 0.56 ± 0.06, k13/k16 = 0.28 ± 0.03, and k13/k17 = 11.0 ± 1.21. Using k15 = (5.07 ± 0.34) × 10−11,13k16 = (9.29 ± 0.51) × 10−11,13 and k17 = (2.10 ± 0.15) × 10−12
14 gives k13 = (2.85 ± 0.29) × 10−11, (2.61 ± 0.26) × 10−11, and (2.31 ± 0.25) × 10−11 cm3 molecule−1 s−1, respectively. The values for k13 are identical within the ranges of uncertainty. Using a similar approach three rate coefficient values for k14 were obtained. A summary of all the rate coefficient ratios and individual rate coefficient determinations in this work is shown in Table 1. The three values for k14 are also identical within the ranges of uncertainty (see Table 1). We choose to quote final values for k13 and k14 as the averages of the three determinations with uncertainties that encompass the extremes of the individual determinations. Hence, k13 = (2.59 ± 0.47) × 10−11 and k14 = (1.36 ± 0.27) × 10−11 cm3 molecule−1 s−1.
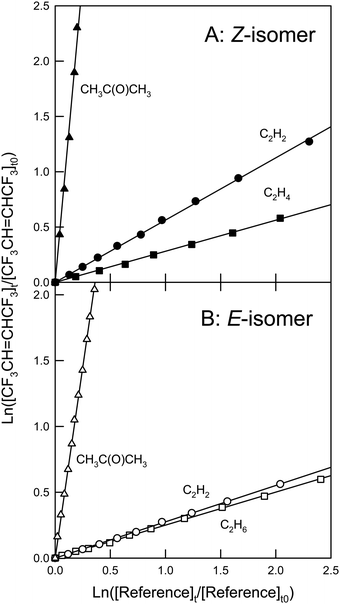 |
| Fig. 1 Panel A: Loss of Z-CF3CH CHCF3versus the loss of C2H2 (circles), C2H4 (squares), and CH3C(O)CH3 (triangles) in the presence of Cl atoms. Panel B: Loss of E-CF3CH CHCF3versus the loss of C2H2 (circles), C2H6 (squares), and CH3C(O)CH3 (triangles) in the presence of Cl atoms. | |
Table 1 Rate coefficient ratios, reference rate coefficient values, and the individual determinations of the rate coefficients of the reactions of Z-CF3CH
CHCF3 (Z) and E-CF3CH
CHCF3 (E) with Cl atoms, OH radicals, and OD radicals
Reaction |
Reference |
k
Reactant/kReference |
k
Reference (cm3 molecule−1 s−1) |
k
Reactant (cm3 molecule−1 s−1) |
Wallington et al.13
Atkinson et al.14
Grenier.36
Paraskevopoulos and Nip.37
|
Z + Cl |
C2H2 |
0.56 ± 0.06 |
(5.07 ± 0.34) × 10−11 a |
(2.85 ± 0.29) × 10−11 |
Z + Cl |
C2H4 |
0.28 ± 0.03 |
(9.29 ± 0.51) × 10−11 a |
(2.61 ± 0.26) × 10−11 |
Z + Cl |
CH3C(O)CH3 |
11.0 ± 1.21 |
(2.1 ± 0.15) × 10−12 b |
(2.31 ± 0.25) × 10−11 |
Z + OH |
C3H8 |
0.38 ± 0.06 |
(1.1 ± 0.08) × 10−12 b |
(4.14 ± 0.40) × 10−13 |
Z + OH |
C2H6 |
1.78 ± 0.18 |
(2.4 ± 0.08) × 10−13 b |
(4.27 ± 0.44) × 10−13 |
Z + OD |
C2H6 |
2.70 ± 0.29 |
(2.74 ± 0.27) × 10−13 c |
(7.39 ± 0.80) × 10−13 |
Z + OD |
n-C4H10 |
0.23 ± 0.03 |
(2.76 ± 0.22) × 10−12 d |
(6.49 ± 0.75) × 10−13 |
|
E + Cl |
C2H2 |
0.28 ± 0.03 |
(5.07 ± 0.34) × 10−11 a |
(1.40 ± 0.14) × 10−11 |
E + Cl |
C2H6 |
0.25 ± 0.03 |
(5.9 ± 0.06) × 10−11 b |
(1.48 ± 0.15) × 10−11 |
E + Cl |
CH3C(O)CH3 |
5.80 ± 0.58 |
(2.1 ± 0.15) × 10−12 b |
(1.22 ± 0.12) × 10−11 |
E + OH |
C3H8 |
0.14 ± 0.02 |
(1.1 ± 0.08) × 10−12 b |
(1.52 ± 0.19) × 10−13 |
E + OH |
C2H6 |
0.80 ± 0.10 |
(2.4 ± 0.08) × 10−13 b |
(1.91 ± 0.23) × 10−13 |
E + OD |
C2H6 |
2.05 ± 0.26 |
(2.74 ± 0.27) × 10−13 c |
(5.63 ± 0.71) × 10−13 |
E + OD |
n-C4H10 |
0.20 ± 0.02 |
(2.76 ± 0.22) × 10−12 d |
(5.59 ± 0.68) × 10−13 |
This is the first determination of k13 and k14. The value of k14 is approximately half of k13. The magnitudes of k13 and k14 are consistent with expectations based on the reactivities of similar HFOs such as E-CF3CH
CHF, Z- and E-CF3CF
CHF, and CF3CF
CF2, which have the rate coefficient values (4.64 ± 0.59) × 10−11,15 (4.36 ± 0.48) × 10−11,16 (5.00 ± 0.56) × 10−11,16 and (2.7 ± 0.3) × 10−11
17 cm3 molecule−1 s−1, respectively.
3.2 Relative rate study of Z- and E-CF3CH
CHCF3 + OH
The rate of reaction (19) and (20) were measured relative to reactions (21) and (22). The reaction mixtures consisted of 1.60–1.98 mTorr Z-CF3CH
CHCF3 or 2.08 mTorr E-CF3CH
CHCF3, 7.19–7.40 mTorr C3H8 or 7.30 mTorr C2H6, and 73.0 mTorr (CH3)2CHONO or 157–224 mTorr CH3ONO in 700 Torr of air. The mixtures were subjected to a total of 265–3180 seconds of UV irradiation. | OH + Z-CF3CH CHCF3 → Products | (19) |
| OH + E-CF3CH CHCF3 → Products | (20) |
Fig. 2 shows the loss of Z- and E-CF3CH
CHCF3versus the loss of reference compounds C3H8 and C2H6 in the presence of OH radicals. Rate coefficient ratios obtained from linear least squares analyses of the data in Fig. 2 are shown in Table 1. Using the rate coefficient values of k21 = (1.1 ± 0.08) × 10−12 and k22 = (2.4 ± 0.08) × 10−13 cm3 molecule−1 s−1,14 gives two values for each k19 and k20, which are identical within the ranges of uncertainty (Table 1). We choose to quote final values for k19 and k20 as the average of the two determinations with uncertainties that encompass the extremes of the individual determinations. Hence, k19 = (4.21 ± 0.62) × 10−13 and k20 = (1.72 ± 0.42) × 10−13 cm3 molecule−1 s−1. Baasandorj et al.7 reported a value for k19 of (4.91 ± 0.50) × 10−13 cm3 molecule−1 s−1, which is in reasonable agreement with the value determined here. The value of k20 is approximately 2/5 the size of k19. The values for k19 and k20 determined in the present work are consistent with expectations based on the OH radical reactivities of similar HFOs such as Z- and E-CF3CF
CHF and Z- and E-CF3CH
CHCl, which have rate coefficients of (1.22 ± 0.14) × 10−12,16 (2.15 ± 0.23) × 10−12,16 (8.45 ± 1.52) × 10−13,18 and (3.61 ± 0.37) × 10−13 cm3 molecule−1 s−1,18 respectively.
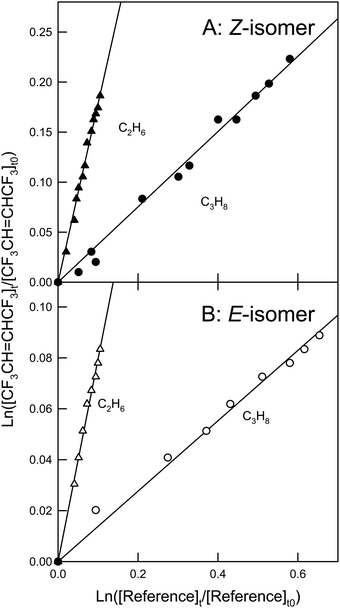 |
| Fig. 2 Panel A: Loss of Z-CF3CH CHCF3versus the loss of C3H8 (circles) and C2H6 (triangles) in the presence of OH radicals. Panel B: Loss of E-CF3CH CHCF3versus the loss of C3H8 (circles) and C2H6 (triangles) in the presence of OH radicals. | |
3.3 Relative rate study of Z- and E-CF3CH
CHCF3 + OD
The rate of reactions (23) and (24) were measured relative to reactions (25) and (26). The initial reaction mixtures consisted of 1.68–2.08 mTorr Z- or E-CF3CH
CHCF3, 6.67–8.34 mTorr C2H6 or 7.30–8.24 mTorr n-C4H10, and 169–230.4 mTorr CD3ONO in 700 Torr air. The mixtures were subject to a total of 860–1080 seconds of UV irradiation. | OD + Z-CF3CH CHCF3 → Products | (23) |
| OD + E-CF3CH CHCF3 → Products | (24) |
| OD + n-C4H10 → Products | (26) |
Fig. 3 shows the loss of Z- and E-CF3CH
CHCF3versus the loss of the two reference compounds C2H6 and n-C4H10 in the presence of OD radicals. Linear regression of the data gives the rate coefficient ratios and values of k23 and k24 shown in Table 1. The two values for k23 are identical within the ranges of uncertainty. The same is the case for k24. We choose to quote final values for k23 and k24 as the averages of the two determinations with uncertainties that encompass the extremes of the individual determinations. Hence, k23 = (6.94 ± 1.25) × 10−13 and k24 = (5.61 ± 0.98) × 10−13 cm3 molecule−1 s−1. The value of k23 determined here is slightly higher than that reported by Baasandorj et al. ((5.73 ± 0.50) × 10−13 cm3 molecule−1 s−1
7), still they are in agreement within the ranges of uncertainty.
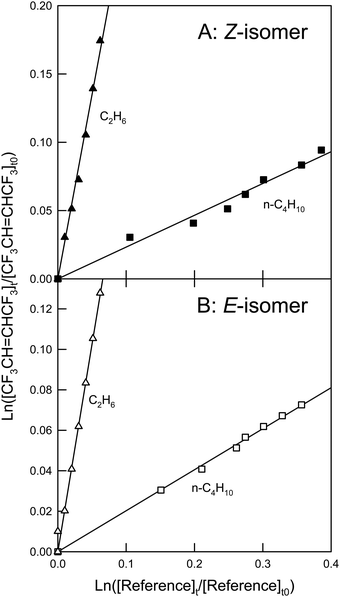 |
| Fig. 3 Panel A: Loss of Z-CF3CH CHCF3versus the loss of C2H6 (circles) and n-C4H10 (squares) in the presence of OD radicals. Panel B: Loss of E-CF3CH CHCF3versus the loss of C2H6 (circles) and n-C4H10 (squares) in the presence of OD radicals. | |
No other studies of OD radicals with HFOs are available in the literature making it difficult to compare the values of k23 and k24 to other similar compounds. The value of k24 is 4/5 the size of k23. This was unexpected, since the reactions of E-CF3CH
CHCF3 with the other oxidants in this study are significantly lower than the corresponding reactions of Z-CF3CH
CHCF3.
3.4 Absolute rate study of Z- and E-CF3CH
CHCF3 + O3
Reaction mixtures consisted of 1.53–1.78 mTorr Z-CF3CH
CHCF3 or 2.08–2.81 mTorr E-CF3CH
CHCF3, 0.73–6.41 Torr O3, and 0–10.4 mTorr c-C6H12 (cyclohexane) in 700 Torr air or 140 Torr O2 made up to 700 Torr with N2. Reaction (27) and (28) were monitored over time. | O3 + Z-CF3CH CHCF3 → Products | (27) |
| O3 + E-CF3CH CHCF3 → Products | (28) |
Ozonolysis can be a source of OH radicals. To avoid complications due to the reaction of Z- and E-CF3CH
CHCF3 + OH, c-C6H12 was added to the reaction mixture as an OH radical scavenger. In the absence of c-C6H12 the loss of Z-CF3CH
CHCF3 + O3 was found to be approximately 15% higher than when c-C6H12 was present. Over the ratios of [c-C6H12]/[Z- or E-CF3CH
CHCF3] = 1.8–6.8 no difference in the O3 reaction rate was observed. Fig. 4 and Fig. S1 in the ESI† show plots of the pseudo first order rate coefficients for Z- and E-CF3CH
CHCF3versus O3 concentration, with the former obtained from the slopes of the degradation of Z- and E-CF3CH
CHCF3versus time at different O3 concentrations (see insets in Fig. 4 and Fig. S1, ESI†). The slopes of the plots in Fig. 4 and Fig. S1 (ESI†) give the O3 rate coefficients for Z- and E-CF3CH
CHCF3, respectively. Hence, k27 = (6.25 ± 0.70) × 10−22 and k28 = (4.14 ± 0.42) × 10−22 cm3 molecule−1 s−1.
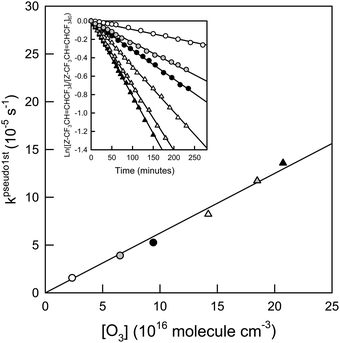 |
| Fig. 4 Pseudo first-order rate coefficients of the reaction O3 + Z-CF3CH CHCF3versus O3 concentration. The inset shows the loss of Z-CF3CH CHCF3versus time at different O3 concentrations. The symbols indicate different O3 partial pressures: 0.73 Torr (white circles), 2.01 Torr (gray circles), 2.91 Torr (black circles), 4.39 Torr (white triangles), 5.71 Torr (gray triangles), and 6.41 Torr (black triangles). See text for details. | |
Baasandorj et al.7 reported an upper limit for the reaction of Z-CF3CH
CHCF3 + O3 of k27 < 6 × 10−21 cm3 molecule−1 s−1, which is in agreement with the rate coefficient determined in the present work. The measured rate coefficient for reaction (28) is the slowest rate coefficient ever determined using the photochemical reactor at CCAR. Prior to this study the slowest rate coefficient for a similar compound reacting with ozone determined is of the reaction of Z-CF3CF
CHF + O3, which is determined to be (1.45 ± 0.15) × 10−21 cm3 molecule−1 s−1.16 The value of k28 is approximately 2/3 that of k27.
3.5 Reactivity trends
As mentioned earlier, one previous study7 exists in the literature on the reactivity of Z-CF3CH
CHCF3, but no studies have ever been conducted involving E-CF3CH
CHCF3. Furthermore, the kinetic study of Baasandorj et al. was limited to the reactions of OH radicals, OD radicals and O3. The rate coefficients reported by Baasandorj et al.7 are all in agreement with our results, although Baasandorj et al.7 also conclude that kOD = kOH for Z-CF3CH
CHCF3 within their reported uncertainties. As seen from Table 1, this is contrary to our findings. We find that the rate coefficient for the reaction with OD radicals is larger than that for the reaction with OH radicals for both compounds. Using computational methods, we investigate this further in Section 3.6.
A summary of the final rate coefficients obtained for all the kinetic experiments is shown in Table 2 along with the rate coefficient values determined previously by Baasandorj et al. as well as rate coefficients of the reactions for other structurally similar HFOs. The Z-isomer has reaction rate coefficients that are greater than those of the E-isomer in the reactions with all the oxidants studied here. This can be explained by the structural differences of the Z- and E-isomers, where more strain is released from the Z-isomer upon addition of the oxidants to the double bond, making it more favorable. Some trends can be observed for the HFOs presented in Table 2. Firstly, the rate coefficients of the reactions with Cl atoms determined here are of the same order as the ones determined for other similar HFOs, but with smaller reaction rate coefficients than the partially fluorinated HFOs, indicating a decrease in reactivity when exchanging a halogen atom (Cl or F) with a CF3 group. For CF3CF
CHF and CF3CH
CHCl the reactivities of the Z- and E-isomers towards Cl atoms are identical within the ranges of uncertainty. In this study the reactivity of the Z-isomer of CF3CH
CHCF3 has been found to be greater than that of the E-isomer. Secondly, the reactions of CF3CF
CF2, Z- and E-CF3CF
CHF with OH radicals proceed with rate coefficients that are one order of magnitude larger than those determined for the other HFOs. This could indicate an increasing effect on the reactivity towards OH radicals by having fluorine on both carbons on the double bond. Additional computational studies could aid the interpretation of this observation. The length of the perfluorinated chain may not be of great importance since the rate coefficient of E-(CF3)2CFCH
CHF + OH is approximately half the size of the one of E-CF3CH
CHF + OH and identical to the one of E-CF3CH
CHCl + OH. Thirdly, the HFOs listed from literature have reactivities towards O3 that are 1–2 orders of magnitude faster than the O3 reactivities of Z- and E-CF3CH
CHCF3. This could be due to differences in reactivity of fluorinated propenes and butenes towards O3. Additional studies on other fluorinated butenes would be needed to verify this.
Table 2 Final rate coefficients for the reactions of Z- and E-CF3CH
CHCF3 with Cl atoms, OH radicals, OD radicals, and O3 as well as the available literature data for Z-CF3CH
CHCF3 and structurally similar HFOs. All units are cm3 molecule−1 s−1
Compound |
k
Cl (10−11) |
k
OH (10−13) |
k
OD (10−13) |
k
O3 (10−22) |
Baasandorj et al.7
Andersen et al.18
Gierczak et al.38
Sulbaek Andersen et al.19
Hurley et al.16
Søndergaard et al.15
Mashino et al.17
Papadimitriou and Burkholder.39
|
Z-CF3CH CHCF3 |
2.59 ± 0.47 |
4.21 ± 0.62 |
6.94 ± 1.25 |
6.25 ± 0.70 |
— |
4.91 ± 0.50a |
5.73 ± 0.50a |
<60a |
E-CF3CH CHCF3 |
1.36 ± 0.27 |
1.72 ± 0.42 |
5.61 ± 0.98 |
4.14 ± 0.42 |
|
Z-CF3CH CHCl |
6.26 ± 0.84b |
8.45 ± 1.52b |
— |
15.3 ± 1.20b |
— |
9.46 ± 0.85c |
— |
— |
E-CF3CH CHCl |
5.22 ± 0.72d |
3.61 ± 0.37b |
— |
14.6 ± 1.20d |
— |
3.76 ± 0.35c |
— |
— |
Z-CF3CF CHF |
4.36 ± 0.48e |
12.2 ± 1.4e |
— |
14.5 ± 1.5e |
E-CF3CF CHF |
5.00 ± 0.56e |
21.5 ± 2.3e |
— |
198 ± 15e |
E-CF3CH CHF |
4.64 ± 0.59f |
9.25 ± 1.72f |
— |
28.1 ± 2.1f |
CF3CF CF2 |
2.7 ± 0.3g |
24 ± 3g |
— |
<30g |
E-(CF3)2CFCH CHF |
— |
3.26 ± 0.26h |
— |
— |
It is interesting to compare the reactivities of Z- and E-CF3CH
CHCF3 to Z-and E-CF3CH
CHCl. For both pairs of isomers it can be seen in Table 2 that both the Cl atom, the OH radical and the O3 rate coefficients obtained for the Z-isomers are faster than the ones obtained for the E-isomers.18,19 All the rate coefficients obtained for Z- and E-CF3CH
CHCl are larger than for Z- and E-CF3CH
CHCF3 even though they have the same number of halogen/CF3 substituents. This can be rationalized by the fact that the total number of fluorine atoms, and associated electron-withdrawing effect, in Z- and E-CF3CH
CHCF3 is double that found in Z- and E-CF3CH
CHCl. Furthermore, greater steric hindrance is associated with the CF3 group than that from the Cl atom. In the case of CF3CF
CHF, the E-isomer has a greater reactivity than the Z-isomer towards Cl atoms, OH radicals and O3, so no general Z- versus E-isomer trend can be assessed from the data in Table 2. Further experimental and computational studies of general trends in HFO reactivity would be of interest, but beyond the scope of the present study.
3.6 Computational study of the reactivity of OH and OD radicals
The calculated geometries of the reactant alkenes, Z- and E-CF3CH
CHCF3, are shown in Fig. 5 together with the geometries of the reaction complexes between the OH radical and the alkenes, the product alkyl radicals, and the connecting transition states. It is worth noting that the transition state in each case closely resembles the OH–alkene complex. We also note that the Z-isomer gives rise to the transition state resembling the complex closest, which is most likely due to the larger strain release in this case, which gives an energetic bonus earlier during the progress of the addition. The free energy changes relative to the separated reactants (alkene + OH and alkene + OD) are shown in Table 3. The formation of a complex between the E-isomer and the OH radical is endothermic by approximately 5.2 kJ mol−1 and there is a barrier for formation of the alkyl radical of 22.6 kJ mol−1. The overall reaction is exothermic by −98.1 kJ mol−1.
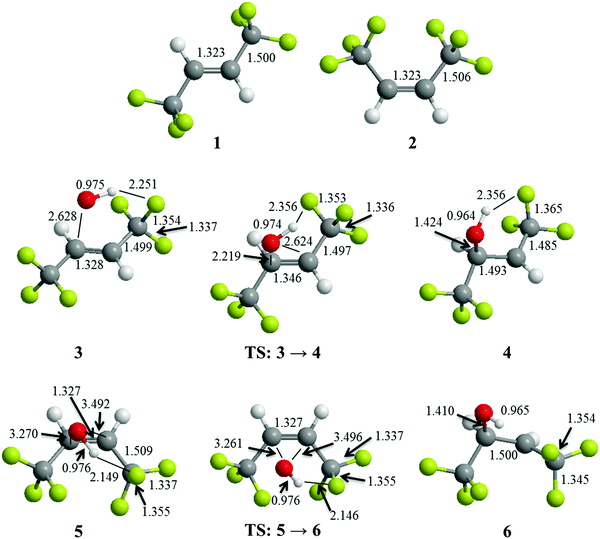 |
| Fig. 5 Structures of optimized geometries of 1: E-CF3CH CHCF3, 2: Z-CF3CH CHCF3, 3: complex of OH + E-CF3CH CHCF3, 4: alkyl radical product formed from OH + E-CF3CH CHCF3, 5: complex of OH + Z-CF3CH CHCF3, 6: alkyl radical product formed from OH + Z-CF3CH CHCF3, and transition states (TS) connecting 3 → 4 and 5 → 6. The highlighted bond lengths are in units of Ångstrøm. See text for details. | |
Table 3 Free energy changes associated with the formation of CF3CH
CHCF3–OH/OD complexes and the formation of alkyl radicals from the complexes as well as the free energy changes associated with the formation of alkyl radicals from the reactions with Cl atomsa
|
ΔrG (complex) |
ΔrG‡ (barrier) |
ΔrG (alkyl radical) |
G4MP2 values in kJ mol−1 at 298.15 K.
|
E-CF3CH CHCF3 + OH |
5.2 |
22.6 |
−98.1 |
E-CF3CH CHCF3 + OD |
4.9 |
22.1 |
−99.6 |
Z-CF3CH CHCF3 + OH |
2.7 |
7.5 |
−112.5 |
Z-CF3CH CHCF3 + OD |
2.5 |
7.3 |
−114.1 |
|
E-CF3CH CHCF3 + Cl |
— |
— |
−57 |
Z-CF3CH CHCF3 + Cl |
— |
— |
−60 |
The OD equivalent gives rise to a slightly more weakly bound complex (by 0.3 kJ mol−1) and a barrier, which is slightly lower (0.5 kJ mol−1). The formation of the alkyl radical on the other hand is more exothermic by 1.5 kJ mol−1. In the case of the Z-isomer the barriers and the endothermicities of formation are significantly reduced. The barrier for the formation of the alkyl radical from the RCF3–HO adduct is 7.5 kJ mol−1 whereas the adduct is 2.7 kJ mol−1 less stable than the separated reactants. The alkyl radical is more stable than the reactants by −112.5 kJ mol−1 and the increased stability reflects release of strain from the Z-isomer. The effect of the deuterium substitution is slightly reduced compared to the E-isomer. The barrier for the OD addition is lower by 0.2 kJ mol−1. The values are summarized in Table 3. The lower barrier in the case of OD is attributed to the generally weaker hydrogen bonds that are in play when a hydrogen is replaced by a deuterium.
The relative barrier difference for OH versus OD addition is in qualitative agreement with experimental finding that OD addition proceeds faster than OH addition in the sense that the barrier for OD addition is smaller for both Z- and E-CF3CH
CHCF3. The experimental result that the effect is smaller in the case of the Z-isomer is also in agreement with the calculations, because the calculated barrier is less impacted by isotope substitution in the Z-isomer case. At a first glance, the barrier differences seem small compared to the experimental relative rate coefficients. To assess the impact on the rate coefficients we evaluated the ratios of kOD/kOH for both Z- and E-CF3CH
CHCF3 from the partition functions, Q, and barrier differences using the equation:
|  | (III) |
where TS is the transition state, complex is the OH/OD–alkene complexes, subscripts on the partition functions denote either the OH or OD radical reaction, and ΔΔ
G‡ is the difference between the barrier heights to form the alkyl radical products. The
kOD/
kOH ratio is found to be 1.8 for
E-CF
3CH
![[double bond, length as m-dash]](https://www.rsc.org/images/entities/char_e001.gif)
CHCF
3 and 1.1 for
Z-CF
3CH
![[double bond, length as m-dash]](https://www.rsc.org/images/entities/char_e001.gif)
CHCF
3. This trend is consistent with the experimental results, showing a larger difference between the OD and OH rate coefficients for
E-CF
3CH
![[double bond, length as m-dash]](https://www.rsc.org/images/entities/char_e001.gif)
CHCF
3 than for
Z-CF
3CH
![[double bond, length as m-dash]](https://www.rsc.org/images/entities/char_e001.gif)
CHCF
3. The calculated total energies and optimized geometries can be found in the ESI
† (Tables S1 and S2).
3.7 Product study of E-CF3CH
CHCF3 + Cl
The initial reaction mixtures for the product study of the reaction E-CF3CH
CHCF3 + Cl consisted of 2.61–4.07 mTorr E-CF3CH
CHCF3 and 73.2–89.0 mTorr Cl2 in 700 Torr air/N2/O2 diluent. The mixtures were subjected to a total of 33–52 seconds of UV irradiation. The reaction proceeds with the addition of a Cl atom to the double bond creating an alkyl radical, that will react with O2 forming a peroxy radical (RO2):
Cl + E-CF3CH CHCF3 → CF3CHClCHCF3 (14) |
| CF3CHClCHCF3 + O2 → CF3CHClCH(O2)CF3 | (29) |
The peroxy radical can react with other peroxy radicals, itself or NO (if present), to form an alkoxy radical (RO): | CF3CHClCH(O2)CF3 + RO2 → CF3CHClCH(O˙)CF3 + RO + O2 | (30) |
The alkoxy radical can then react with O2 to give a ketone or decompose via C–C bond scission to give a CF3CHCl radical and CF3CHO. CF3CHCl will react with O2 giving CF3C(O)Cl: | CF3CHClCH(O˙)CF3 + O2 → CF3CHClC(O)CF3 + HO2 | (31a) |
| CF3CHClCH(O˙)CF3 + M → CF3CHCl + CF3CHO + M | (31b) |
|  | (32) |
Fig. 6 shows spectra of a mixture of 2.61 mTorr E-CF3CH
CHCF3 and 73.2 mTorr Cl2 in 700 Torr air, before (panel A) and after (panel B) 24 seconds of UV irradiation. 59% of E-CF3CH
CHCF3 was consumed in the irradiation. Panel E is a residual spectrum obtained by subtracting all remaining E-CF3CH
CHCF3 from panel B (panel B – 0.41 × panel A). IR features present in panel E are assigned to CF3CHClC(O)CF3, as the only observed product. While we do not have sample of CF3CHClC(O)CF3 with which to obtain a genuine reference spectrum, a calculated spectrum of the compound is shown in panel F. This and other spectra were calculated using the GAUSSIAN 09 suite of programs, revision D.01 at the B3LYP/6-31+G(d,p) level (see below).12 No formation of CF3CHO (panel C) or CF3C(O)Cl (panel D) was observed in these experiments. Upper limits for the yields of CF3CHO and CF3C(O)Cl were determined as 1% for both compounds. Therefore, we conclude that reaction (31b) is not significant in the Cl atom initiated degradation. Fig. 7 shows the formation of CF3CHClC(O)CF3 as a function of the loss of E-CF3CH
CHCF3 in the presence of Cl atoms.
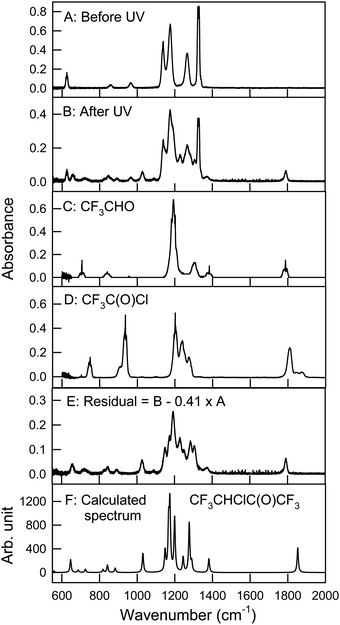 |
| Fig. 6 Panel A: IR spectrum of 2.61 mTorr E-CF3CH CHCF3 and 73.2 mTorr Cl2 before UV irradiation, panel B: spectrum of the reaction mixture after 24 seconds UV irradiation, panel C: reference spectrum of CF3CHO, panel D: reference spectrum of CF3C(O)Cl, panel E: residual spectrum (panel B – 0.41 × panel A) assigned to CF3CHClC(O)CF3, and panel F: calculated IR spectrum of CF3CHClC(O)CF3 at the B3LYP/6-31+G(d,p) level. See text for details. | |
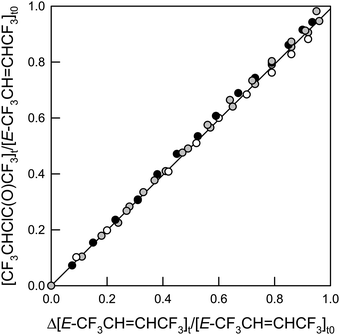 |
| Fig. 7 Formation of CF3CHClC(O)CF3versus the loss of E-CF3CH CHCF3 in the presence of Cl atoms. The shades indicate the O2 partial pressure: 16 Torr (white), 140 Torr (gray), and 700 Torr (black) in a total of 700 Torr made up with air or N2. | |
Calculated spectra of E-CF3CH
CHCF3 and several conformers of CF3CHClC(O)CF3 were calculated at the B3LYP/6-31+G(d,p) level. One conformer of CF3CHClC(O)CF3 was calculated at the ωB97XD/cc-pVTZ level being in agreement with the other calculations. The optimized geometries and IR spectra are shown in the ESI† (Tables S3–S8 and Fig. S2–S7).
3.8 Product study of Z-CF3CH
CHCF3 + Cl
A product study of the reaction of Z-CF3CH
CHCF3 + Cl was performed using initial reaction mixtures of 1.12–1.91 mTorr Z-CF3CH
CHCF3, 75.4–87.3 mTorr Cl2, and 0 or 10.4 mTorr NO in 700 Torr air or O2 diluent. The mixtures were subjected to a total of 17–29 seconds of UV irradiation. The reaction of Z-CF3CH
CHCF3 + Cl is initiated by the addition of Cl atoms to the double bond of Z-CF3CH
CHCF3 creating an alkyl radical as for E-CF3CH
CHCF3 in reaction (14).
Cl + Z-CF3CH CHCF3 → CF3CHClCHCF3 (13) |
After addition of the Cl atom, the Z/E isometry is lost, free rotation of the C–C bond is possible, and the following reactions in the degradation of the Z-isomer will be the same as observed for the E-isomer in reactions (29)–(32).
Fig. 8 shows spectra of a reaction mixture of 1.91 mTorr Z-CF3CH
CHCF3 and 75.4 mTorr Cl2 in 700 Torr air, before (panel A) and after (panel B) 17 seconds of UV irradiation. 62% of Z-CF3CH
CHCF3 was consumed in the irradiation. Panel C shows a reference spectrum of E-CF3CH
CHCF3 and panel D is a residual spectrum obtained by subtracting all remaining features of Z-CF3CH
CHCF3 (0.38 × panel A) and E-CF3CH
CHCF3 (0.03 × panel C) from panel B. This spectrum is assigned to CF3CHClC(O)CF3. Panel E shows the residual spectrum of CF3CHClC(O)CF3 obtained from the product study of E-CF3CH
CHCF3.
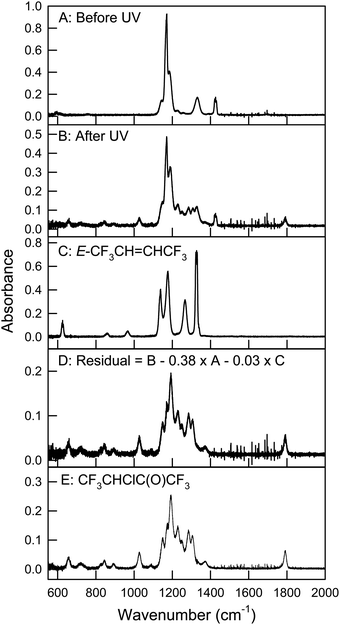 |
| Fig. 8 Panel A: A mixture of 1.91 mTorr Z-CF3CH CHCF3 and 75.4 mTorr Cl2 in 700 Torr air before UV irradiation, panel B: the reaction mixture after 17 seconds UV irradiation, panel C: reference spectrum of E-CF3CH CHCF3, panel D: residual spectrum obtained from subtracting features of Z- and E-CF3CH CHCF3 (panel B – 0.38 × panel A – 0.03 × panel C) assigned to CF3CHClC(O)CF3, and panel E: spectrum of CF3CHClC(O)CF3 obtained from the product study of E-CF3CH CHCF3 in the presence of Cl atoms. See text for details. | |
Fig. 9 shows the formation of CF3CHClC(O)CF3 and E-CF3CH
CHCF3versus the loss of Z-CF3CH
CHCF3. The shades of the symbols indicate different O2 partial pressures and the presence or absence of NO. No effect of varying the O2 partial pressure was observed. The formation of E-CF3CH
CHCF3 is evidence of isomerization. After a Cl atom has added to the double bond in Z-CF3CH
CHCF3 (reaction (13)), the center C–C bond has free rotation and the double bond will reform to the more stable E-conformation in competition with reaction (29) expelling a Cl atom:
| CF3CHClCHCF3 → E-CF3CH CHCF3 + Cl | (33) |
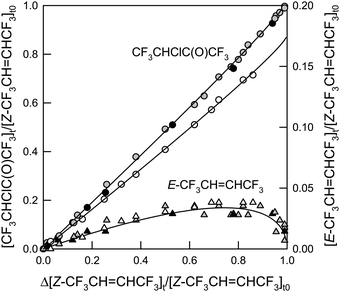 |
| Fig. 9 Formation of CF3CHClC(O)CF3 (circles, left y-axis) and E-CF3CH CHCF3 (triangles, right y-axis) versus the loss of Z-CF3CH CHCF3 in the presence of Cl atoms in a total of 700 Torr air in the absence (gray) and presence (white) of NO or 700 Torr O2 in the absence of NO (black). The lines are non-linear fits to the data. See text for details. | |
As discussed in Section 3.7 E-CF3CH
CHCF3 reacts with Cl to give 100% CF3CHClC(O)CF3. No evidence of isomerization was observed in the degradation of E-CF3CH
CHCF3. During the course of the reaction of Z-CF3CH
CHCF3 with Cl atoms the apparent yield of E-CF3CH
CHCF3 decreases and the concentration of CF3CHClC(O)CF3 increases in a non-linear way. Fits to the data are based on reactions (34a), (34b) and (14):
| Cl + Z-CF3CH CHCF3 → αE-CF3CH CHCF3 + Cl | (34a) |
|  | (34b) |
|  | (14) |
Here
α and
β are the initial yields of
E-CF
3CH
![[double bond, length as m-dash]](https://www.rsc.org/images/entities/char_e001.gif)
CHCF
3 and CF
3CHClC(O)CF
3, respectively. A method described by Meagher
et al.,
20 is used to fit the
E-CF
3CH
![[double bond, length as m-dash]](https://www.rsc.org/images/entities/char_e001.gif)
CHCF
3 and CF
3CHClC(O)CF
3 data. The equation describing the fractional conversion of
Z- to
E-CF
3CH
![[double bond, length as m-dash]](https://www.rsc.org/images/entities/char_e001.gif)
CHCF
3 is:
|  | (IV) |
Here
k34 and
k14 are the rate coefficients of the reactions of Cl +
Z- and
E-CF
3CH
![[double bond, length as m-dash]](https://www.rsc.org/images/entities/char_e001.gif)
CHCF
3 determined above, where
k34 =
k13, and
x is the conversion of
Z-CF
3CH
![[double bond, length as m-dash]](https://www.rsc.org/images/entities/char_e001.gif)
CHCF
3, defined as:
|  | (V) |
In this fit, the
k14/
k13 ratio is known, so this value is fixed to be:
|  | (VI) |
This gives an initial yield of
E-CF
3CH
![[double bond, length as m-dash]](https://www.rsc.org/images/entities/char_e001.gif)
CHCF
3 of
α = (6.9 ± 0.8)%. The fit to the CF
3CHClC(O)CF
3 data uses
eqn (IV) as a modification to a linear fit, accounting for
reaction (14), describing the conversion of
Z-CF
3CH
![[double bond, length as m-dash]](https://www.rsc.org/images/entities/char_e001.gif)
CHCF
3 to CF
3CHClC(O)CF
3:
|  | (VII) |
Fitting
eqn (VII) to the CF
3CHClC(O)CF
3 data with the known values of
k14/
k13 and
α, the initial yield of CF
3CHClC(O)CF
3 is
β = (95 ± 10)% in the absence of NO. Combining the initial yields of
E-CF
3CH
![[double bond, length as m-dash]](https://www.rsc.org/images/entities/char_e001.gif)
CHCF
3 and CF
3CHClC(O)CF
3 mass balance is obtained. It is clear that at atmospheric pressure, the dominant pathway of the reaction of Cl +
Z-CF
3CH
![[double bond, length as m-dash]](https://www.rsc.org/images/entities/char_e001.gif)
CHCF
3 is
viareaction (34b).
In the presence of NO the yield of CF3CHClC(O)CF3 is slightly decreased compared to the experiments in the absence of NO. The yield of E-CF3CH
CHCF3 is not affected by the presence of NO, so eqn (VII) can be used to fit the CF3CHClC(O)CF3 data using the known values of k14/k13 and α. This gives an initial yield of CF3CHClC(O)CF3 of β = (81 ± 8)% in the presence of NO. The formation of ClNO and ClNO2 was observed in these experiments. A minor product with an IR absorption peak at 1699 cm−1 was observed, which, assuming conserved mass balance, could have a yield of 12%. This compound was not positively identified, but could be due to the formation of an organic nitrate compound.
While we did not perform any comprehensive OH radical initiated product studies of Z- and E-CF3CH
CHCF3, Baasandorj et al. identified some products of the reaction of Z-CF3CH
CHCF3 with OH radicals.7 They observed C(O)F2 and CF3CHO as the main F-containing products, suggesting that C–C scission of the central carbon bond could be a more significant pathway in the atmospheric degradation initiated by OH radical than what we have been able to observe above in the Cl atom initiated reaction.
Calculated IR spectra of two conformers of Z-CF3CH
CHCF3 at the B3LYP/6-31+G(d,p) level are supplied in the ESI† (Tables S9, S10 and Fig. S8, S9) and show good agreement with the experimental spectrum.
3.9 Isomerization of Z- and E-CF3CH
CHCF3
Experiments were performed to investigate any potential isomerization in the reactions of Z-CF3CH
CHCF3 with OH or OD radicals giving E-CF3CH
CHCF3 as observed for the reaction of Z-CF3CH
CHCF3 with Cl atoms. Reaction mixtures consisting of 1.21–1.37 mTorr Z-CF3CH
CHCF3 and 43.9 mTorr CH3ONO or 42.9 mTorr CD3ONO in 700 Torr air diluent were subjected to a total of 110–210 seconds of UV irradiation. No formation of E-CF3CH
CHCF3 was observed in these experiments. Calculations were performed of the energy of the initial alkyl radical formed from the addition of Cl atoms to Z-CF3CH
CHCF3. The alkyl radical energy is approximately twice that of the alkyl radical formed with OH or OD radicals (see Table 3), making it more likely that the reaction can proceed in the reverse direction eliminating the Cl atom and reforming CF3CH
CHCF3. The E-isomer has less strain than the Z-isomer thus making it more likely to be formed. A similar calculation was performed of the alkyl radical formed from the reaction of Cl + E-CF3CH
CHCF3. The energy of this initial alkyl radical is slightly smaller than that from the Z-isomer (see Table 3), but in the experiments, no isomerization was observed. The optimized structures of both initial alkyl radicals formed from Cl atoms are shown in Fig. S11 in the ESI.† Comparing the barriers of formation of the alkyl radicals from the OH/OD radical additions, the E-isomer barriers are more than double those of the Z-isomer, making the barriers the potential limiting factor of isomerization. Similar barriers can be expected from the Cl atom additions to Z- and E-CF3CH
CHCF3, explaining why no isomerization was observed for the reaction Cl + E-CF3CH
CHCF3. It would be expected that E-CF3CH
CHCF3 will not isomerize in the reactions with OH or OD radicals for the same reasons as discussed above. No additional experiments were performed with OH/OD + E-CF3CH
CHCF3 besides the ones used for determining the rate coefficients.
3.10 Product study of CF3CHClC(O)CF3 + Cl
To further investigate the oxidation of the primary product, CF3CHClC(O)CF3, experiments were conducted in which all of the starting material, Z-CF3CH
CHCF3, was allowed to react with Cl to generate CF3CHClC(O)CF3. Only then, the loss of CF3CHClC(O)CF3 and the subsequent formation of products was monitored, normalizing the products to the initial concentration of CF3CHClC(O)CF3. All E-CF3CH
CHCF3 had also reacted at this point. The initial reaction mixtures were 1.12–1.91 mTorr Z-CF3CH
CHCF3 and 75.4–87.3 mTorr Cl2 in 700 Torr air or O2. The mixtures were subjected to a total of 3150–5400 seconds of UV irradiation after all Z-CF3CH
CHCF3 was converted to CF3CHClC(O)CF3. Three products are observed in the degradation of CF3CHClC(O)CF3: CF3C(O)Cl, C(O)F2, and CF3O3CF3. Fig. 10 shows IR spectra recorded before (panel A) and after (panel B) UV irradiation of a mixture of CF3CHClC(O)CF3 and Cl2. Panel C shows the resulting spectrum after subtracting all features of CF3CHClC(O)CF3 in panel A from panel B (panel B – 0.56 × panel A). Panels D and E show reference spectra of C(O)F2 and CF3C(O)Cl, respectively. The residual obtained when subtracting features of C(O)F2 and CF3C(O)Cl from panel C is shown in panel F. Panel G shows a reference spectrum of CF3O3CF3. The residual spectrum is assigned to CF3O3CF3, and quantified as such.21
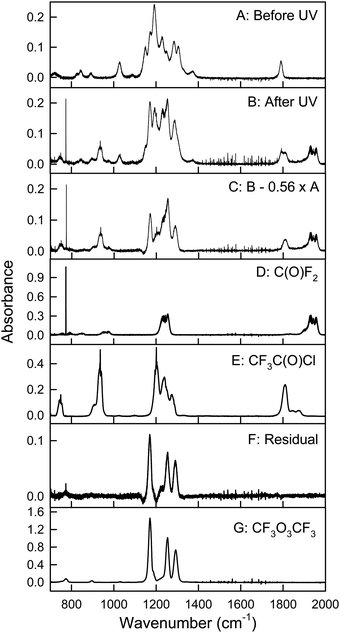 |
| Fig. 10 Panel A: IR spectrum of CF3CHClC(O)CF3 + Cl2 before UV irradiation, panel B: spectrum of the reaction mixture after 25 minutes of UV irradiation, panel C: the resulting spectrum of subtracting the remaining features of CF3CHClC(O)CF3 from panel B (panel B – 0.56 × panel A), panel D: reference spectrum of C(O)F2, panel E: reference spectrum of CF3C(O)Cl, panel F: residual spectrum, when subtracting C(O)F2 and CF3C(O)Cl from panel C, and panel G: reference spectrum of CF3O3CF3. The residual spectrum in panel F is assigned to CF3O3CF3. See text for details. | |
The degradation of CF3CHClC(O)CF3 is initiated by Cl atoms by abstraction of the hydrogen atom forming an alkyl radical that reacts with O2 forming a peroxy radical:
| CF3CHClC(O)CF3 + Cl → CF3CClC(O)CF3 + HCl | (35) |
| CF3CClC(O)CF3 + O2 → CF3C(O2)ClC(O)CF3 | (36) |
The peroxy radical will then react with itself or NO (if present) or another peroxy radical forming an alkoxy radical:
| CF3C(O2)ClC(O)CF3 + RO2 → CF3C(O˙)ClC(O)CF3 + RO + O2 | (37) |
The alkoxy radical formed will break by C–C scission to give CF
3C(O)Cl and a C(O)CF
3 radical that will either decompose to give CO and a CF
3 radical or react with O
2 to give a peroxy radical:
| CF3C(O˙)ClC(O)CF3 → CF3C(O)Cl + C(O)CF3 | (38) |
| C(O)CF3 + O2 → (O2)C(O)CF3 | (40) |
The alkyl radical, C(O)CF
3, will predominantly react with O
2 (97% under laboratory conditions, 99.5% in the atmosphere)
viareaction (40) and the remainder (3% or 0.5%) will decompose
viareaction (39).
22 The peroxy radical formed in
reaction (40) will then react with itself or another peroxy radical giving an alkoxy radical, which decomposes to give a CF
3 radical and CO
2:
22 | (O2)C(O)CF3 + RO2 → (O)C(O)CF3 + RO + O2 | (41) |
| (O)C(O)CF3 → CO2 + CF3 | (42) |
The CF
3 radical will react with O
2 forming a peroxy radical that can react with another peroxy radical to give an alkoxy radical and O
2:
| CF3O2 + RO2 → CF3O + RO + O2 | (44) |
The formed alkoxy radical CF
3O can abstract a hydrogen atom from a hydrocarbon (RH) forming an alcohol. This alcohol will eliminate HF forming C(O)F
2:
| CF3O + RH → CF3OH + R | (45) |
The peroxy radical CF
3O
2 and the alkoxy radical CF
3O can also react with each other forming the trioxide CF
3O
3CF
3 (bis-(trifluoromethyl)trioxide):
| CF3O + CF3O2 + M → CF3O3CF3 + M | (47) |
Fig. 11 shows the formation of products versus the loss of CF3CHClC(O)CF3. The solid lines through the CF3C(O)Cl data are linear least squares analyses of the data. The slopes give yields of CF3C(O)Cl of (78 ± 8)% in the experiments where UVB lamps were used for Cl2 photolysis and (102 ± 10)% when using UVA lamps to photolyze Cl2. The dotted lines through the C(O)F2 and CF3O3CF3 data are trend lines to serve as visual aids for inspection of the data. As a measure of the combined yield of CF3 radicals formed in the degradation of CF3CHClC(O)CF3, diamonds show the sum of the fractional formation of C(O)F2 and twice the fractional formation of CF3O3CF3. As described above the CF3 radicals will eventually give either C(O)F2 or CF3O3CF3. CF3O3CF3 will react on the walls of the chamber and form two C(O)F2 molecules. This is evidenced in Fig. 11, by the decrease in the slopes of formation of CF3O3CF3 and the increase in the slopes of the formation of C(O)F2 during the course of the experiments. The solid lines through the diamonds in Fig. 11 are linear regressions giving yields of CF3 radicals of (95 ± 10)% and (122 ± 13)% for the experiments using UVA and UVB lamps, respectively. UVB lights are expected to photolyze CF3CHClC(O)CF3, as has been observed for similar fluorinated ketones.23,24 The products observed in the experiments using UVB lamps are therefore a combination of the reaction with Cl atoms and of the photolysis. The experiment using UVA lamps gives products of the reaction with Cl atoms only. Here, the yield of the reaction is indistinguishable from 100% of CF3C(O)Cl and the co-formed CF3 radical (observed as C(O)F2 and CF3O3CF3). In the experiments using UVB lamps, (78 ± 8)% of the loss of CF3CHClC(O)CF3 is via reaction with Cl atoms observed as CF3C(O)Cl and the remaining is loss by photolysis. The photolysis of CF3CHClC(O)CF3 gives an alkoxy radical that will break into two radicals; CF3CH and C(O)CF3:
| CF3CHClC(O)CF3 + hν(UVB) → CF3CHC(O)CF3 + Cl | (48) |
| CF3CHC(O)CF3 → CF3CH + C(O)CF3 | (49) |
The CF
3CH radical will react with O
2 forming a peroxy radical and then proceed to react with another peroxy radical to form CF
3CHO:
| CF3CHO2 + RO2 → CF3CHO + RO + O2 | (51) |
CF
3CHO has a fast reaction with Cl giving a C(O)CF
3 radical:
25,26 | CF3CHO + Cl → C(O)CF3 + HCl | (52) |
The C(O)CF
3 radical decomposition will follow the reaction pathway outlined in
reactions (39)–(47) yielding a CF
3 radical that will react to give either C(O)F
2 or CF
3O
3CF
3. The total observed formation of CF
3 radicals in the experiments with UVB lamps is 122% (as mentioned above). Of this, 78% is the co-product of CF
3C(O)Cl from the Cl atom reaction, leaving 44% CF
3 radicals formed from the photolysis of CF
3CHClC(O)CF
3. The photolysis of CF
3CHClC(O)CF
3 and the reaction with Cl atoms balances the mass in the experiments using UVB lamps. No change in the product yields was observed with an O
2 partial pressure varying between 140 and 700 Torr.
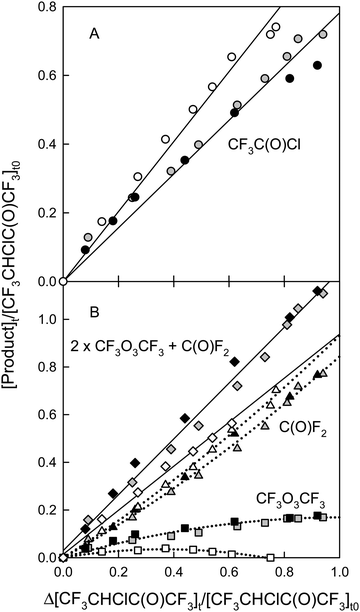 |
| Fig. 11 Panel A: Formation of CF3C(O)Cl (circles) versus the loss of CF3CHClC(O)CF3 in the presence of Cl atoms in a total of 700 Torr air using UVA (white) or UVB (gray) lamps and 700 Torr O2 using UVB lamps (black). Panel B: C(O)F2 (triangles), and CF3O3CF3 (squares) versus the loss of CF3CHClC(O)CF3 in the presence of Cl atoms in a total of 700 Torr air using UVA (white) or UVB (gray) lamps and 700 Torr O2 using UVB lamps (black). The diamond symbols are the sum of CF3 radicals formed (2 × CF3O3CF3 + C(O)F2). The solid lines are linear fits to the data and the dotted lines are trend lines through the data to ease visual inspection of the data, see text for details. | |
3.11 Atmospheric lifetimes of Z- and E-CF3CH
CHCF3
Compounds such as Z- and E-CF3CH
CHCF3 can leave the atmosphere via photolysis, wet and dry deposition or reaction with atmospheric oxidants: NO3 radicals, O3, OH radicals and Cl atoms. Z- and E-CF3CH
CHCF3 does not absorb light in wavelengths <200 nm, so photolysis in the troposphere is not important.27 Compounds such as Z- and E-CF3CH
CHCF3 are likely to be in the gas phase rather than aqueous phase, so wet deposition is not likely to be an important atmospheric sink. The volatility of both compounds will render dry deposition unlikely as an atmospheric removal mechanism. The reaction with NO3 radicals and O3 is too slow to be of significance when compared to that of reaction with OH radicals and Cl atoms.28 Even though the rate coefficients for the reactions of Z- and E-CF3CH
CHCF3 with Cl atoms are two orders of magnitude faster than those of the reactions with OH radicals, the reaction with OH radicals is the main sink for Z- and E-CF3CH
CHCF3. The global average atmospheric concentration of Cl atoms is generally low; approximately [Cl] = 1 × 103 atom cm−3,29 which is 3 orders of magnitude lower than the average global of OH radical concentration of [OH] = 1 × 106 molecule cm−3.30 Locally Cl atom concentrations can be significantly higher, e.g. [Cl] = 1.8 × 104 atom cm−3 in the marine boundary layer, so in some cases the Cl atom the reactions can compete with the OH radical reaction.31 The rate coefficients of the reactions with OH radicals determined here are at a temperature of 296 ± 2 K, but the appropriate temperature used to estimate atmospheric lifetimes based on OH radical reactions is 272 K.32 This needs to be considered when estimating the atmospheric lifetimes of the two compounds. Baasandorj et al. performed their study of the kinetics of the reaction of Z-CF3CH
CHCF3 + OH radicals at temperatures of 212–374 K and found an Arrhenius expression for temperatures <300 K giving k19 = (5.19 ± 0.53) × 10−13 cm3 molecule−1 s−1 at 272 K, showing a slight temperature dependence.7 This value is indistinguishable from our value for k19 determined at here at 296 K ± 2 K. It is assumed that the reaction of E-CF3CH
CHCF3 + OH will have a similar temperature dependency. Hence, we use the rate coefficients determined in this study at 296 ± 2 K to estimate atmospheric lifetimes of Z- and E-CF3CH
CHCF3 of 27 and 67 days, respectively. Baasandorj et al. estimated the lifetime of Z-CF3CH
CHCF3 as 22 days, in reasonable agreement with our present estimate.7
3.12 IR spectra, radiative efficiencies, and global warming potentials of Z- and E-CF3CH
CHCF3
The IR spectra in absorption cross section σ of Z- and E-CF3CH
CHCF3 are shown in Fig. 12. The integrated absorption cross sections of the two spectra (550–2000 cm−1) have been determined to be (2.51 ± 0.13) × 10−16 and (2.96 ± 0.15) × 10−16 cm molecule−1, for Z- and E-CF3CH
CHCF3, respectively.
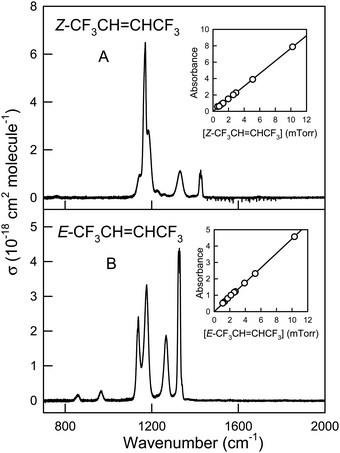 |
| Fig. 12 IR spectra of 1.30 mTorr Z-CF3CH CHCF3 (panel A) and 2.25 mTorr E-CF3CH CHCF3 (panel B). The insets show the linearity of the absorbance. | |
Using the method described by Pinnock et al.33 the radiative efficiencies for Z- and E-CF3CH
CHCF3 were found to be 0.334 and 0.300 W m−2 ppb−1, respectively. The value for Z-CF3CH
CHCF3 is in agreement with the value estimated by Baasandorj et al. of 0.38 W m−2 ppb−1 using the same method.7 This method assumes that the gases are well-mixed in the atmosphere. For gases such as Z- and E-CF3CH
CHCF3 this is not the case since their atmospheric lifetime is not long enough for vertical mixing. Therefore we employ a correction factor, f(τ), dependent on the atmospheric lifetime of the compounds, τ, as described by Hodnebrog et al.,34
|  | (VIII) |
where
a,
b,
c, and
d are constants with values of 2.962, 0.9312, 2.994, and 0.9302, respectively. The correction factors were calculated to be
f(
τ) = 0.21 and 0.38, for
Z- and
E-CF
3CH
![[double bond, length as m-dash]](https://www.rsc.org/images/entities/char_e001.gif)
CHCF
3 respectively. This gives effective values of the radiative efficiencies for
Z- and
E-CF
3CH
![[double bond, length as m-dash]](https://www.rsc.org/images/entities/char_e001.gif)
CHCF
3 of 0.069 and 0.113 W m
−2 ppb
−1, respectively. The radiative efficiencies and atmospheric lifetimes are used in the calculation of the global warming potentials (GWPs) of
Z- and
E-CF
3CH
![[double bond, length as m-dash]](https://www.rsc.org/images/entities/char_e001.gif)
CHCF
3 using the following equation,
| 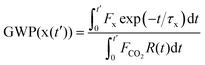 | (IX) |
where
Fx is the radiative efficiency of x (x =
Z- or
E-CF
3CH
![[double bond, length as m-dash]](https://www.rsc.org/images/entities/char_e001.gif)
CHCF
3),
τx is the atmospheric lifetime of x with an exponential decay,
t′ is the time horizon (typically 20, 100, and 500 years),
FCO2 is the radiative efficiency of CO
2, and
R(
t) is a response function that describes the decay of an instantaneous pulse of CO
2. The GWP is calculated relative to CO
2 mass for mass, and the denominator in
eqn (IX) is the absolute global warming potential (AGWP) values of CO
2. These have been determined to be AGWP(CO
2) = 2.49 × 10
−14, 9.17 × 10
−14, and 32.2 × 10
−14 W year m
−2 kg
−1, (= 0.196, 0.722, and 2.534 W year m
−2 ppb
−1) for 20, 100, and 500 year time horizons.
35 Thus we estimate the GWPs for
Z-CF
3CH
![[double bond, length as m-dash]](https://www.rsc.org/images/entities/char_e001.gif)
CHCF
3 to be 6, 2, and 0 for time horizons of 20, 100, and 500 years, respectively. The GWPs for
E-CF
3CH
![[double bond, length as m-dash]](https://www.rsc.org/images/entities/char_e001.gif)
CHCF
3 are estimated as 26, 7, and 2 for time horizons of 20, 100, and 500 years, respectively.
4. Conclusions and atmospheric impact
The present work provides a comprehensive description of the atmospheric chemistry and fate of Z- and E-CF3CH
CHCF3. The kinetics of the reactions of Z- and E-CF3CH
CHCF3 with Cl atoms, OH radicals, OD radicals, and O3 have been determined. This is the first kinetic study of E-CF3CH
CHCF3 and the first determination of the rate coefficients of the reactions of Z-CF3CH
CHCF3 with Cl atoms and O3. Using the obtained rate coefficients for the reactions with OH radicals we find that both Z- and E-CF3CH
CHCF3 have short atmospheric lifetimes of 27 and 67 days, respectively. GWP values for both compounds are small; 2 and 7 for the 100 year time horizon for Z- and E-CF3CH
CHCF3, respectively. The Cl atom initiated degradation mechanism of Z- and E-CF3CH
CHCF3 is summarized in Fig. 13. For both Z- and E-CF3CH
CHCF3 the primary degradation product is CF3CHClC(O)CF3. E-CF3CH
CHCF3 gives CF3CHClC(O)CF3 in a yield indistinguishable from 100% and Z-CF3CH
CHCF3 gives (95 ± 10)% CF3CHClC(O)CF3 and (7 ± 1)% E-CF3CH
CHCF3. CF3CHClC(O)CF3 reacts with Cl atoms to give the secondary product CF3C(O)Cl in a yield indistinguishable from 100%, co-formed with a CF3 radical observed as C(O)F2 and CF3O3CF3. Calculations were performed to investigate differences in the reactivities of Z- and E-CF3CH
CHCF3 towards OH and OD radicals and the observed isomerization of Z-CF3CH
CHCF3 giving E-CF3CH
CHCF3 in the experiments with Cl atoms. Energies of a pre-reaction complex, transition states and the formed alkyl radical were calculated for both alkenes for the reactions with OH and OD radicals as well as the energies of the alkyl radicals of the reaction of both alkenes with Cl atoms. The calculated energies are in good agreement with the experimental observations and in agreement with the observed reactivity trends. Based on the short atmospheric lifetimes, the small GWP values and the non-toxic products of the Cl atom initiated degradation, the atmospheric impact of both Z- and E-CF3CH
CHCF3 is negligible. Further studies of the OH radical initiated products are needed to assess the atmospheric impact of the products formed by atmospheric degradation.
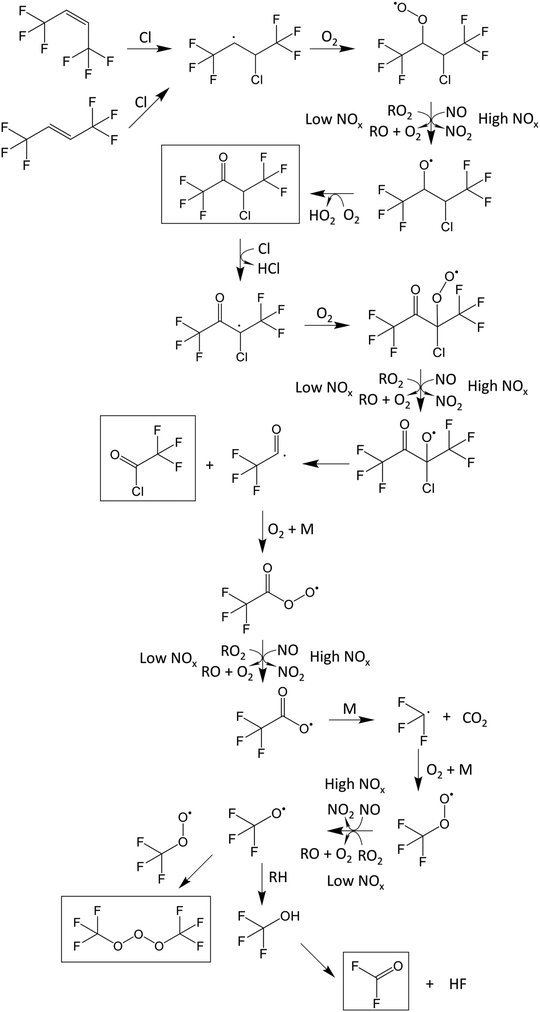 |
| Fig. 13 Cl atom initiated degradation mechanism of Z- and E-CF3CH CHCF3. The observed products are indicated by the boxes. | |
Acknowledgements
The authors thank Rajiv R. Singh (Honeywell) for supplying us with a sample of Z-CF3CH
CHCF3. We thank Mogens Brøndsted Nielsen and Martyn Jevric (University of Copenhagen) for supplying samples of CD3ONO and (CH3)2CHONO and for aid in the synthesis of CH3ONO. Thanks are due to Timothy J. Wallington (Ford Motor Company) for kindly providing the absorption cross section of CF3O3CF3.
References
- M. J. Molina and F. S. Rowland, Nature, 1974, 249, 810–812 CrossRef CAS.
- F. S. Rowland, Ambio, 1990, 19, 281–292 Search PubMed.
- J. C. Farman, B. G. Gardiner and J. D. Shanklin, Nature, 1985, 315, 207–210 CrossRef CAS.
- T. Midgley and A. L. Henne, Ind. Eng. Chem., 1930, 22, 542–545 CrossRef CAS.
- DuPont, DuPont Develops HFO Foam Expansion Agent with Low Global Warming Potential, Additives for Polymers, 2014, 6, 2 Search PubMed.
- F. Molés, J. Navarro-Esbrí, B. Peris, A. Mota-Babiloni, Á. Barragán-Cervera and K. Kontomaris, Appl. Therm. Eng., 2014, 71, 204–212 CrossRef.
- M. Baasandorj, A. R. Ravishankara and J. B. Burkholder, J. Phys. Chem. A, 2011, 115, 10539–10549 CrossRef CAS PubMed.
- E. J. K. Nilsson, C. Eskebjerg and M. S. Johnson, Atmos. Environ., 2009, 43, 3029–3033 CrossRef CAS.
- L. A. Curtiss, P. C. Redfern and K. Raghavachari, J. Chem. Phys., 2007, 127, 124105 CrossRef PubMed.
-
M. J. Frisch, G. W. Trucks, H. B. Schlegel, G. E. Scuseria, M. A. Robb, J. R. Cheeseman, G. Scalmani, V. Barone, B. Mennucci, G. A. Petersson, H. Nakatsuji, M. Caricato, X. Li, H. P. Hratchian, A. F. Izmaylov, J. Bloino, G. Zheng, J. L. Sonnenberg, M. Hada, M. Ehara, K. Toyota, R. Fukuda, J. Hasegawa, M. Ishida, T. Nakajima, Y. Honda, O. Kitao, H. Nakai, T. Vreven, J. J. A. Montgomery, J. E. Peralta, F. Ogliaro, M. Bearpark, E. B. J. J. Heyd, K. N. Kudin, V. N. Staroverov, R. Kobayashi, J. Normand, K. Raghavachari, A. Rendell, J. C. Burant, S. S. Iyengar, J. Tomasi, M. Cossi, N. Rega, J. M. Millam, M. Klene, J. E. Knox, J. B. Cross, V. Bakken, C. Adamo, J. Jaramillo, R. Gomperts, R. E. Stratmann, O. Yazyev, A. J. Austin, R. Cammi, C. Pomelli, J. W. Ochterski, R. L. Martin, K. Morokuma, V. G. Zakrzewski, G. A. Voth, P. Salvador, J. J. Dannenberg, S. Dapprich, A. D. Daniels, O. Farkas, J. B. Foresman, J. V. Ortiz, J. Cioslowski and D. J. Fox, Gaussian 09, Revision A.02, Gaussian Inc., Wallingford CT, 2009 Search PubMed.
- L. A. Curtiss, P. C. Redfern and K. Raghavachari, Gaussian-4 Theory, J. Chem. Phys., 2007, 126, 084108 CrossRef PubMed.
-
M. J. Frisch, G. W. Trucks, H. B. Schlegel, G. E. Scuseria, M. A. Robb, J. R. Cheeseman, G. Scalmani, V. Barone, B. Mennucci, G. A. Petersson, H. Nakatsuji, M. Caricato, X. Li, H. P. Hratchian, A. F. Izmaylov, J. Bloino, G. Zheng, J. L. Sonnenberg, M. Hada, M. Ehara, K. Toyota, R. Fukuda, J. Hasegawa, M. Ishida, T. Nakajima, Y. Honda, O. Kitao, H. Nakai, T. Vreven, J. J. A. Montgomery, J. E. Peralta, F. Ogliaro, M. Bearpark, E. B. J. J. Heyd, K. N. Kudin, V. N. Staroverov, R. Kobayashi, J. Normand, K. Raghavachari, A. Rendell, J. C. Burant, S. S. Iyengar, J. Tomasi, M. Cossi, N. Rega, J. M. Millam, M. Klene, J. E. Knox, J. B. Cross, V. Bakken, C. Adamo, J. Jaramillo, R. Gomperts, R. E. Stratmann, O. Yazyev, A. J. Austin, R. Cammi, C. Pomelli, J. W. Ochterski, R. L. Martin, K. Morokuma, V. G. Zakrzewski, G. A. Voth, P. Salvador, J. J. Dannenberg, S. Dapprich, A. D. Daniels, O. Farkas, J. B. Foresman, J. V. Ortiz, J. Cioslowski and D. J. Fox, Gaussian 09, Revision D.01, Gaussian Inc., Wallingford CT, 2009 Search PubMed.
- T. J. Wallington, J. M. Andino, I. M. Lorkovic, E. W. Kaiser and G. Marston, J. Phys. Chem., 1990, 94, 3644–3648 CrossRef CAS.
- R. Atkinson, D. L. Baulch, R. A. Cox, J. N. Crowley, R. F. Hampson, R. G. Hynes, M. E. Jenkin, M. J. Rossi and J. Troe, Atmos. Chem. Phys., 2006, 6, 3625–4055 CrossRef CAS.
- R. Søndergaard, O. J. Nielsen, M. D. Hurley, T. J. Wallington and R. Singh, Chem. Phys. Lett., 2007, 443, 199–204 CrossRef.
- M. D. Hurley, J. C. Ball and T. J. Wallington, J. Phys. Chem. A, 2007, 111, 9789–9795 CrossRef CAS PubMed.
- M. Mashino, Y. Ninomiya, M. Kawasaki, T. J. Wallington and M. D. Hurley, J. Phys. Chem. A, 2000, 104, 7255–7260 CrossRef CAS.
- L. L. Andersen, F. F. Østerstrøm, M. P. Sulbaek Andersen, O. J. Nielsen and T. J. Wallington, Chem. Phys. Lett., 2015, 639, 289–293 CrossRef CAS.
- M. P. Sulbaek Andersen, E. J. K. Nilsson, O. J. Nielsen, M. S. Johnson, M. D. Hurley and T. J. Wallington, J. Photochem. Photobiol., A, 2008, 199, 92–97 CrossRef.
- R. J. Meagher, M. E. McIntosh, M. D. Hurley and T. J. Wallington, Int. J. Chem. Kinet., 1997, 29, 619–625 CrossRef CAS.
- Absorption cross section obtained from Dr T. J. Wallington, Ford Motor Company, private communication July 2016.
- T. J. Wallington, M. D. Hurley, O. J. Nielsen and J. Sehested, J. Phys. Chem., 1994, 98, 5686–5694 CrossRef CAS.
- B. Ballesteros, E. Jiménez, A. Moreno, A. Soto, M. Antiñolo and J. Albaladejo, Chemosphere, 2017, 167, 330–343 CrossRef CAS PubMed.
- Y. Diaz-de-Mera, A. Aranda, A. Notario, A. Rodriguez, D. Rodriguez and I. Bravo, Phys. Chem. Chem. Phys., 2015, 17, 22991–22998 RSC.
- M. P. Sulbaek Andersen, O. J. Nielsen, M. D. Hurley, J. C. Ball, T. J. Wallington, J. E. Stevens, J. W. Martin, D. A. Ellis and S. A. Mabury, J. Phys.
Chem. A, 2004, 108, 5189–5196 CrossRef.
- T. J. Wallington and M. D. Hurley, Int. J. Chem. Kinet., 1993, 25, 819–824 CrossRef CAS.
-
J. G. Calvert, J. N. Pitts Jr., Photochemistry, John Wiley and Sons Inc., New York, 1966 Search PubMed.
-
B. J. Finlayson-Pitts, J. N. Pitts Jr., Kinetics and Atmospheric Chemistry, Chemistry of the Upper and Lower Atmosphere, Academic Press, San Diego, 2000, ch. 5, pp. 130–178 Search PubMed.
-
B. J. Finlayson-Pitts, J. N. Pitts Jr., Rates and Mechanisms of Gas-Phase Reactions in Irradiated Organic–NOx–Air Mixtures, Chemistry of the Upper and Lower Atmosphere, Academic Press, San Diego, 2000, ch. 6, pp. 179–263 Search PubMed.
- R. Prinn, J. Huang, R. Weiss, D. Cunnold, P. Fraser, P. Simmonds, A. McCulloch, C. Harth, P. Salameh and S. O'Doherty, Science, 2001, 292, 1882–1888 CrossRef CAS PubMed.
- W. Allan, H. Struthers, D. C. Lowe and S.E. Mikaloff Fletcher, J. Geophys. Res.: Atmos., 2010, 115, 8 CrossRef.
- C. M. Spivakovsky, J. A. Logan, S. A. Montzka, Y. J. Balkanski, M. Foreman-Fowler, D. B. A. Jones, L. W. Horowitz, A. C. Fusco, C. A. M. Brenninkmeijer, M. J. Prather, S. C. Wofsy and M. B. McElroy, J. Geophys. Res.: Atmos., 2000, 105, 8931–8980 CrossRef CAS.
- S. Pinnock, M. D. Hurley, K. P. Shine, T. J. Wallington and T. J. Smyth, J. Geophys. Res.: Atmos., 1995, 100, 23227–23238 CrossRef CAS.
- Ø. Hodnebrog, M. Etminan, J. S. Fuglestvedt, G. Marston, G. Myhre, C. J. Nielsen, K. P. Shine and T. J. Wallington, Rev. Geophys., 2013, 51, 300–378 CrossRef.
-
IPCC
, Climate Change 2013: The Physical Science Basis. Contribution of Working Group I to the Fifth Assessment Report of the Intergovernmental Panel on Climate Change, Cambridge University Press, Cambridge, United Kingdom and New York, NY, USA, 2013, p. 1535 Search PubMed.
- N. R. Greiner, J. Chem. Phys., 1968, 48, 1413 CrossRef CAS.
- G. Paraskevopoulos and W. S. Nip, Can. J. Chem., 1980, 58, 2146–2149 CrossRef CAS.
- T. Gierczak, M. Baasandorj and J. B. Burkholder, J. Phys. Chem. A, 2014, 118, 11015–11025 CrossRef CAS PubMed.
- V. C. Papadimitriou and J. B. Burkholder, J. Phys. Chem. A, 2016, 120, 6618–6628 CrossRef CAS PubMed.
Footnote |
† Electronic supplementary information (ESI) available. See DOI: 10.1039/c6cp07234h |
|
This journal is © the Owner Societies 2017 |