DOI:
10.1039/C6AY02719A
(Paper)
Anal. Methods, 2017,
9, 134-140
Electrochemical sensor for the facile detection of trace amounts of bisphenol A based on cyclodextrin-functionalized graphene/platinum nanoparticles
Received
30th September 2016
, Accepted 23rd November 2016
First published on 23rd November 2016
Abstract
Bisphenol A (BPA) is an important and widely used industrial chemical that is harmful to both the environment and human health. Graphene/platinum nanoparticles (Gr/PtNPs) functionalized with heptakis-(2,3,6-tri-O-methyl)-β-cyclodextrin (TM-β-CD) were successfully synthesized using a simple wet chemistry strategy. The new nanocomposite had both the excellent properties of the Gr/PtNPs (large surface area, high conductivity and excellent electrocatalytic activity) and the cyclodextrin (host–guest supramolecular recognition and enrichment capability). It had a remarkable synergistic effect on the electrochemical reaction of BPA and a highly sensitive electrochemical sensor was developed to detect BPA. The oxidation peak current of BPA was greatly enhanced at the TM-β-CD-Gr/PtNPs modified glassy carbon electrode (GCE) compared with the Gr/PtNPs/GCE and the TM-β-CD-Gr/GCE. Under the optimum condition, the peak current for BPA increased linearly with concentration in the range 5.0 × 10−8 to 8.0 × 10−5 mol L−1. The detection limit was 1.5 × 10−8 mol L−1 (S/N = 3). This sensor is cost-effective and convenient and shows great potential for the detection of trace amounts of phenol compounds in environmental pollutants.
Introduction
Bisphenol A (BPA; Scheme 1) is an important synthetic organic chemical and is widely used in the production of polycarbonate plastics and epoxy resins.1 It is used in metal food containers, canned beverage drinks and plastic drinking bottles.2 However, as a typical endocrine disruptor, BPA has similar chemical structures to natural oestrogen and can lead to negative effects on health, such as cancer, heart disease or obesity, or abnormalities in liver function.3 Low (parts per billion) concentrations of BPA can damage sexual organs.4 Thus developing a sensitive, fast, simple and cost-efficient method for the determination of BPA is important for both the environment and human health. Various methods have been developed for the detection of BPA, such as gas chromatography-mass spectroscopy,5 high-performance liquid chromatography,6 liquid chromatography-mass spectroscopy,7 capillary electrophoresis,8 fluorescence spectrometry,9 chemiluminescent spectrometry10 and surface-enhanced Raman scattering.11 However, these methods often involve complicated sample pre-treatment, high costs and time-consuming processes. Electrochemical methods, with the advantages of sensitivity, simplicity and accuracy, have attracted considerable attention for the determination of BPA. Modified electrodes have been widely used in the development of BPA sensors. Nanomaterials, such as graphene–ionic liquids,12 rGO–Fc–NH2/AuNPs,2 ZnTsPc/f-GN,13 Tyrs/rGO–Cn,3 Pt/Gr–CNTs14 and RhB-incubated poly-β-CD/EG,15 have been used for the detection of BPA. All of these have demonstrated that the nanomaterials play important parts in constructing highly sensitive BPA sensors. Therefore designing new nanomaterials to further accelerate the development of BPA sensors is a challenging topic.
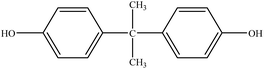 |
| Scheme 1 Chemical structure of BPA. | |
Graphene, a two-dimensional aromatic sheet composed of an sp2-bonded carbon atom monolayer, has attracted much interest since its discovery in 2004.16 It can be used as an electrode material for constructing high-performance electrochemical sensors or biosensors due to its good conductivity, strong mechanical strength, large specific surface area and electrocatalytic activity.17 Many important advances have shown that the innovative combination of graphene with metal nanoparticles (NPs) opens up new ways of utilizing graphene-based hybrid nanomaterials as enhanced materials to construct new, high-performance electrochemical sensing platforms.18 Among the diverse nanomaterials, platinum nanoparticles (PtNPs) have received extensive study due to their unique properties, which include high electrical conductivity and excellent electrocatalytic activity.19 The incorporation of PtNPs onto graphene nanosheets enhances electron transfer and amplifies signals.
Cyclodextrins (CDs) are toroidal-shaped structures with a hydrophilic exterior and hydrophobic inner cavity. These characteristics allow them to selectively bind various inorganic, organic and biological guest molecules into their cavities to form a stable host–guest inclusion complex with high molecular selectivity.20 CDs are water-soluble, environmentally friendly and can improve the stability and solubility of functional materials.21 When introducing CDs into PtNPs/graphene hybrids, the new material can be obtained with both the unique properties of graphene (large surface area, high conductivity and catalytic effects), PtNPs (excellent electrocatalytic activity) and CDs (host–guest supramolecular recognition and enrichment capability), which provides good opportunities for applications in electrocatalysis and sensors.22,23
Heptakis-(2,3,6-tri-O-methyl)-β-cyclodextrin (TM-β-CD), a derivative of β-CD, can form supramolecular structures with BPA more tightly than other CDs. We therefore chose TM-β-CD to synthesize high-quality graphene/PtNPs nanocomposites (TM-β-CD-Gr/PtNPs) by a facile one-pot approach. Scheme 2 shows the procedure for preparing the TM-β-CD-Gr/PtNPs and BPA sensor via an electrochemical strategy. The results indicated that the oxidation signal of BPA was remarkably improved after modifying the electrode with TM-β-CD-Gr/PtNPs. This shows that TM-β-CD-Gr/PtNPs hybrids can be used for the electrochemical detection of BPA with high sensitivity. The sensor also showed good repeatability and stability for the determination of BPA. Moreover, this sensor was successfully used for the determination of BPA in tap water samples with satisfactory results.
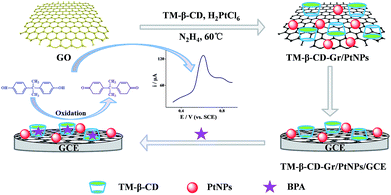 |
| Scheme 2 Schematic of the procedure for the synthesis of TM-β-CD-Gr/PtNPs and the detection of BPA using an electrochemical strategy. | |
Experimental
Materials
Graphite and chloroplatinic acid (H2PtCl6) were obtained from Alfa Aesar. BPA was obtained from Aladdin. TM-β-CD was purchased from Sigma-Aldrich. Ammonia solution (25–28 wt%) and hydrazine solution (50 wt%) were purchased from Beijing Chemical Reagent Factory (Beijing, China). A 1.0 × 10−2 M standard solution of BPA was prepared in alcohol. All other reagents were of analytical-reagent grade and were used without further purification. The water used throughout all experiments was purified using a Millipore system.
Apparatus
Ultraviolet-visible (UV-vis) absorption spectra were recorded on a U-3010 spectrometer (Hitachi, Tokyo, Japan). X-ray photoelectron spectroscopy (XPS) measurements were performed on an ESCALAB-MKII 250 photoelectron spectrometer (VG) with Al Kα radiation as the X-ray source for excitation. The morphology of the nanocomposites was recorded on a JELO-2100 transmission electron microscope with an accelerating voltage of 200 kV. Electrochemical measurements were made using a CHI660C electrochemical workstation (Chenhua Instruments, Shanghai, China). A three-electrode system was used with the glassy carbon electrode (GCE, 3 mm diameter) as the working electrode; a Pt wire electrode and a saturated calomel electrode were used as the counter and reference electrodes, respectively.
Synthesis of TM-β-CD-Gr/PtNPs, Gr/PtNPs and TM-β-CD-Gr
Graphene oxide (GO) was synthesized from natural graphite powder by Hummers' method with a slight modification.24 The TM-β-CD-Gr/PtNPs were prepared as follows: first, 1.5 mL of 1.0 mg mL−1 homogeneous GO dispersion were mixed with 3.0 mL of 4.0 mg mL−1 TM-β-CD aqueous solution. Then, 100 μL of ammonia solution, 100 μL of 19.3 mM H2PtCl6 and 20 μL of hydrazine solution were added to the flask. After vigorous stirring for a few minutes, the flask was put in a water bath (60 °C) for 3.5 h and a stable black dispersion was obtained. The dispersion was filtered through a nylon membrane (0.22 μm) and washed twice with water to remove free TM-β-CD. TM-β-CD-Gr/PtNPs were obtained. The method of preparation of the Gr/PtNPs with TM-β-CD-Gr was similar to that for the TM-β-CD-Gr/PtNPs hybrids, except TM-β-CD and H2PtCl6 were not added.
Preparation of TM-β-CD-Gr/PtNPs/GCE, Gr/PtNPs/GCE and TM-β-CD-Gr/GCE
The GCE was polished to a mirror-like surface with 0.05 μm alumina slurry and then sonicated with ethanol and redistilled water. The pretreated GCE was dried with high-purity nitrogen gas, and then 6.0 μL of 0.31 mg mL−1 TM-β-CD-Gr/PtNPs, Gr/PtNPs or TM-β-CD-Gr were carefully cast onto the cleaned GCE before drying at room temperature to obtain the modified electrodes.
Results and discussion
Characterization of the TM-β-CD-Gr/PtNPs
The TM-β-CD-Gr/PtNPs were characterized by UV-vis spectroscopy. Fig. 1A shows that the GO dispersion had a maximum absorption at 229 nm due to the π–π* transition of aromatic C
C bonds and a shoulder at 290–300 nm corresponding to the n–π* transition of the C
O bond.25 The absorption spectrum of H2PtCl6 had a maximum absorption at 259 nm due to the coordinated metal charge transfer transition of [PtCl6]2−. After the GO solution had been reduced by hydrazine, the absorption peak at 229 nm significantly red-shifted to 267 nm. This indicates that the electronic conjugation within graphene was restored by hydrazine.26 The obvious absorption peak of H2PtCl6 at 259 nm disappeared, confirming that H2PtCl6 was reduced to PtNPs.
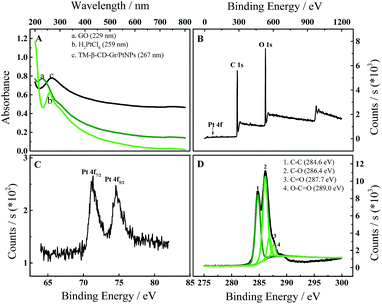 |
| Fig. 1 (A) UV-vis absorption spectra of (a) GO, (b) H2PtCl6 and (c) TM-β-CD-Gr/PtNPs solution. (B) Survey XPS spectrum of TM-β-CD-Gr/PtNPs. (C and D) XPS spectra of (C) Pt 4f and (D) C 1s for TM-β-CD-Gr/PtNPs. | |
The formation of the TM-β-CD-Gr/PtNPs was further characterized by XPS. Compared with the graphene nanosheets, the TM-β-CD-Gr/PtNPs (Fig. 1B) showed a clear Pt 4f peak originating from the PtNPs. The peaks at 71.1 and 74.5 eV correspond to Pt 4f7/2 and Pt 4f5/2, respectively (Fig. 1C). Fig. 1D shows the C 1s deconvolution spectrum of the TM-β-CD-Gr/PtNPs, revealing the presence of four types of carbon bond: C–C (284.6 eV), C–O (286.4 eV), C
O (287.7 eV) and O–C
O (289.0 eV). Compared with the XPS spectrum of reduced graphene reported previously,23 the peak associated with C–C (284.6 eV) was weaker than that of C–O (286.4 eV). This is because TM-β-CD has a number of C–O bonds. These results demonstrate that TM-β-CD-Gr/PtNPs nanocomposites were formed.
The morphology of TM-β-CD-Gr/PtNPs can be seen from transmission electron microscopy (TEM). Fig. 2A shows that most of the PtNPs were uniformly distributed on the graphene surface and the size of the PtNPs varied from 2.0 to 5.0 nm with an average diameter of 3.3 ± 0.3 nm (Fig. 2B).
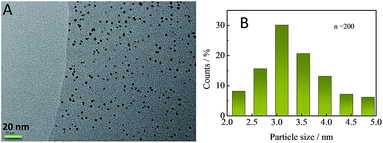 |
| Fig. 2 (A) TEM image of TM-β-CD-Gr/PtNPs. (B) Size distribution of PtNPs on TM-β-CD-Gr (n = 200). | |
Electrochemical behaviour of BPA on the TM-β-CD-Gr/PtNPs/GCE
It is well known that β-CD molecules can accommodate a variety of guest molecules within their cavities, showing a high sensitivity for detecting common environmental pollutants (including BPA) via the formation of supramolecular complexes. TM-β-CD, one of its derivatives, can form tighter supramolecules with BPA than other CDs and was chosen to chemically functionalize graphene to obtain TM-β-CD-Gr/PtNPs. It is worth noting that the introduction of a number of TM-β-CD molecules onto the surface of Gr/PtNPs can not only improve the dispersion and stability of the nanocomposite, but also enhance the electrochemical signal of BPA. To demonstrate this, the electrochemical behaviour of 10.0 μM BPA on the bare GCE, Gr/PtNPs/GCE, TM-β-CD-Gr/GCE and TM-β-CD-Gr/PtNPs/GCE was investigated by cyclic voltammetry in 0.1 M phosphate-buffered solution. Fig. 3, curve a shows that BPA exhibited very weak oxidation peaks at the bare GCE. However, the peak currents of BPA clearly increased at the Gr/PtNPs/GCE (Fig. 3, curve b) and TM-β-CD/Gr/GCE (Fig. 3, curve c). The signal enhancement of curve b may be attributed to the excellent electroconductivity of graphene and the catalytic activity of the PtNPs. The further increase in the peak current of curve c can be ascribed to the enrichment capability of TM-β-CD. At the TM-β-CD-Gr/PtNPs/GCE (curve d), the peak current exhibited an obvious increase compared with that obtained at the other two modified electrodes. This indicates that the Gr/PtNPs improved the catalytic activity and increased the effective electrode area towards BPA oxidation in addition to the enrichment capability of TM-β-CD by the formation of a supramolecular system with BPA. The effective surface areas of the bare GCE, TM-β-CD-Gr/GCE and TM-β-CD-Gr/PtNPs/GCE were determined by chronocoulometry and were 0.064, 0.137 and 0.171 cm2, respectively. These phenomena suggest that the TM-β-CD-Gr/PtNPs not only showed the excellent properties of Gr/PtNPs, but also exhibited the excellent supramolecular recognition capability of TM-β-CD. All these results demonstrated that the sensor had been successfully fabricated.
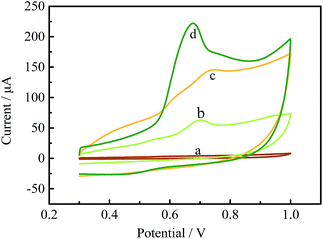 |
| Fig. 3 Cyclic voltammograms of 10.0 μM BPA at (a) the GCE, (b) the Gr/PtNPs/GCE, (c) the TM-β-CD-Gr/GCE and (d) the TM-β-CD-Gr/PtNPs/GCE in 0.1 M phosphate buffer. | |
Optimization of the determination conditions
Influence of pH.
Fig. 4A shows the influence of pH on the electrochemical response of the TM-β-CD-Gr/PtNPs/GCE towards the detection of BPA by cyclic voltammetry. The results show that the pH affected both the peak potential and the peak current of BPA. Fig. 4B shows that the peak potentials shifted negatively as the pH increased. The regression equation of the oxidation peak potential and pH is: Epa (V) = −0.067pH + 1.157 (r =0.9788). The slope of the regression equation is close to the theoretical value of 59 mV pH−1, indicating that the number of electrons and protons involved in the electrochemical oxidation of the BPA in the TM-β-CD-Gr/PtNPs/GCE system should be equal.27 The peak current of BPA increased with increasing pH from 5.0 to 7.0 and then decreased with further increases in pH. Therefore phosphate buffer (0.1 M) with a pH of 7.0 was used as the supporting electrolyte for BPA in the subsequent electrochemical experiments.
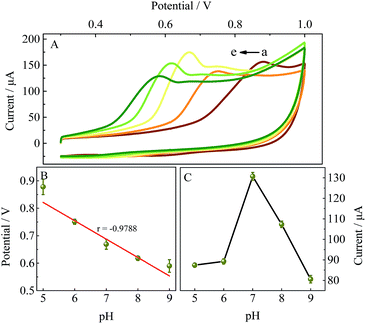 |
| Fig. 4 (A) Cyclic voltammograms of 10.0 μM BPA on the TM-β-CD-Gr/PtNPs/GCE at different pH values (from a to e): 5.0, 6.0, 7.0, 8.0 and 9.0. (B) Relationship between pH and peak potential. (C) Effect of pH on the peak current. The measurements were repeated three times to obtain the standard deviation. | |
Effect of the amount of TM-β-CD-Gr/PtNPs.
The amount of modifier plays an important part in the detection of BPA. Fig. 5A shows that the peak current increased remarkably when the amount of 0.31 mg mL−1 TM-β-CD-Gr/PtNPs suspension deposited on the GCE increased from 3.0 μL to 6.0 μL. However, when the volume of TM-β-CD-Gr/PtNPs exceeded 6.0 μL, the peak current decreased dramatically. This may be ascribed to the thicker film of TM-β-CD-Gr/PtNPs that blocked the transfer of electrons and slowed the mass transfer process. Therefore 6.0 μL of 0.31 mg mL−1 TM-β-CD-Gr/PtNPs suspension was chosen as the optimum volume.
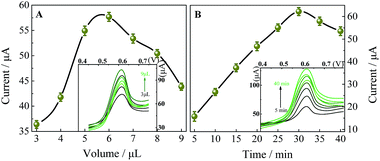 |
| Fig. 5 (A) Effect of the amount of TM-β-CD-Gr/PtNPs (0.31 mg mL−1) on the peak current. (B) Effect of the accumulation time on the peak current of 10.0 μM BPA in 0.1 M phosphate buffer (pH 7.0) at the TM-β-CD-Gr/PtNPs/GCE. The measurements were repeated three times to obtain the standard deviation. | |
Influence of the accumulation time.
Another key factor that influences the determination of BPA is the accumulation time. Fig. 5B shows that the peak current of 10 μM BPA on the TM-β-CD-Gr/PtNPs/GCE increased rapidly with the accumulation time in the range 5–30 min and then remained almost unchanged. This indicates that the adsorption/extraction of BPA on the electrode reached equilibrium. Therefore 30 min was chosen as the optimum accumulation time in the following experiments.
Effect of scan rate.
Fig. 6A shows the cyclic voltammograms of 10 μM BPA in phosphate buffer (pH 7.0) with scan rates varying from 40 to 200 mV s−1. The scan rate affects both the peak potential and the peak current. The oxidation peak potentials (Epa) shifted positively with increasing scan rate and the oxidation peak currents varied with the scan rate. The regression equation is: ipa (μA) = 0.7399ν (mV s−1) + 22.83 (r = 0.9936) (Fig. 6B). This result suggests that the electrochemical process of BPA at the TM-β-CD-Gr/PtNPs/GCE is surface-controlled.
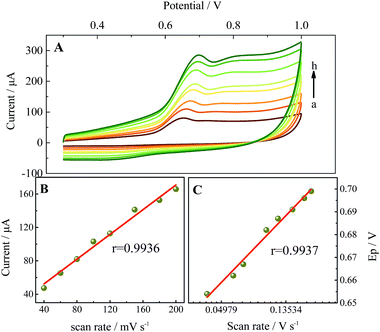 |
| Fig. 6 (A) Cyclic voltammograms of 10.0 μM BPA at the TM-β-CD-Gr/PtNPs/GCE in 0.1 M phosphate buffer (pH 7.0) at different scan rates (from a to h): 40, 60, 80, 100, 120, 150, 180 and 200 mV s−1. (B) Plot of the oxidation peak currents versus scan rate. (C) Relationship between the peak potential and the logarithm of the scan rate. | |
Fig. 6C shows the linear relationships between the oxidation peak potential and the natural logarithm of scan rate. The regression equation is: Epa (V) = 0.02932
ln
ν (V s−1) + 0.7469 (r = 0.9937). In the case of a surface-controlled and totally irreversible electrode process, according to Laviron,28Ep may be expressed as follows:
where
E0 is the formal potential,
k0 is the electrochemical rate constant,
R is the gas constant,
T is the temperature,
α is the transfer coefficient,
n is the number of electrons transferred in the rate-determining step,
ν is the scan rate and
F is the Faraday constant. From the slope of the line, we can calculate that the value of
αn was 0.8757. When
α was assumed to be 0.5, the number of electrons
n was about 2.
29 A possible oxidation reaction mechanism is shown in
Scheme 3.
 |
| Scheme 3 Oxidation reaction mechanism of BPA at the TM-β-CD-Gr/PtNPs/GCE. | |
Electrochemical sensing of BPA on the TM-β-CD-Gr/PtNPs/GCE
Differential pulse voltammetry (DPV) has a higher sensitivity than cyclic voltammetry and is more suitable for quantitative analysis. Therefore the electrochemical sensing performance of TM-β-CD-Gr/PtNPs/GCE towards BPA was investigated by DPV under the optimum conditions. Fig. 7 shows that the peak currents of BPA increased linearly with concentration in the range 50 nM to 80 μM. The linear regression equation is: ipa (μA) = 25.583
log
C (M) + 189.637 (r = 0.9986), where C is the concentration of BPA and ipa is the oxidative peak current. The detection limit for BPA was estimated to be 15 nM (S/N = 3), which is comparable, or more sensitive than, the previous electrochemical sensors for the determination of BPA. The reported BPA analytical parameters are summarized in Table 1 and indicate that the TM-β-CD-Gr/PtNPs/GCE had a broad linear range and a low detection limit.
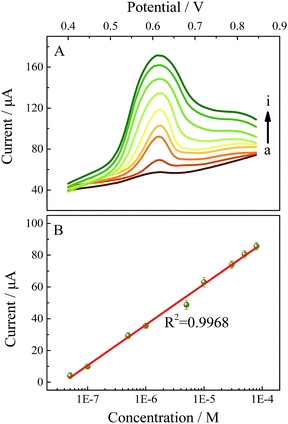 |
| Fig. 7 (A) DPV response at the TM-β-CD-Gr/PtNPs/GCE in 0.1 M phosphate buffer (pH 7.0) for different concentrations of BPA (from a to i): 0.050, 0.10, 0.50, 1.0, 5.0, 10, 30, 50 and 80 μM. (B) Calibration graph between the oxidation peak current and the BPA concentration (logarithm). The measurements were repeated three times to obtain the standard deviation. | |
Table 1 Analytical parameters for BPA detection at different modified electrodes
Modified electrode |
Linear range (μM) |
Detection limit (nM) |
Reference |
CMK-3/nano-CILPE |
0.2–150 |
50 |
30
|
GR-IL/GCE |
0.02–2 |
80 |
12
|
ECL(Ru(byp)32+/N–C dots) |
0.03–1 |
10 |
31
|
Pt/Gr–CNTs/GCE |
0.06–10.0 |
42 |
14
|
ZnTsPc/f-GN/GCE |
0.05–4 |
20 |
13
|
NCNF/CPE |
0.1–60 |
50 |
32
|
NPG/GCE |
0.1–50 |
12.1 |
33
|
MWCNT–PTh–Pt/GCE |
0.05–0.4 |
3 |
34
|
TM-β-CD-Gr/PtNPs/GCE |
0.050–80 |
15 |
This work |
Repeatability and stability of the TM-β-CD-Gr/PtNPs/GCE
The repeatability of the TM-β-CD-Gr/PtNPs/GCE was investigated by detecting the peak current of 10 μM BPA in ten parallel determinations. The relative standard deviation was 4.4%. To investigate the stability of the sensor, the modified electrode was stored in a refrigerator at 4 °C for 3 weeks; the signal decreased by 3.7% compared with the original response. These results illustrate that the sensor showed good repeatability and stability for the detection of BPA.
Interference study
An interference study was conducted by the determination of 10 μM BPA in the presence of five-fold concentrations of organic molecules and 100-fold concentrations of metal and inorganic ions under the optimum conditions. Fig. 8 shows that five-fold concentrations of uric acid, ascorbic acid, citric acid, glucose, o-nitrophenol, m-nitrophenol and p-nitrophenol had no obvious interference, with deviations <10%. Some metal and inorganic ions, such as 100-fold concentrations of Ca2+, Mg2+, K+, Na+, Ni2+, Fe3+, Al3+, NO3−, SO42− and Cl−, had no influence on the detection of BPA. These results demonstrate that the proposed sensor is relatively selective for the determination of BPA.
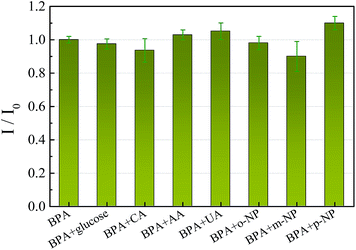 |
| Fig. 8 Interference study of the proposed method for BPA detection (I0, peak current of BPA; I, peak current of the interferent). Error bars show the standard deviations of measurements from three experiments. CA, citric acid; AA, ascorbic acid; UA, uric acid; and NP, nitrophenol. | |
Practical sample analysis
The practical application of the electrochemical sensor was investigated by the standard addition method. A series of samples obtained by spiking different concentrations of BPA into tap water was analysed. The results are summarized in Table 2, which shows that the recoveries were in the range 98.2–102.5%. This demonstrates that the proposed electrochemical sensor has promising potential applications in the analysis of real samples.
Table 2 Detection of BPA in tap water
Sample |
BPA added (μM) |
BPA found (μM) |
Recovery (%) |
RSD (%) (N = 5) |
1 |
0.80 |
0.82 |
102.5 |
4.02 |
2 |
1.0 |
1.01 |
101.0 |
3.18 |
3 |
3.0 |
2.98 |
99.3 |
4.20 |
4 |
5.0 |
4.91 |
98.2 |
2.40 |
Conclusion
TM-β-CD-Gr/PtNPs were used as novel electrode materials for the sensitive detection of BPA by an electrochemical method. The TM-β-CD attached to the Gr/PtNPs surface plays an important part in forming well-dispersed graphene and shows high supramolecular recognition properties with the enrichment of guest molecules. By using the excellent properties of TM-β-CD-Gr/PtNPs, a simple electrochemical sensing platform for the detection of BPA has been successfully constructed. This method has a high sensitivity, good stability and a broad linear range. This sensor is also convenient and cost-effective and offers great opportunities for the detection of phenol compounds in environmental pollutants.
Acknowledgements
This research work was supported by the National Natural Science Foundation of China (No. 21275093) and the Young “Sanjin” Scholar Foundation of Shanxi Province, 2016.
References
- H. Sambe, K. Hoshina, K. Hosoya and J. Haginaka, J. Chromatogr. A, 2006, 1134, 16–23 CrossRef CAS PubMed.
- N. Huang, M. Liu, H. Li, Y. Zhang and S. Yao, Anal. Chim. Acta, 2015, 853, 249–257 CrossRef CAS PubMed.
- K. K. Reza, M. A. Ali, S. Srivastava, V. V. Agrawal and A. M. Biradar, Biosens. Bioelectron., 2015, 74, 644–651 CrossRef CAS PubMed.
- F. S. vom Saal, P. S. Cooke, D. L. Buchanan, P. Palanza, K. A. Thayer, S. C. Nagel, S. Parmigiani and W. V. Welshons, Genes, Chromosomes Cancer, 2015, 55, 143–147 Search PubMed.
- A. Ballesteros-Gómez, S. Rubio and D. Pérez-Bendito, J. Chromatogr. A, 2009, 1216, 449–469 CrossRef PubMed.
- J. Sajiki, K. Takahashi and J. Yonekubo, J. Chromatogr. B: Biomed. Sci. Appl., 1999, 736, 255–261 CrossRef CAS.
- H. Sambe, K. Hoshina, K. Hosoya and J. Haginaka, Analyst, 2005, 130, 38–40 RSC.
- S. Alsudir, Z. Iqbal and E. P. C. Lai, Electrophoresis, 2012, 33, 1255–1262 CrossRef CAS PubMed.
- K. V. Ragavan, L. S. Selvakumar and M. S. Thakur, Chem. Commun., 2013, 49, 5960–5962 RSC.
- X. Chen, C. Wang, X. Tan and J. Wang, Anal. Chim. Acta, 2011, 689, 92–96 CrossRef CAS PubMed.
- E. Chung, J. Jeon, J. Yu, C. Lee and J. Choo, Biosens. Bioelectron., 2015, 64, 560–565 CrossRef CAS PubMed.
- P. Jing, X. M. Zhang, Z. X. Wu, L. Bao, Y. L. Xu, C. Z. Liang and W. Q. Cao, Talanta, 2015, 141, 41–46 CrossRef CAS PubMed.
- K. Y. Hou, L. Huang, Y. B. Qi, C. X. Huang, H. B. Pan and M. Du, Mater. Sci. Eng., C, 2015, 49, 640–647 CrossRef CAS PubMed.
- Z. X. Zheng, Y. L. Du, Z. H. Wang, Q. L. Feng and C. M. Wang, Analyst, 2013, 138, 693–701 RSC.
- X. Zhang, L. Wu, J. W. Zhou, X. H. Zhang and J. H. Chen, J. Electroanal. Chem., 2015, 742, 97–103 CrossRef CAS.
- K. S. Novoselov, A. K. Geim, S. V. Morozov, D. Jiang, Y. Zhang, S. V. Dubonos, I. V. Grigorieva and A. A. Firsov, Science, 2004, 306, 666–669 CrossRef CAS PubMed.
- D. Chen, L. Tang and J. Li, Chem. Soc. Rev., 2010, 39, 3157–3180 RSC.
- S. Niyogi, E. Bekyarova, M. E. Itkis, J. L. McWilliams, M. A. Hamon and R. C. Haddon, J. Am. Chem. Soc., 2006, 128, 7720–7721 CrossRef CAS PubMed.
- S. Guo, D. Wen, Y. Zhai, S. Dong and E. Wang, ACS Nano, 2010, 4, 3959–3968 CrossRef CAS PubMed.
- M. V. Rekharsky and Y. Inoue, Chem. Rev., 1998, 98, 1875–1918 CrossRef CAS PubMed.
- R. Challa, A. Ahuja, J. Ali and R. K. Khar, AAPS PharmSciTech, 2005, 6, E329–E357 CrossRef PubMed.
- G. Zhu, P. Gai, L. Wu, J. Zhang, X. Zhang and J. Chen, Chem.–Asian J., 2012, 7, 732–737 CrossRef CAS PubMed.
- Y. J. Guo, S. J. Guo, J. T. Ren, Y. M. Zhai, S. J. Dong and E. K. Wang, ACS Nano, 2010, 4, 4001–4010 CrossRef CAS PubMed.
- W. S. Hummers and R. E. Offeman, J. Am. Chem. Soc., 1958, 80, 1339 CrossRef CAS.
- P. K. Ang, S. Wang, Q. L. Bao, J. T. L. Thong and K. P. Loh, ACS Nano, 2009, 3, 3587–3594 CrossRef CAS PubMed.
- D. Li, M. B. Muller, S. Gilje, R. B. Kaner and G. G. Wallace, Nat. Nanotechnol., 2008, 3, 101–105 CrossRef CAS PubMed.
- P. H. Deng, Z. F. Xu and Y. F. Kuang, J. Electroanal. Chem., 2013, 707, 7–14 CrossRef CAS.
- E. Laviron, J. Electroanal. Chem. Interfacial Electrochem., 1974, 52, 355–393 CrossRef CAS.
- H. F. Zhang, L. F. Xu and J. B. Zheng, Talanta, 2007, 71, 19–24 CrossRef CAS PubMed.
- Y. H. Li, X. R. Zhai, X. S. Liu, L. Wang, H. R. Liu and H. B. Wang, Talanta, 2016, 148, 362–369 CrossRef CAS PubMed.
- L. B. Li, B. Yu, X. P. Zhang and T. Y. You, Anal. Chim. Acta, 2015, 895, 104–111 CrossRef CAS PubMed.
- J. Y. Sun, Y. Liu, S. M. Lv, Z. R. Huang, L. Cui and T. Wu, Electroanalysis, 2016, 28, 439–444 CrossRef CAS.
- X. P. Yan, C. Q. Zhou, Y. Yan and Y. Zhu, Electroanalysis, 2015, 27, 2718–2724 CrossRef CAS.
- J. Wan, Y. X. Si, C. Li and K. Zhang, Anal. Methods, 2016, 8, 3333–3338 RSC.
|
This journal is © The Royal Society of Chemistry 2017 |
Click here to see how this site uses Cookies. View our privacy policy here.