A database on the stability of silver and gold nanostructures for applications in biology and biomolecular sciences†
Received
8th September 2016
, Accepted 23rd October 2016
First published on 8th November 2016
Abstract
Colloidal stability of nanoparticles in biological media is an important consideration when trying to ensure reliable data interpretation of in vitro and in vivo systems. We have developed a detailed colloidal stability library of newly synthesized gold, silver and gold-core silver-shell plasmonic nanoparticles, stabilized with aspartame, glucosamine and sucralose, in various biologically relevant buffers and bacterial and mammalian cell culture media. The stabilizer selection reflects the preference for molecules that are non-toxic, inexpensive, readily available, water soluble and easy-to-replace if that is the end-user preference. An on-line resource provides detailed stability information on each of the 81 systems examined. To illustrate how to utilize this stability library, we conducted bacterial toxicity and biocompatibility experiments through the use of one specific set of nanomaterials in the presence and absence of plasmonic irradiation.
Introduction
Noble metal nanostructures have become very useful in biology and medicine, ranging from diagnostics,1–3 drug delivery,4–6 therapies involving nanomaterials,7–9 tumour treatment,10 and numerous catalytic processes both in vitro and in vivo.11–13 The diversity of materials with different size, morphology, polydispersity and surface stabilizers make it difficult for researchers to find or describe materials for a given application,14,15 or for regulatory agencies to find what is acceptable in the context of environmental, medical or cosmetic applications. In a recent call for “Nanotechnology – Improved Grand Challenges”, the US government proposed one of the goals for 2015 to be “Determine the environmental, health, and safety characteristics of a nanomaterial in a month”.16 As of 2016, this appears to be a challenging and highly optimistic goal, but it also suggests the need to establish well-characterized libraries of materials, which can be screened for potential applications and would provide an optimal starting point in order to fast-track the evaluation of nanomaterials.
There are numerous variations in nanoparticles (NP) being produced across various laboratories. For this reason, in order to screen the suitability of NP for in vitro studies, it is essential to consider factors such as size, morphology, capping agent(s) and stability in biologically relevant media. For example, cellular uptake of NP is highly controlled by a particular size17,18 and in order to obtain reliable and repeatable findings, monodisperse samples are frequently preferred. Another important factor is the surface charge produced by the capping agent (stabilizer), because it can dictate the stability and behaviour of the NP upon changing microenvironment and molecular interactions.19,20 Although the aforementioned parameters each serve an important function, it is critical to preserve the NP stability in biological media, as instability of any kind can result in undesirable or unpredictable biological outcomes. In general, most bacterial and mammalian cell culture media are composed of a cocktail of various buffers, inorganic salts and biomacromolecules. Once dispersed in the biological media, unstable NP may undergo aggregation, dissolution or lead to the undesirable adsorption of biological components present in the media.19,21,22 While this instability can clearly skew results, there are limited examples of research that has taken the liberty of conducting stability testing to evaluate the fate of NP, once exposed to biological media prior to assessing its biological function.15 For these reasons, it is essential to evaluate the NP stability prior to conducting cell experiments in order to observe if the NP integrity is preserved during the experiments.
In this study, we explore the stability, biocompatibility, and antibacterial capacity of spherical metallic NP consisting of gold and silver, as well as some core–shell bimetallic structures. We have excluded copper, as earlier work suggests it dissolves readily to Cu(II),23 and does not appear to have the nanoparticulate stability that we deemed as necessary for inclusion in this research. As far as surface stabilizers, we have concentrated on well-characterized small molecules that we know to be water compatible and non-toxic. Of the tested capping agents, common sweeteners, such as aspartame and sucralose proved to be good choices, as well as glucosamine, a common nutritional supplement and a main derivative in Gram (+) bacterial metabolic pathways.24 Preliminary experiments with saccharin were not very promising, while steviol proved to be too expensive and hard to obtain with acceptable purity. We have also avoided common surface stabilizers that contain S–H or S–S bonds, as we anticipate that many library users may choose to substitute the surface stabilizer (e.g., aspartame) for other materials that are relevant to their own research or application; thus, we have preferred easy-to-replace stabilizers.
Keeping in mind that our library targets biological applications, we have tested their stability in various media, starting with water, saline solution, various buffers such as Dulbecco's phosphate buffer saline (DPBS) and 4-(2-hydroxyethyl)-1-piperazineethanesulfonic acid (HEPES) buffer. We also incorporated some of the more commonly used growth media for bacteria such as Luria Bertani (LB), Mueller Hinton (MH) and Minimal Salts (M9) media, and eukaryotic growth media such as Dulbecco's Modified Eagle Medium (DMEM) and Keratinocyte Basal Medium (KBM).
Experimental
Methodology
We have examined the stability of the nanostructures as a function of stabilizer/capping agent and media by monitoring the loss of the plasmon absorption that characterizes these noble metal nanostructures. In order to easily classify the NP as being stable in a particular media it was necessary to define an acceptable degradation threshold, from which one could easily classify the materials as either being stable or unstable. As such, we decided that solutions retaining ≥80% of their initial plasmon absorption would be considered stable for a selected period of time. It is for this reason we have summarized the timescale of stability of the various nanomaterials, as the time it takes for a 20% decrease in plasmon absorption at the wavelength on their initial maximum absorbance. While this information can be used as a preliminary screening tool, detailed kinetic and spectroscopic data for each material are provided either in the main body of this article or as ESI;† further, we developed an on-line resource where detailed stability information is readily available. For a select group of these nanomaterials, we also tested their bacterial toxicity and biocompatibility (EC50) in the presence and absence of plasmon irradiation.
Chemicals used
All reagents used were of analytical grade. Silver nitrate – 99% (AgNO3), sodium borohydryde (NaBH4), sodium chloride (NaCl), chloroauric acid (HAuCl4·3H2O), sucralose, glucosamine hydrochloride, N-2-hydroxyethyl piperazine-N-2-ethane sulphonic acid (HEPES), Luria-Bertani (LB) broth, Mueller Hinton (MH) broth, Dulbecco's phosphate buffer saline (DPBS), tyrosine, Dulbecco's Modified Eagle's media (DMEM) without phenol red were all purchased from Sigma-Aldrich. Aspartame was purchased from Supelcore and keratinocyte serum free medium (KBM) was purchased from Lonza (Mississauga, ON). 2-Methyl-1-propanone (Irgacure-2959) was a gift from BASF that was supplied by Dempsey Corporation Canada. Sodium chloride was purchased from Alfa Aesar. Milli-Q deionized water (18.2 MΩ) was used throughout all the experiments.
Silver nanoparticle synthesis
Three silver nanoparticle suspensions were synthesized utilizing a simple benchtop protocol25 with minor modifications using NaBH4(aq) as the reducing agent and aspartame (Asp), glucosamine (Glu) and sucralose (Suc) as the stabilizers. Prior to the NP synthesis, all glassware was cleaned by soaking in H2O2
:
H2SO4 (1
:
3). All the reagents were freshly prepared prior to the synthesis. Initially, a 15 mL solution consisting of 3.0 mM NaBH4(aq) and 1.0 mM aspartame, 1.5 mM glucosamine and 1.5 mM sucralose was prepared for the synthesis of AgNP@Asp, AgNP@Glu and AgNP@Suc respectively. The AgNP synthesis was initiated with the addition 15 mL of 0.5 mM AgNO3(aq) to the previous reaction mixture, under continuous stirring in a magnetic stir plate. With the exception of the aspartame batch, prior to adding the AgNO3(aq), the pH of the aqueous solution containing NaBH4 and aspartame was adjusted to 8.3, by adding 0.1 M HCl(aq) as needed. The reaction mixtures were then further stirred vigorously on a magnetic stir plate for 2 h, leading to the stable bright yellow colour characteristic of AgNP. The AgNP were left in the dark for approximately 24 h for further stabilization (ripening).
Gold nanoparticle synthesis
Three AuNP colloidal suspensions reduced and stabilized with aspartame, glucosamine and sucralose were synthesized at room temperature with minor modifications to the previously published protocol by Qin et al.26 Prior to the nanoparticle synthesis, all glassware were cleaned by soaking in H2O2
:
H2SO4 (1
:
3). A solution (50 mL) containing aspartame (10 mM), glucosamine (10 mM) and sucralose (50 mM) was prepared and the pH was adjusted to 11, by adding 1.0 M NaOH(aq). Finally, 200 μL of 50 mM HAuCl4(aq) solution was added to the aqueous stabilizer solution under continuous stirring. Initially the solution remained colourless, following which it turned red slowly (aspartame – 12 h, glucosamine – 1 h, and sucralose – 10 min), indicating the formation of AuNP. The sucralose capped AuNP were only stirred for 2 h and allowed to further stabilized in the dark for 24 h. The aspartame and the glucosamine stabilized gold nanoparticles were continuously stirred for 48 h, and allowed to further stabilize in the dark for 24 h.
Gold–silver core–shell nanoparticle synthesis
The protocol for the gold–silver core–shell structures is based on an established protocol27 with some minor modifications. Prior to the nanoparticle synthesis, all the glassware were cleaned by soaking in H2O2
:
H2SO4 (1
:
3).
AuNP@Ag@Asp synthesis.
333 μL of AuNP seeds (∼12 nm), produced using Irgacure-2959 (I-2959) reduction, 116.7 μL of 10 mM AgNO3(aq), 116.7 μL of 10 mM I-2959(aq) and 14 μL of 4 mM aspartame(aq) were mixed together and brought up to a final volume 7000 μL with Chellex Milli-Q deionized water in a quartz tube.
AuNP@Ag@Glu synthesis.
The glucosamine capped gold–silver core–shell nanoparticles were synthesized based on the protocol mentioned above, but with the following modifications: 105.0 μL of 10 mM AgNO3(aq), 94.0 μL of 10 mM I-2959(aq) and 28.0 μL of 4 mM glucosamine(aq).
AuNP@Ag@Suc synthesis.
The sucralose capped gold–silver core–shell nanoparticles were synthesized based on the protocol mentioned above, but with the following modifications: 116.7 μL of 10 mM AgNO3(aq), 94.0 μL of 10 mM I-2959(aq) and 18.7 μL of 4 mM sucralose(aq).
Finally, the aqueous solution mixtures were purged with N2(g) for 1 h and irradiated with UVA light, using a Luzchem LZC-4 photoreactor, for 30 min. The synthesized NP were left in dark for 24 h for further stabilization.
Nanoparticle characterization
The nanomaterials synthesized were characterized using various spectroscopic techniques, including ultraviolet-visible (UV-Vis) spectroscopy, dynamic light scattering (DLS), zeta potential and transmission electron microscopy (TEM). UV-Vis measurements were recorded using the SanDrop spectrophotometer (AnalytikJena), using a 1 cm path length quartz cuvette. The hydrodynamic diameters and zeta potential of the nanomaterials were recorded using a Malvern Zetasizer Nano ZS, using disposable 1 cm path length cuvettes and disposable 0.5 mL folded capillary cuvette (Malvern), for DLS and zeta potential, respectively. The samples for TEM analysis were prepared by adding a 25 μL drop of the nanoparticles to the copper side of the carbon-coated copper grid and dried under vacuum overnight. The TEM images were obtained using JSM-7500F FESEM (Jeol).
Media preparation and stability measurements
All media were freshly prepared prior to conducting the stability experiments; 12.5% dilutions of DPBS and saline were used, as preliminary experiments revealed instantaneous instability of NP dispersed in these media. DMEM without phenol red was supplemented with 10% FBS. In all cases, the NP were dispersed into relevant media 1
:
3 (v/v). The stability measurements were conducted based on the change in plasmon absorbance maxima (Absmax) at different time points, where Absmax at different time points were normalized based on Absmax at t0. The stability measurements were conducted for 7 days for NP dispersed in water, DPBS, saline and HEPES buffer. In order to avoid contamination, the stability measurement for NP dispersed in LB, MH, M9, DMEM and KBM cell culture media were conducted under sterile conditions, for a period of 3 days. All media composition is shown in Table S1.†
Antibacterial capacity
The antimicrobial properties of the aspartame, glucosamine and sucralose stabilized gold and silver nanoparticles, AgNO3 and chloroauric solution were evaluated in S. epidermidis (SE19) and P. aeruginosa (PA14). An overnight culture of each bacterium in LB was diluted to a cell density of ∼106 CFU mL−1 in PBS. Afterwards, in each well of a 96 well plate, 100 μL of bacterial suspension and 100 μL of the tested solutions were mixed softly in a shaker. The well plate was irradiated at 37 °C during 18 h with a Luzchem Expo panel populated by 525 nm LEDs. Every 1 h, aliquots of each samples were diluted properly and seeded in MH agar plates. CFU were counted from the agar by quadruplicated after 18 h of incubation at 37 °C, and the log of CFU mL−1 were calculated for each sample with the corresponding SD. An identical wellplate was kept in the dark as a control.
Eukaryotic biocompatibility
The in vitro biocompatibility of AuNP@Suc was evaluated using NIH/3T3 fibroblast cell line (CRL-1658, ATCC, USA). The fibroblast cells were maintained in Dulbecco's Modified Eagle Medium supplemented with 10% fetal bovine serum, 1% penicillin/streptomycin and 0.1% gentamycin and cultured at 37 °C, 5.0% CO2 and 100% humidity. The cell viability was evaluated using CellTiter 96 Aqueous MTS colorimetric assay, where the cell survival of 5 × 103 cells seeded in 96 well-plate incubated with NP for 12 h. All the NP used were washed prior to cellular incubation. Varying concentrations of serial dilutions of the AuNP@Suc were incubated in the presence and absence of illumination from a Luzchem Expo panel containing 525 nm LEDs. The media containing the nanomaterials was then rinsed with PBS and replaced with 75 μL of supplemented DMEM without phenol red and 15 μL of MTS. The absorbance at 490 nm was measured to evaluate the cell viability by comparing to the control, after incubation for 2 h.
Results and discussion
Synthesis and characterization
NaBH4 can efficiently reduce Au3+ and Ag+ to produce relatively monodisperse NP.25 In this study, silver nanoparticles (AgNP) were synthesised using NaBH4 as a reducing agent in a one-pot method using AgNO3 as the precursor salt in the presence of stabilizers such as aspartame, glucosamine and sucralose. A simple relationship was found between the concentration of stabilizer and the intensity of the plasmon band. An optimal concentration of the stabilizer (500 μM aspartame, 750 μM glucosamine and 750 μM sucralose) was selected for the AgNP synthesis for the remainder of the study. After letting the AgNP undergo Ostwald ripening for 24 hours, we observed plasmon absorption maxima centred at 393.5, 391.0, and 393.5 nm (all ± 0.5 nm) for AgNP@Asp, AgNP@Glu and AgNP@Suc, respectively (Fig. 1 top). Moreover, the UV-Vis spectra of these AgNP indicated that they are stable for at least 7 days (Fig. S1†). In contrast to the AgNP, the gold nanoparticles (AuNP) were synthesized without NaBH4. Using HAuCl4 as precursor, the Au was both reduced and stabilized utilizing aspartame, glucosamine and sucralose respectively at room temperature. This relies on the fact that the stabilizers utilized serve as mild reducing agents, as they incorporate electron rich –OH, –NH2 functional moieties. The fact that the Au precursor can be reduced under ambient conditions by the stabilizers alone, while the Ag salt requires NaBH4 can be easily explained by their corresponding reduction potentials. The higher electropositive reduction potential of Au3+ (E° = 1.000)28 in comparison to Ag+ (E° = 0.799),28 indicates that it is more easily reduced. The initiation and manipulation of the reaction kinetics of this reduction process were achieved with the addition of NaOH.29 It should also be noted that both AuNP@Asp and AuNP@Glu took 48 h to achieve complete reduction, whereas AuNP@Suc took only 2 h. This may be due to the complex formation between the Cl− and gold, which could enhance the ripening process.30 After letting the colloidal solutions ripen for 1 day, we observed plasmon maxima centred at 520.0, 522.5, 518.5 nm for AuNP@Asp, AuNP@Glu and AuNP@Suc, respectively (Fig. 1B).
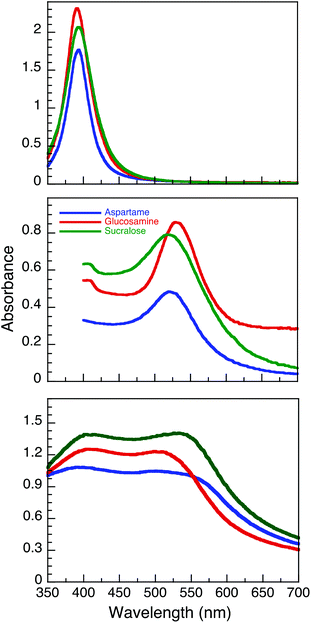 |
| Fig. 1 UV-Vis spectra of AgNP (top), AuNP (middle) and AuNP@Ag (bottom) functionalized with aspartame, glucosamine and sucralose. The spectra were taken 24 h post synthesis. | |
Our group had previously published a novel method for synthesizing aspartame stabilized gold–silver core–shell nanoparticles (AuNP@Ag@Asp),27 where both Au3+ and Ag+ were sequentially reduced in the presence of UVA light with Irgacure-2959. Utilizing the same protocol, we were able to synthesize glucosamine (AuNP@Ag@Glu) and sucralose (AuNP@Ag@Suc) stabilized gold–silver core–shell NP. Two strong peaks with similar intensities corresponding to silver and gold plasmon absorptions can be observed at 390.0/501.0, 406.0/509.0, 402.5/533.0 nm for AuNP@Asp, AuNP@Glu and AuNP@Suc respectively, post ripening (Fig. 1C).
In order to evaluate the morphology, size and surface stability of the nine NP synthesized, TEM, DLS and zeta potential measurements were conducted and are summarized in Table 1. TEM images (Fig. 2) obtained for all NP, revealed their spherical nature and relatively uniform size distribution. The sizes obtained from DLS measurements are also in good agreement with the TEM measurements, as larger DLS dimensions are normally anticipated given that this technique measures hydrodynamic size and not just core dimensions.
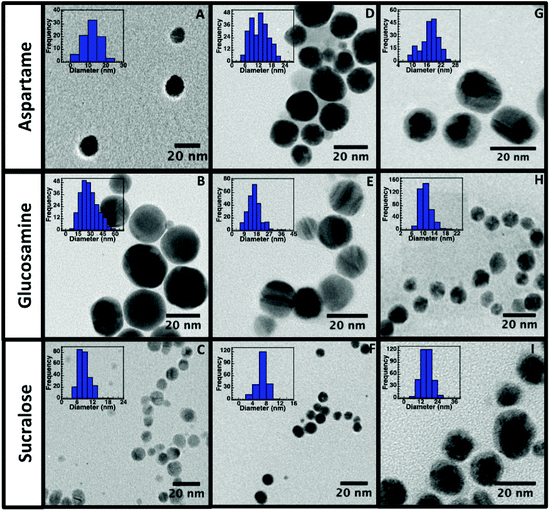 |
| Fig. 2 Representative transmission electron microscopy images AgNP (A–C), AuNP (D–F) and AuNP@Ag (G–I) stabilized with aspartame (A, D, G), glucosamine (B, E, H) and sucralose (C, F, I). The scale bar corresponds to 20 nm. The insets represent the corresponding NP size distribution (n > 250). | |
Table 1 Physical characterization summary of the nine nanomaterials synthesized
Nanoparticle |
Capping agent |
Aspartame |
Glucosamine |
Sucralose |
AgNP
|
TEM diameter (nm) |
11.8 ± 4.4 |
28.3 ± 9.9 |
8.7 ± 2.3 |
DLS diameter (nm) |
46.9 ± 1.3 |
56.9 ± 0.1 |
22.3 ± 0.3 |
ζ potential (mV) |
−57.9 ± 1.2 |
−35.5 ± 3.2 |
−37.0 ± 1.8 |
|
AuNP
|
TEM diameter (nm) |
12.4 ± 4.0 |
16.5 ± 5.2 |
6.8 ± 1.5 |
DLS diameter (nm) |
26.1 ± 2.4 |
42.0 ± 0.3 |
16.7 ± 0.2 |
ζ potential (mV) |
−47.3 ± 1.5 |
−47.4 ± 1.5 |
−26.6 ± 1.5 |
|
AuNP@Ag
|
TEM diameter (nm) |
17.8 ± 4.3 |
10.7 ± 2.1 |
16.0 ± 4.1 |
DLS diameter (nm) |
66.3 ± 1.0 |
47.4 ± 0.3 |
70.3 ± 1.0 |
ζ potential (mV) |
−43.1 ± 0.3 |
−35.8 ± 0.9 |
−42.8 ± 0.9 |
Both TEM and DLS measurements also confirm that sucralose stabilized AgNP (Fig. 2C) and AuNP (Fig. 2F) yielded the smallest particles. As the same amount of reducing agent, NaBH4, was used in all of the AgNP synthesis, the presence of sucralose might have a synergistic effect during the reduction process or during the coalescence step of the NP growth. Sucralose may also be a better stabilizer in comparison to the other stabilizers, which could ultimately result in minimal aggregation. The TEM images obtained for AuNP@Ag (Fig. 2G–I), reveals a darker core region, corresponding to the Au core, surrounded by a lighter region of Ag, where AuNP@Ag@Glu were found to be the smallest and more uniform out of all three stabilizers used. As seen in Table 1, zeta-potential analysis conducted on all nine nanomaterials had electronegative charges lower than −20 mV confirming its stability by electrostatic repulsion. It is also important to note that zeta potential values above and below the range of +20 mV to −20 mV are considered stable colloidal solutions, as the higher Coulombic repulsion inhibits NP aggregation.
Colloidal stability in biological media
In biological environments, colloidal stability is significantly dependent on the intrinsic properties of the NP, such as the surface functionalization and charge, in addition to the ionic strength, pH and protein composition of the specific biological media.19,21,31,32 We have evaluated the NP stability and the corresponding time-dependent plasmon evolution of NP dispersed in relevant media, utilizing UV-Vis spectrophotometry. Transformations in the UV-Vis spectra were then assessed for 7 days for NP dispersed in water, DPBS, saline and HEPES buffer and for 3 days for NP dispersed in LB, MH, M9, DMEM and KBM cell culture media. We then evaluated the progression of the plasmon maxima and shift at various time points. The stability of the NP were determined based on the following equation: | 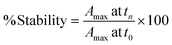 | (1) |
We have presented the time dependent UV-Vis spectra along with the time it took for the NP plasmon to decrease by 20% once dispersed in each media. For clarification purposes, although two plasmons corresponding to both Au and Ag were found for the AuNP@Ag samples, the plasmon band that decreased by 20% initially was selected for stability measurements. The data are summarized in Fig. 5 and the downloadable tool available.
UV-Vis spectra of the NP dispersed in water show that all nine NP were found to be highly stable (Fig. S4.1†). However, the plasmon for AgNP red-shifted by 3.3 nm, 7.5 nm and 6.7 nm for AgNP@Asp, AgNP@Glu and AgNP@Suc, respectively, indicating slow aggregation occurring for a smaller population after 7 days. Nevertheless, the plasmon absorption intensity did not significantly decrease. Thus, we can consider these AgNP to be relatively stable. AgNP and AuNP@Ag dispersed in saline solution (Fig. S4.5†) were found to be very unstable, where a similar trend was noted for the AgNP dispersed in PBS (Fig. S4.3†), with the exception of AgNP@Suc. Saline and PBS have relatively higher concentrations of monovalent counter ions, such as Na+ and Cl− (see Table S1†). The stabilizers used here are weakly bound to the NP surface and the AgNP destabilization can be therefore accelerated in the presence of Cl−, resulting in the shielding of the surface charge and the formation of a AgCl precipitate (thus compromising the integrity of AgNP).33,34 HEPES, another commonly used buffer for mammalian cell culture was found to be a very stable buffer for most of the NP, with the exception of AgNP@Asp (t0.8-stability = 14.5 h), see Fig. S4.8.†
Of all three bacteriological media, M9 was found to be the most stable media (Fig. 4), with the exception of AgNP@Asp being the least stable, where the plasmon was red-shifted and broadened over time. M9 media contains minimal nutrients available for bacterial growth35 and has a composition of relatively low ionic strength, with limited biological molecules such as proteins and sugars, in comparison to LB and MH. In the presence of LB, the NP plasmon was red-shifted for the NP that had a slower destabilization process (Fig. S4.9†), attributed to biomacromolecular adsorption and slow aggregation. AgNP@Asp and AuNP@Suc were the only samples found to be stable in LB, nevertheless a slight plasmon red-shift of 7.2 nm and 6.0 nm respectively was still observed for those NP A distinctive point to address is that AuNP@Asp, AuNP@Glu and all three AuNP@Ag colloidal samples crashed instantaneously upon dispering in LB. MH is also a media composed of high concentrations of proteins, such as casein. The majority of NP dispersed in MH resulted in destabilization (Fig. S4.11†), with the exception of AuNP@Asp and AuNP@Suc. The plasmon peak of all three AgNP samples significantly decreased and red-shifted, which could be attributed to the competitive process between ligand assisted AgNP dissolution and protein adsorption.19,36 It is critical to note that the Ag plasmon corresponding to AuNP@Ag disappeared in the presence of MH, confirming its destabilizing effect on Ag.
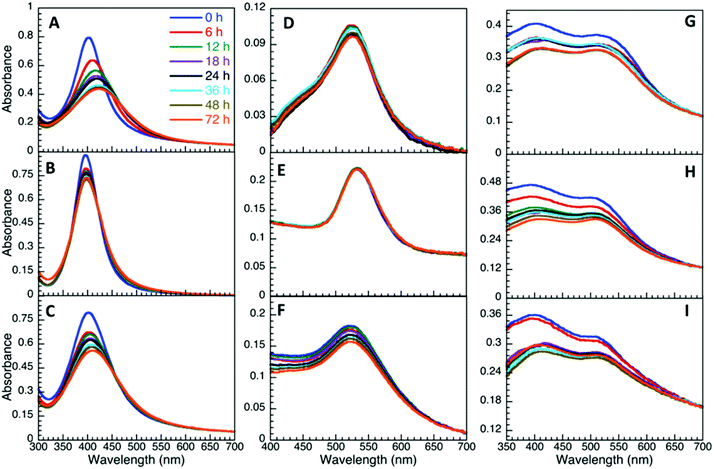 |
| Fig. 3 Time evolution of UV-Vis spectra of AgNP (A–C), AuNP (D–F) and AuNP@Ag (G–I) stabilized with aspartame (A, D, G), glucosamine (B, E, H) and sucralose (C, F, I) dispersed in M9 (1 : 3 v/v). | |
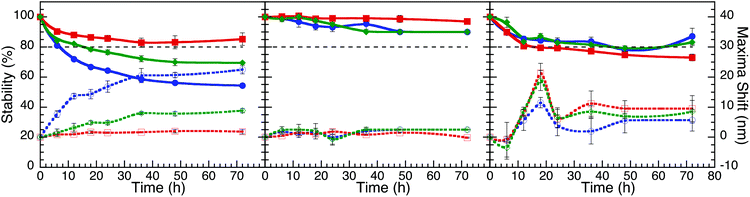 |
| Fig. 4 Stability curves and plasmon shifts of AgNP (A), AuNP (B) and AuNP@Ag (C) stabilized with aspartame (blue), glucosamine (red) and sucralose (green), dispersed in M9 media (1 : 3 v/v). These plots are based on the data from Fig. 3. | |
Eukaryotic media such as DMEM and KBM are composed of varying amounts of proteins, sugars and counter-ions, which upon mixing could further alter the NP fate (refer to Fig. 5). In the presence of DMEM containing 10% fetal bovine serum (FBS), all three AuNP samples were found to be the most stable. However, a significant broadening and red-shifting of the plasmon was observed on the AuNP@Suc, which could be a result of serum adsorption. The serum adsorption was predominant on all three AgNP samples, where a substantial plasmon red-shift and decreased intensity was observed over 72 hours. AuNP@Ag showed to have intermediate stability attributes. We also reported the NP stability in KBM, which had no serum, therefore the effect of the counter-ions present in the medium should have had a significant impact on the NP stability.21,31 An instantaneous destabilizing effect on all three AuNP@Ag was observed upon media dispersion, validated by the observation of the instantaneous colour change. As for the AgNP and AuNP samples, aggregation was evident as the plasmon began to broaden over time. Interestingly, a new peak emerged at longer wavelength (>550 nm) for all three AgNP batches revealing the heterogeneous aggregation process.
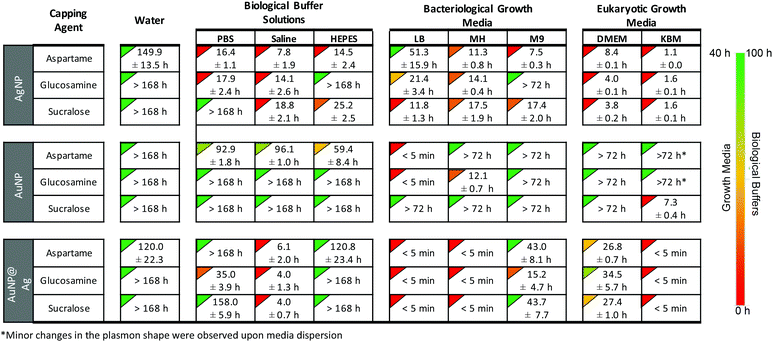 |
| Fig. 5 Image of the home page for the downloadable web-tool available as ESI,† showing the relative stability times (t0.8-stability) of AgNP, AuNP and AuNP@Ag functionalized with aspartame, glucosamine and sucralose in various buffer solutions and growth media. The NP were dispersed in media 1 : 3 (v/v) and the time it took for the plasmon maxima to decrease by 20% is shown below. The complete kinetic and spectroscopic stability data of the 81 NP-media combinations are available in the ESI.† We have also created an open-access interactive tool for viewing the complete stability data that can be downloaded as ESI.† | |
Biocompatibility and antibacterial capacity
The aforementioned, NP can undergo undesirable changes in some of the media tested, resulting in modified biological interactions,36 consequently altering its cellular uptake31 and specific toxicity.15,37 In order to evaluate one example of the use of the stability library compiled in this report, we examined the AuNP@Glu biocompatibility and antibacterial capacity. Based on the stability library (refer to Fig. 5), DMEM and DPBS were the choices of media for the biocompatibility and microbiological assays respectively. Cell viability on 3T3 fibroblast cells was conducted by incubating AuNP@Suc in the presence and absence of 530 nm irradiation. No significant cytotoxicity was observed at various concentrations in the absence of plasmon irradiation (65C). However, in the presence of 530 nm irradiation, concentrations above 1.2 μg mL−1 (4 to 25 times higher than the one needed to achieve the antibacterial effect) were found to be toxic and are attributed to the photothermal effect of AuNP.38,39 No significant toxicity was seen in the controls conducted using 5 mM sucralose in the presence and absence of light, meaning that this small molecule is indeed a biocompatible stabilizer agent.
All NP proved to have antibacterial capacity once they were irradiated at 530 nm. Once more, just to mention one example, Time Kill Curves results for AuNP@Suc are plotted in Fig. 6. While the number of CFU mL−1 remained constant in controls of S. epidermidis and P. aeruginosa with and without AuNP@Suc kept in the dark, the “light activated” NP score a complete inhibition of growth for both strains in a few hours; it is relevant to note that the suspensions irradiated but not treated with NP were not affected, ruling out any light interference on bacterial growth (Fig. 6A and B). These results agree with the current literature, which also provide evidence of the location of this type of NP either on the lipopolysaccharide membrane or in the inner bacteria medium.40,41
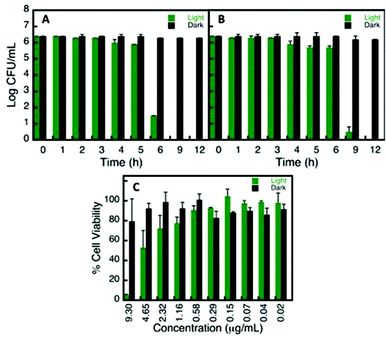 |
| Fig. 6 Biocompatibility and antimicrobial data of AuNP@Suc in presence and absence of 530 nm irradiation. The amount of S. epidermidis (A) and P. aeruginosa (B) survival colonies were counted in the presence of 0.37 μg mL−1 post 18 h incubation at 37 °C on agar plates. The experiment was carried out in PBS. Cell viability assay of NIH/3T3 fibroblast cells (C) in the presence of varying concentration of AuNP@Suc post 12 h incubation. | |
The similarities between the results found for S. epidermidis and P. aeruginosa suggest that the photoantibacterial effect of these NP it is largely independent of the characteristics of the strains, since these two are very different types of bacteria. The first one is a Gram positive coccus that normally need a rich media to growth, and the second one is a Gram negative bacillus capable of growing almost anywhere, even in plain water or dry surfaces.42 The potential anti-infective applications are therefore very promising and we anticipate that will stimulate further work in the field.
Conclusion
We have presented a detailed stability summary of gold and silver nanomaterials, functionalized with aspartame, glucosamine and sucralose. The key result is a library of 81 combinations of nanostructure, stabilizer and media providing future researchers in the field a convenient tool to identify systems that may be best suited for their own nanoscience projects. The motivation for this work was our own search for suitable combinations of materials for our projects, eventually leading to the completion of the systems in Fig. 5; of these 29 are labeled in red and clearly not suitable, but two thirds of the systems show some level of stability.
We have also demonstrated how researchers in the future can use this information to conduct reliable in vitro and microbiological assays. Ultimately, we are hoping that the stability information obtained from this study can be utilized as a pre-screening tool prior for conducting future studies on potential biomedical applications.
Acknowledgements
We thank the Natural Sciences and Engineering Research Council of Canada, the Canada Research Chairs Program and the Canada Foundation for Innovation for generous support.
References
-
R. Franco, P. Pedrosa, F. F. Carlos, B. Veigas and P. V. Baptista, Handbook of Nanoparticles, 2016, p. 1339 Search PubMed.
- A. Kumar, B. M. Boruah and X.-J. Liang, J. Nanomater., 2011, 2011, 22 Search PubMed.
- C.-W. Yen, H. de Puig, J. O. Tam, J. Gómez-Márquez, I. Bosch, K. Hamad-Schifferli and L. Gehrke, Lab Chip, 2015, 15, 1638 RSC.
- T. Lajunen, L. Viitala, L.-S. Kontturi, T. Laaksonen, H. Liang, E. Vuorimaa-Laukkanen, T. Viitala, X. Le Guével, M. Yliperttula and L. Murtomäki, J. Controlled Release, 2015, 203, 85 CrossRef CAS PubMed.
- F. Benyettou, R. Rezgui, F. Ravaux, T. Jaber, K. Blumer, M. Jouiad, L. Motte, J.-C. Olsen, C. Platas-Iglesias and M. Magzoub, J. Mater. Chem. B, 2015, 3, 7237 RSC.
- A. Kumar, H. Ma, X. Zhang, K. Huang, S. Jin, J. Liu, T. Wei, W. Cao, G. Zou and X.-J. Liang, Biomaterials, 2012, 33, 1180 CrossRef CAS PubMed.
- G. Von Maltzahn, J.-H. Park, K. Y. Lin, N. Singh, C. Schwöppe, R. Mesters, W. E. Berdel, E. Ruoslahti, M. J. Sailor and S. N. Bhatia, Nat. Mater., 2011, 10, 545 CrossRef CAS PubMed.
- E. Alarcon, B. Vulesevic, A. Argawal, A. Ross, P. Bejjani, J. Podrebarac, R. Ravichandran, J. Phopase, E. Suuronen and M. Griffith, Nanoscale, 2016, 8, 6484 RSC.
- M. Vignoni, H. de Alwis Weerasekera, M. J. Simpson, J. Phopase, T.-F. Mah, M. Griffith, E. I. Alarcon and J. C. Scaiano, Nanoscale, 2014, 6, 5725 RSC.
- R. Xing, K. Liu, T. Jiao, N. Zhang, K. Ma, R. Zhang, Q. Zou, G. Ma and X. Yan, Adv. Mater., 2016, 28, 3669 CrossRef CAS PubMed.
- J. P. M. Almeida, A. L. Chen, A. Foster and R. Drezek, Nanomedicine, 2011, 6, 815 CrossRef CAS PubMed.
- F. Cavalieri, M. Tortora, A. Stringaro, M. Colone and L. Baldassarri, J. Hosp. Infect., 2014, 88, 183 CrossRef CAS PubMed.
- W. Q. Lim and Z. Gao, Nano Today, 2016, 11, 168 CrossRef CAS.
- X. Zhou, W. Xu, G. Liu, D. Panda and P. Chen, J. Am. Chem. Soc., 2009, 132, 138 CrossRef PubMed.
-
H. de Alwis Weerasekera and M. Griffith and E. I. Alarcon, in Silver Nanoparticle Applications, Springer, 2015, p. 93 Search PubMed.
-
L. Whitman, A Call for Nanotechnology-Inspired Grand Challenges, https://www.whitehouse.gov/blog/2015/06/17/call-nanotechnology-inspired-grand-challenges, Accessed August 15, 2016.
- B. D. Chithrani, A. A. Ghazani and W. C. Chan, Nano Lett., 2006, 6, 662 CrossRef CAS PubMed.
- C. He, Y. Hu, L. Yin, C. Tang and C. Yin, Biomaterials, 2010, 31, 3657 CrossRef CAS PubMed.
- J. S. Gebauer, M. Malissek, S. Simon, S. K. Knauer, M. Maskos, R. H. Stauber, W. Peukert and L. Treuel, Langmuir, 2012, 28, 9673 CrossRef CAS PubMed.
- I. Lynch and K. A. Dawson, Nano Today, 2008, 3, 40 CrossRef CAS.
- Z. Ji, X. Jin, S. George, T. Xia, H. Meng, X. Wang, E. Suarez, H. Zhang, E. M. Hoek and H. Godwin, Environ. Sci. Technol., 2010, 44, 7309 CrossRef CAS PubMed.
- X. Li, J. J. Lenhart and H. W. Walker, Langmuir, 2011, 28, 1095 CrossRef PubMed.
- N. L. Pacioni, V. Filippenko, N. Presseau and J. Scaiano, Dalton Trans., 2013, 42, 5832 RSC.
- A. Imada, Y. Nozaki, F. Kawashima and M. Yoneda, Microbiology, 1977, 100, 329 CrossRef CAS PubMed.
- J. R. Polte, X. Tuaev, M. Wuithschick, A. Fischer, A. F. Thuenemann, K. Rademann, R. Kraehnert and F. Emmerling, ACS Nano, 2012, 6, 5791 CrossRef CAS PubMed.
- G. W. Qin, J. Liu, T. Balaji, X. Xu, H. Matsunaga, Y. Hakuta, L. Zuo and P. Raveendran, J. Phys. Chem. C, 2008, 112, 10352 CAS.
- C. Fasciani, M. J. Silvero, M. A. Anghel, G. A. Argüello, M. C. Becerra and J. C. Scaiano, J. Am. Chem. Soc., 2014, 136, 17394 CrossRef CAS PubMed.
-
P. Vanysek, CRC handbook of chemistry and physics, 1998, p. 87 Search PubMed.
- J. Liu, G. Qin, P. Raveendran and Y. Ikushima, Chem. – Eur. J., 2006, 12, 2131 CrossRef CAS PubMed.
- L. Zhao, X. Ji, X. Sun, J. Li, W. Yang and X. Peng, J. Phys. Chem. C, 2009, 113, 16645 CAS.
- D. Mahl, C. Greulich, W. Meyer-Zaika, M. Köller and M. Epple, J. Mater. Chem., 2010, 20, 6176 RSC.
- E. Casals, T. Pfaller, A. Duschl, G. J. Oostingh and V. F. Puntes, Small, 2011, 7, 3479 CrossRef CAS PubMed.
- J. Liu, D. A. Sonshine, S. Shervani and R. H. Hurt, ACS Nano, 2010, 4, 6903 CrossRef CAS PubMed.
- J. Liu and R. H. Hurt, Environ. Sci. Technol., 2010, 44, 2169 CrossRef CAS PubMed.
- In Cold Spring Harber Protocols, 2010.
- L. V. Stebounova, E. Guio and V. H. Grassian, J. Nanopart. Res., 2011, 13, 233 CrossRef CAS.
- T. Xia, M. Kovochich, J. Brant, M. Hotze, J. Sempf, T. Oberley, C. Sioutas, J. I. Yeh, M. R. Wiesner and A. E. Nel, Nano Lett., 2006, 6, 1794 CrossRef CAS PubMed.
- M. J. Silvero, G. A. Argüello and M. C. Becerra, J. Nanopharm. Drug Deliv., 2014, 2, 148 CrossRef.
- S. Simoncelli, H. de Alwis Weerasekera, C. Fasciani, C. N. Boddy, P. F. Aramendia, E. I. Alarcon and J. C. Scaiano, J. Phys. Chem. Lett., 2015, 6, 1499 CrossRef CAS PubMed.
- X. Li, S. M. Robinson, A. Gupta, K. Saha, Z. Jiang, D. F. Moyano, A. Sahar, M. A. Riley and V. M. Rotello, ACS Nano, 2014, 8, 10682 CrossRef CAS PubMed.
- W.-C. Huang, P.-J. Tsai and Y.-C. Chen, Nanomedicine, 2007, 2, 777 CrossRef CAS PubMed.
- M. Favero, L. Carson, W. Bond and N. Petersen, Science, 1971, 173, 836 CAS.
Footnote |
† Electronic supplementary information (ESI) available: Nanoparticle synthesis, biological media composition and stability. A tabulated database of 81 combinations of particle, media and stability (as in the image of Fig. 5). See DOI: 10.1039/c6bm00629a |
|
This journal is © The Royal Society of Chemistry 2017 |