DOI:
10.1039/C6AY02656G
(Paper)
Anal. Methods, 2017,
9, 124-133
A novel ditopic chemosensor for cadmium and fluoride and its possible application as a pH sensor†
Received
24th September 2016
, Accepted 8th November 2016
First published on 1st December 2016
Abstract
A urea-based BPC [1,5-bis(perfluorophenyl)carbonohydrazide] molecule with four acidic NH protons has been synthesized by a facile synthetic process. The molecule was found to be a ditopic chemosensor for Cd2+ and F− ions. BPC was synthesized from low-cost starting materials, dinitrophenyl hydrazine and triphosgene. The host–guest interactions between the ions (Cd2+ and F−) were not only confirmed by convenient spectroscopic techniques such as UV-Vis, PL, 1H-NMR, FT-IR, and cyclic voltammetry but also through modern DFT, the results of which were in good agreement with the experimental results. In vitro studies in human cancer cells (HeLa cells) were successfully performed with BPC and Cd2+ using fluorescence microscopy. The reversible UV-Vis response for BPC with F−, OH− and H+ mimics multiple logic functions and can be used for several complex electronic circuits based on logic operations. The pH sensor (BPC) can be further interfaced with suitable circuitry interfaced with appropriate programming for ease of access and enhancement of its practical applications.
Introduction
Recently, the design and development of chromogenic and fluorogenic chemosensors for cations and anions has gained immense significance because of their enormous environmental and medicinal applications. It is challenging yet necessary to develop chemosensors which can detect toxic ions by induced signal changes (change in wavelength after recognition or changes in intensity or emission wavelength). Among various toxic and carcinogenic1 cations, Cd2+ is one of the most toxic metal ions because of its adverse effects on human health, even at low levels. Its exposure through food, dust, etc. leads to several heart diseases as well as liver and kidney damage. The safe limit of Cd2+ in drinking water is from 3 to 5 ppb.2 Therefore, small organic molecules as chemosensors which can detect Cd2+ ion optically by the naked eye are currently in great demand. It is noteworthy that the existing methods of Cd2+ quantification are costly and tedious.3 To date, there have been few reports of selective fluorimetric/colorimetric chemosensors for Cd2+.4 One reason for this is strong interference from Zn2+; this occurs because these cations share similar chemical properties, as they are in the same group. To date, urea-based systems have been largely exploited with the aim of sensing selective anions by the presence of amide hydrogens. Urea-based chemosensors for sensing cations are rare in the literature.5 In consideration of this, herein, emphasis has been given to the design and synthesis of a rare urea-based chemosensor that can sense cations and anions both selectively and simultaneously (a ditopic chemosensor).6 The chemosensor BPC [1,5-bis(perfluorophenyl)carbonohydrazide] in the present work is not only a selective sensor for a toxic cation, Cd2+, but also selectively senses a specific anion, F− ion, in organic–aqueous medium.
It is a well-known fact that variation in pH plays a crucial role in physiological processes. In the human body, normal pH varies between 6.8 and 7.4.7 Therefore, variations in body pH (lower or higher compared to pH 7) are often responsible for abnormal functioning of human cells. In general, cancer cells are linked with changes or variations in pH in comparison to normal cells.8 Recently, pH sensors for monitoring pH inside living cells and evaluating several intracellular activities have become important. Considering the importance of pH sensing, and due to the presence of four NH hydrogens, the newly developed sensor molecule represents a step forward in sensing pH in the range from pH 6 to 11.
To understand host–guest interactions and to support experimental outcomes, density functional theory (DFT) studies are essential.9
As the basis of current computers, electronic logic gates perform binary arithmetic and several logical operations. Logic gates are mainly switches with output and input modes, where the output mode (0 or 1) depends on the existing or applied input mode (0 or 1).10,11 Molecular logic gates actually function based on input signals arising from chemical processes and show output signals in line with spectroscopic outcomes. The reversible recognition events of BPC in the presence of OH− and H+ have been interpreted in a logic gate9a,c operation. Currently, the interfacing of chemistry with electronics is of significant interest; this interfacing is achieved by connecting microcontroller services with chemosensors, such as BPC in the present work, with proper circuitry and suitable programming for ease of access and enhancement of the practical applications of the chemosensors.
Experimental
Materials
All starting materials (chemical reagents and solvents) were commercially available and were of analytical grade. Solvents such as dichloromethane, methanol, and ether were distilled and dried prior to use. All tetrabutyl ammonium salts of anions, such as fluoride (hydrate), chloride, nitrate, bromide, hydrogen sulfate, acetate and phosphate, were purchased from Sigma Aldrich chemical company and were used as received. DMSO and DMSO-d6 were of spectroscopic grade, were purchased from Merck India Pvt. Ltd, and were used without further purification.
Apparatus
The infrared spectra were recorded on a Perkin Elmer FT-IR Spectrum 100 spectrophotometer. The mass spectra were obtained using an Advion Expression compact mass spectrometer (CMS, serial number: 3013-0140). 1H-NMR spectra were recorded using a Bruker AV-400 spectrometer. UV-Vis spectra were acquired using a CARY60 spectrophotometer. Fluorescence experiments were performed using a Perkin Elmer LS-45 spectrometer. Cyclic voltammetry was performed with a CH Instruments apparatus, and X-ray crystallography was performed using a Bruker Smart APEX-II [Mo Tube X-Ray Source 0; 3-Axis goniometer; 512 px × 512 px CCD camera (detector)]. Cell imaging was performed using a ZEISS Observer Z1 microscope.
Computational details
Calculations were performed using Turbomole (V7.0).12 The geometry optimizations were carried out at the DFT(BP86)/def-SV(P) level. B-P86 functional exchange: LDA + Becke (B88) correlation; LDA (VWN) + Perdew (P86) iterations performed with small grid spherical integration; Lebedev's spherical grid spherical grid size: 5, i.e. gridpoints: value for diffuse not defined radial integration, 590: Chebyshev 2nd kind (scaling 3) radial gridsize: 8; integration cells: 35; partition function: Becke; partition sharpness: 3 basis sets def-SV(P) were used for fluorine, nitrogen, oxygen, carbon and hydrogen-like atoms. 1e-integrals were neglected if expon. factor < 0.734497 × 10−11. The difference densities algorithm was switched on. The maximal number of linear combinations of difference densities was 20. Diagonalization of the Fock matrix in the last SCF iteration was activated. Automatic virtual orbital shift was switched on if e(lumo)–e(homo) < 0.10000000. DIIS switched on: error vector was FDS-SDF. Max. iterations for DIIS: 4 DIIS matrix (see manual). Scaling factor of diagonals: 1.200; threshold for scaling factor: 0.000; scf convergence criterion: increment of total energy < 0.1000000 × 10−5 and increment of one-electron energy < 0.1000000 × 10−2.
X-ray crystallography
A Bruker Smart APEX-II instrument [Mo Tube X-Ray Source 0; 3-Axis goniometer; 512 px × 512 px CCD camera (detector)] equipped with a Mo-target rotating-anode X-ray source and a graphite monochromator (Mo K-alpha, λ = 0.71073 Å) was used throughout. The ambient temperature was 296 K. Final cell constants were obtained from least-squares fits of all measured reflections. The SADABS program was used for Bruker area detector absorption and other corrections. The Bruker ShelXTL13 software package was used for solution, refinement and artwork of the structures. All non-hydrogen atoms were anisotropically refined, and hydrogen atoms were placed at calculated positions and refined as riding atoms with isotropic displacement parameters.
Synthesis
(BPC) 1,5-bis(perfluorophenyl)carbonohydrazide (Scheme 1).
To a solution of pentafluorophenyl hydrazine (0.198 g, 1 mM) in dichloromethane, solid triphosgene (0.296 g, 1 mM) was added under N2 atmosphere. Immediately, a saturated aqueous solution of NaHCO3 (7 ml) was added dropwise. Effervescence of carbon dioxide was observed and gradually diminished. Stirring was continued for 12 h under nitrogen atmosphere. The crude material thus obtained was collected, placed in a separating funnel, and washed with water several times to remove excess NaHCO3 (Scheme S1†). The colourless dichloromethane solution was collected after washing with water, and excess water was removed with anhydrous Na2SO4. White crystals of BPC were obtained by slow evaporation of the acetonitrile solution.
Results and discussion
The white crystalline BPC was fully characterized by solid state elemental analyses, FT-IR (Fig. S1†) and single crystal X-ray analysis (Fig. S4 and Tables S1–S4†), and by solution state analyses such as UV-Vis, 1H-NMR and ESI-MS (Fig. S6, S3 and S2†). CHN anal. calcd for C13N4OF10H4: C: 36.96%; H: 0.9%; N: 13.27%; found. C: 36.8%; H: 0.8%; N: 12.8%. Yield: 85 g, 20.1%; (m/z) calcd 422 for C13N4OF10H4: found 421.39: 1H-NMR (300 MHz, DMSO-d,6 Me4Si) δ = 8.6 (s, 2H), 7.69 (m, 2H).
Crystal discussion
The crystal structure of BPC molecule shows a bridge [(–NH–NH)2–C
O] linking two almost planar phenyl units (Fig. 1). The ORTEP (Oak Ridge Thermal-Ellipsoid Plot Program) and atom numbering scheme are shown in Fig. S4.† The strength of ORTEP lies in its capacity to generate stereoscopic images automatically. The probability was found to be 30%. Elongated ellipsoids generally indicate distortion. ORTEP provides various pieces of information about a crystal structure, such as its R factor, space group, and crystal system. The single crystal of BPC molecule crystallized in the space group P21/c (R 0.06). The crystals were obtained after dissolving the compound in acetonitrile solvent followed by slow evaporation. The C
O distance in BPC was found to be 1.236 Å. The adjacent C–N distances are 1.362 Å and 1.341 Å, respectively (compared with the urea unit); the variation in bond length of the two adjacent C–N distances is due to H-bonding, which is extensively present on one C–N side; meanwhile, on the other C–N side, its presence is less prominent (video ESI file, Fig. S5†). The N–N distances of the hydrazine unit are 1.406 and 1.401 Å, which are slightly shorter than the bond distances in unsubstituted hydrazine. The N–C(
O)–N angle is 115.7°, while the N–N–C angles at the adjacent sides are 121.86° (N006–N008–C00G) and 120.99° (C00G–N1–N00A), respectively.
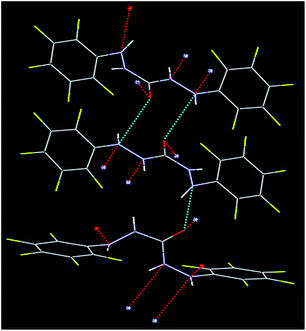 |
| Fig. 1 The crystal structure of BPC molecule. | |
Optical responses of BPC and Cd2+
The absorption spectra of the BPC molecule in acetonitrile–water medium shows two responses, one at 224 nm and the other at 270 nm (Fig. S5†), which correspond to the π–π* and n–π* bands14 of the molecule. There is no charge transfer band in the molecule. With gradual addition of Cd2+, the 270 nm band increases in absorbance intensity; also, a new peak at 490 nm emerges and steadily increases in intensity, although less significantly than the 270 nm peak (Fig. 2a). This response proves that coordination occurs between BPC and Cd2+. 0 to 100 equiv. Cd2+ was added. The peak at 490 nm increased further in intensity from approximately 0.5 to 1 with time, indicating that slow kinetics prevailed in the system. The colorimetric responses of BPC to different cations are shown in Fig. 3; the responses indicate the high selectivity of BPC for sensing Cd2+ over other cations. The m/z value of BPC–Cd(NO3)2 adduct was found to be 597 (Fig. 2b), as predicted.
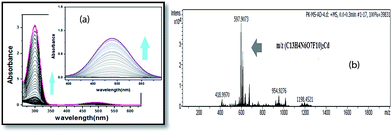 |
| Fig. 2 (a) Titration of BPC with Cd(NO3)2 in ACN–H2O (7 : 3 v/v) medium; (b) ESI-mass spectrum of BPC with Cd(NO3)2 adduct, showing the m/z value of 597. | |
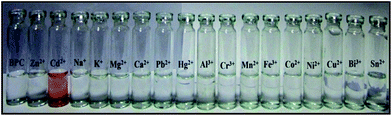 |
| Fig. 3 Colorimetric responses of BPC with various cations in ACN–H2O (7 : 3 v/v) medium. | |
BPC and F−.
With BPC and F−, further interesting results occurred. The peak at 224 nm (Fig. S6†) diminishes in intensity, while the peak at 270 nm gradually increases in intensity (Fig. 4). Simultaneously, a new peak at 490 nm emerges and reaches a very strong absorbance intensity upon addition of about 2.0 equivalents of F−. The increase in charge transfer in the system due to the greater electron density results in the emergence of a peak at 490 nm. The colorimetric response of BPC with different anions is shown in Fig. S7.†
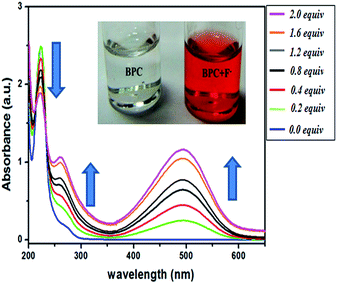 |
| Fig. 4 Titration of BPC with F− from 0–2 equivalent in ACN–H2O (9 : 1v/v) medium; inset: color of BPC in the presence (right) and absence (left) of F−. | |
To verify the reversibility of the response of BPC to F− followed by Cd2+ and EDTA, additional UV-Vis experimentation was performed (Fig. S8†). First, F− was added in excess to BPC solution; as expected, the peak at 490 nm increased in intensity. On adding Cd2+ in excess, the peak diminished from 1.5 units to 0.1 units due to the complexation of F− with Cd2+ and the formation of CdF2 complex with persistence of BPC. The Cd2+ peak at 490 nm had an absorbance value of 0.1. Next, with addition of EDTA, which is a stronger ligand than F−, a Cd—EDTA complex formed and F− ions were liberated. As a result, the 490 nm peak increased in intensity, showing greater charge transfer in BPC with F− ions along with an increase of the absorbance value to >1 unit.
Excitation and emission spectra
BPC and Cd2+.
To study the emission response of the chemosensor BPC to Cd2+, a fluorescence titration experiment was carried out in acetonitrile–H2O medium (Fig. S9†); it can be seen that the λem of BPC appears at 330 nm, with a very strong emission intensity of more than 700 nm. On very slow addition of Cd2+, the intensity decreased drastically from 700 nm to a final intensity of less than 100 nm. The peak simultaneously shifted to 355 nm. This is very different from general cases where fluorescence enhancement occurs with the addition of cations. This can be explained with the following approach. As we can see from the crystal structure of BPC molecule, the two pentafluorophenyl rings are quite distant from each other and face outward. However, when Cd2+ is added, it forms a bridge that enables electron transfer to occur in the system. Cd2+ is an electron-rich (d10) system; therefore, electrons can flow from the pi orbitals of one phenyl ring through the pi orbitals of Cd2+ to the other ring.17 As a result, PET is facilitated in the electron-rich system, and quenching of fluorescence is the obvious outcome.
BPC and F−.
In the presence of F− (Fig. S10†), fluorescence quenching occurs in in the BPC molecule. The study was performed in ACN–H2O medium; BPC initially showed very strong emission intensity of above 700 units, as stated previously. On gradual addition of F−, quenching of the intensity began and finally reached around 100 units. There was an almost negligible shift in the λmax position in the emission profile. This strong quenching of photoluminescence may be attributed to the occurrence of photoinduced electron transfer (PET) in the molecule following the increase in electron density in the system due to the addition of F− ion.
The binding constants of the BPC⋯Cd2+ adduct and the BPC⋯F− adduct were found to be 0.1 × 101 M−1 and 0.1 × 103 M−1, respectively, as calculated from the B–H plot (Fig. S19 and S20†). The limits of detection for Cd2+ and F− with BPC were found to be 2.8 × 10−5 M and 5.75 × 10−6 M, respectively, calculated from the plot of intensity vs. the concentration plot (Fig. S21 and S22†).12
pH studies
The chemosensor shows pH changes in the neutral–basic pH range from 6 to 11 (Fig. 5). The UV titration of BPC with OH− in ACN–H2O medium shows similar results to that of F− with BPC; however, saturation is obtained faster because of rapid deprotonation by the OH− ions. In MeOH–H2O medium (Fig. S11†), addition of NaOH to BPC shows similar changes; however, saturation is obtained faster than in ACN–H2O medium. The effect of acid on BPC is also shown in Fig. S12.† Interestingly, and in sharp contrast to F−, the fluorescence intensity of BPC with OH− decreases from 600 to 400 units; it then increases in intensity and attains a saturation of 1000 units with a bathochromic shift from the initial 330 nm to 350 nm (Fig. S13†). In the case of F−, the fluorescence intensity continued to diminish with gradual addition of the anion in organic medium. However, when OH− in the form of aqueous NaOH was added, the outcome was not analogous to that of F−. This anomaly can be attributed to the initial addition of OH−, which induced the fluorescence intensity of BPC to decrease similarly to F−, but more rapidly. With the passage of time, as an increasing amount of OH− was introduced in the system, the quantity of H2O in the system was also enhanced. This increased aqueous level of the system may be responsible for the enhanced response of BPC, in which the trend adopts an initial decrease followed by an increase in the emission profile. The ratio of emission intensity as a function of pH is shown in Fig. S14.† The acid–base reversibility, which persisted up to the 4th cycle, is shown in Fig. S15.† The ESI-mass spectrum of BPC with NaOH shows adduct formation at 463 (Fig. S16†). The pH sensitivity of BPC covering a wide range (pH 6 to 11), including the physiological pH range, endows it with potential applications as a pH sensor inside living human cells, although the pKas of pH sensors for in vitro applications lie in the range of 4 to 8.18 However, there are reports in the literature of sensors with pKa values less than 4 and greater than 8 for detecting intracellular pH.15
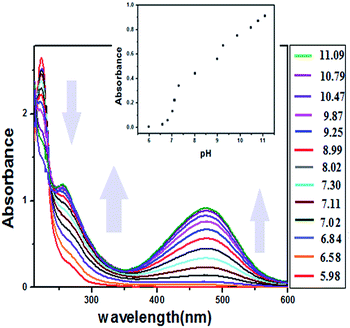 |
| Fig. 5 Titration of BPC with OH− from pH 6–11 in ACN–H2O medium (1 : 1 v/v); inset: plot of absorbance of BPCvs. pH. | |
Electrochemical studies
The electrochemical responses of BPC with TBAF/Cd(NO3)2/NaOH were examined by cyclic voltammetry studies in acetonitrile solvent with TBABF4 as the supporting electrolyte.18 The molecule BPC shows quasi-reversible cyclic voltammetry. The cathodic potential is at 0.16 V with a current height of 0.0138 mA, and the anodic potential is at 0.455 V with a current height of −0.033 mA. On addition of small amounts of TBAF (10−3 M, 5 μl), initially, there was little change in the peak positions (Fig. 6a). However, with the addition of about 100 μl of 10−3 M TBAF, some noticeable changes occurred in the initial responses of BPC. The reversibility of BPC disappeared with addition of TBAF. The cathodic potential flattened and shifted to −0.52 V, while the anodic voltage increased in current height from −0.033 mA to −0.0616 mA with no noticeable change in potential. Interestingly, a new potential appeared in the anodic region at 1.133 V. The increase in electron density in the system disrupts reduction and facilitates oxidation with increasing anodic current flow; thus, an additional peak is generated at 1.133 V. This result is in agreement with the fluorescence titration experimentation of BPC with F−, where an increase in the electron density of the system leads to fluorescence quenching.
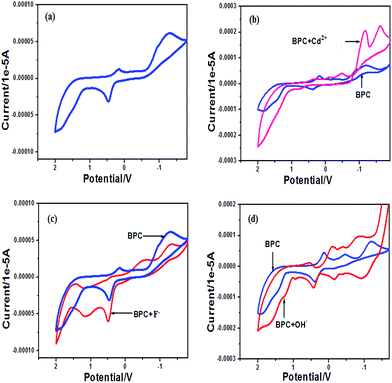 |
| Fig. 6 Electrochemical response of (a) BPC (10−3 M) in acetonitrile (b) with Cd2+, (c) with F− and (d) with OH− (each 10−2 M); supporting electrolyte, TBABF4; scan rate, 0.1 V s−1. | |
With BPC and OH− (Fig. 6b), very similar results are obtained; however, the current flow is greater due to an increase in the negative charge in the system on account of the rapid deprotonation of BPC by OH−.
Table 1 summarizes the electrochemical responses of BPC with F−/OH−/Cd2+ ions.
Table 1 Summary of the electrochemical responses of BPC to F−/OH−/Cd2+
|
BPC
|
After interaction with anions |
Initial |
F− |
Reduction |
E
c = 0.1633V |
E
c = −0.52 V |
I
c = 0.0138 mA |
I
c = 0.023 mA |
Oxidation |
E
a = 0.456 V |
E
a = 0.49 V |
I
a = −0.033 mA |
I
a = −0.0616 mA |
|
E
a = 1.133 V |
I
a = −0.0545 mA |
|
Initial |
OH− |
Reduction |
E
c = 0.142 V |
E
c = −0.109 V |
I
c = 0.046 mA |
I
c = 0.063 mA |
Oxidation |
E
a = 0.42 V |
E
a = 0.425 V |
I
a = 0.0723 mA |
I
a = −0.072 mA |
|
Initial |
Cd2+ |
Reduction |
E
c = 0.173 V |
E
c = −0.113 V |
I
c = 0.032 mA |
I
c = 0.0274 mA |
|
E
c = −0.484 V |
I
c = 0.041 mA |
|
E
c = −1.176 V |
I
c = 0.207 mA |
Oxidation |
E
a = 0.375 V |
E
a = 0.732 V |
I
a = −0.025 mA |
I
a = −0.0238 mA |
IR studies
The infrared spectrum16 of BPC (Fig. 7a) can be divided into three zones: (i) in the 3500 cm−1 to 3000 cm−1 range, several peaks are present. A small triplet at 2967 cm−1, 3014 cm−1 and at 3069 cm−1 may be due to aromatic sp2 and aliphatic sp2 C–H stretches. At an even higher range, there are three sharp, adjacent peaks at 3234, 3328 and 3411 cm−1 which gradually decrease in intensity and are definitely due to the N–H stretching of amides and secondary amines; (ii) in the 2800 cm−1 to 2000 cm−1 range, there are two clear peaks at 2685 cm−1 and at 2450 cm−1; and (iii) a third region from 2000 cm−1 to 500 cm−1 shows numerous sharp peaks at 1775, 1680 (amide, –C
O), and 1520, 1320, 1270, 1130, 970, and 890 cm−1, which correspond to the peaks of the urea moiety.
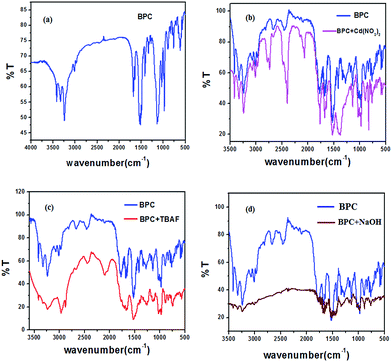 |
| Fig. 7 IR spectra of (a) BPC, (b) BPC with Cd2+, (c) BPC with NaOH and (d) BPC with Cd(NO3)2. | |
With the addition of TBAF salt (Fig. 7c), the peaks from 3500 cm−1 to 3000 cm−1 become flattened and broadened, showing the interaction between the NH hydrogens and F−. The peaks from 2000 cm−1 to 500 cm−1 also become flattened, suggesting greater electronic distribution in the system with the addition of F− ion.
In the case of Cd(NO3)2 (Fig. 7b), the peaks at 2685 cm−1 and 2450 cm−1 become sharper, and a new peak emerges at 2050 cm−1. In the entire spectrum of BPC, all the peaks become sharper on addition of Cd(NO3)2.
With NaOH (Fig. 7d), the peaks in the 3500 cm−1 to 3000 cm−1 range are greatly flattened, indicating strong interactions between the NH protons and OH−. In the 2800 cm−1 to 2000 cm−1 range, the peaks disappear. The region from 2000 cm−1 to 500 cm−1 shifts and becomes flattened.
NMR
A 1H-NMR titration experiment19 of BPC with F− at room temperature was performed in DMSO-d6 to obtain insight into the mode of interaction of BPC with F− (Fig. 8). There are only two distinct protons (a and b, Scheme 1) in the BPC molecule; their signals appear at 7.86 ppm and 8.6 ppm, respectively. With gradual addition of F−, the intensities of both the peaks at 7.86 ppm and 8.6 ppm diminish gradually, and they finally disappear at 2.0 equivalents of F− addition. No shifting occurs in the peak positions, which suggests that the interaction is dominated by H-bonding in the system. When we compare these results with the 1
:
2 binding mode in the modern DFT (vide infra) study, it is seen that there are two possibilities for F− binding with BPC, as shown in Fig. 9b and c. Because both proton signals diminish simultaneously, the second option, shown in Fig. 9c, is more likely.
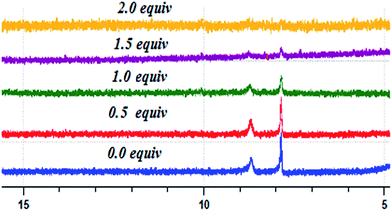 |
| Fig. 8
1H-NMR titration experiment of BPC with TBAF in DMSO-d6. | |
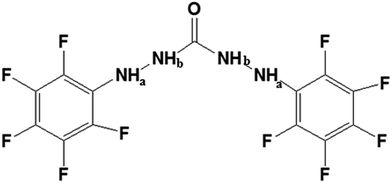 |
| Scheme 1 | |
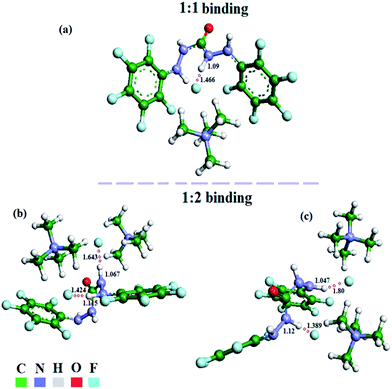 |
| Fig. 9 Energy-minimized structures of BPC with TBAF at (a) 1 : 1 binding and (b) and (c) 1 : 2 binding in different orientations. | |
The 1H-NMR titration experiment of BPC with Cd(NO3)2 in DMSO-d6 (Fig. S17†) depicts the slow kinetics of the interaction of the cation (Cd2+) with BPC compared to F−. Even after addition of 50 equivalents of Cd(NO3)2, the peak intensity decrease is nominal.
The 19F-NMR titration of BPC with Cd(NO3)2 in DMSO-d6 (Fig. S18†) depicts almost no change in either the peak shifting or intensity of the fluorine atoms, suggesting no participation of the pentafluorophenyl units in bond formation between Cd2+ and BPC.
Theoretical calculations
In order to draw a correlation between experimental and theoretical studies, modern DFT has been applied to the aforementioned systems. First, the geometry of the structure of BPC molecule was optimized (Fig. S23 and Table S5†). In the 1
:
1 BPC⋯F− adduct (Fig. 9a), it can be seen that the N008–H bond is projected towards the F− ion, while the H atoms are projected away from the anion. The N008–H distance, which is 0.86 Å in the crystal structure of the molecule, increases to 1.09 Å in the host–guest adduct. The bond distance between H and F− was found to be 1.466 Å, suggesting a typical H-bonding interaction.20 The energy of the adduct was found to be −2103.03 eV.
In 1
:
2 adduct formation between BPC and F−, out of several conformers, the two best possible were chosen. In the case shown in Fig. 9b, the adduct forms between two F− ions and two H atoms of the same arm of BPC. The N⋯H distances, which were 0.86 Å, initially elongated to 1.067 and 1.15 Å. The distances between F− and H were found to be 1.64 Å and 1.42 Å, which are typical of H-bonding interactions. In the other case, shown in Fig. 9c, the two F− atoms interact with the NHa hydrogen of one arm and the NHb hydrogen of the other arm. The corresponding distances are 1.047 and 1.12 Å. The respective H⋯F distances are 1.80 and 1.389 Å, which also depicts H-bonding interactions between BPC and F− anion. The host–guest interaction energy in Fig. 9b is −2417.0136 eV (with a HOMO–LUMO gap of 3.33 eV), while that in Fig. 9c is −2416.999 eV (with a HOMO–LUMO gap of 3.121 eV). The Cartesian coordinates of the geometry-optimized structures of the 1
:
1 and 1
:
2 adducts are shown in Tables S6–S8.†
DFT studies were also performed on BPC with NaOH (Fig. S24†). It was clear from these studies that with NaOH, BPC underwent deprotonation; this is in sharp contrast to F−, where a H-bonding interaction prevailed between the host and the guest. Three different situations were created to obtain insight into the acid–base interactions with and without aqueous medium. In the case shown in Fig. S24a,† the interaction of NaOH and BPC resulted in deprotonation of the Hb proton, which is more acidic than the Ha proton, and liberation of the (H)(Hb)2O molecule.
In the case shown in Fig. S24b,† when one H2O molecule is introduced to the system, a strong interaction is observed between Ha and the O of the OH− atom (1.45 Å); however, no deprotonation is observed. This may be due to the greater hydration of OH− ions with H2O molecules, which partially weakens the strength of the base.
In the third case, shown in Fig. S24c,† two BPC molecules interact with one NaOH molecule in the presence of one H2O molecule. The OH− interacts with and deprotonates one Hb atom of one BPC molecule, while the other remains intact. The Na+ is stabilised by the two BPC molecules, which fulfils its six coordination sites.
The Cartesian coordinates for the theoretical calculations of BPC with NaOH are summarized in Tables S9–S11.†
Inside living cells
Human lung carcinoma cell lines (A549) (Department of Biotechnology, National Institute of Technology (NIT), Durgapur-713209, West Bengal, India) were used to investigate the fluorescence emission of BPC and the quenching of fluorescence of BPC by Cd2+ inside living cells (Fig. 10). The cells were grown on sterile polylysine-coated cover glasses submerged in cell culture dishes containing 10 ml DMEM medium (Hi-Media) containing 10% FBS (Hi-Media) with 1% penicillin/streptomycin antibiotics in appropriate concentrations; the cells were then incubated in a CO2 incubator with a humidified atmosphere (95% air/5% CO2) at 37 °C for 1 day. The cells, after attaining about 70% confluence, were treated with 100 μM BPC in serum-free DMEM for 12 hours. Then, the cells were analysed microscopically at 100× magnification for the absorption and emission of fluorescence at 350 nm and 465 nm, respectively, using a florescence microscope (Zeiss Axio Observer) with a DAPI filter; the images were captured under DAPI and bright field backgrounds, and both images were then merged to observe the emission of fluorescence. Then, the same cells were treated with 100 μM Cd(NO3)2 for 12 hours, and the fluorescence quenching in the cells was observed.
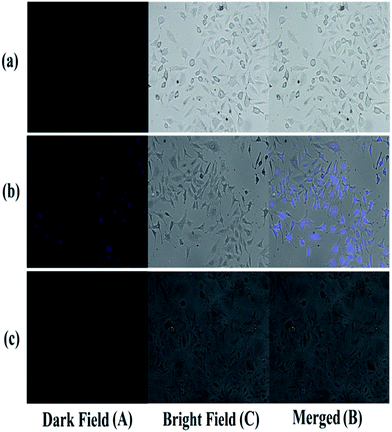 |
| Fig. 10 Microscopic live cell imaging study of HeLa cells taken with bright field (A) and dark fluorescence backgrounds (B); merged image of both backgrounds (C) to show the pattern of luminescence exhibited by all the cells in the microscopic field: (a) when the cells were untreated, (b) when the cells were treated with BPC and (c) when the BPC-treated cells were treated with Cd2+. | |
In order to study the ability of BPC to monitor pH changes inside living cells, the following experiment was performed (Fig. 11). At lower pH (i.e. under acidic conditions), all the cells showed increases in luminescence, whereas at higher pH, quenching of luminescence in the cells was observed. In Fig. 5, a decrease of emission intensity with increasing pH from 6 to 11 can be observed. The cells were cultured in PBS buffer, and BPC in DMSO was applied within the cells. HeLa cells (human cervical cancer) were used in this study to show the fluorescence response of BPC inside cancer cells under different pH conditions. The cells were cultured in DMEM (Dulbecco's Modified Eagle's Medium) with 10% FBS (fetal bovine serum) and appropriate penicillin and streptomycin antibiotics. The cells were maintained under three different pH conditions, acidic (pH 6.0), neutral (pH 7.0) and alkaline (pH 8.0), before treatment with BPC. Then, all the cells were treated with BPC (∼1 × 10−6 M) and allowed to take it up in order to exhibit fluorescence.
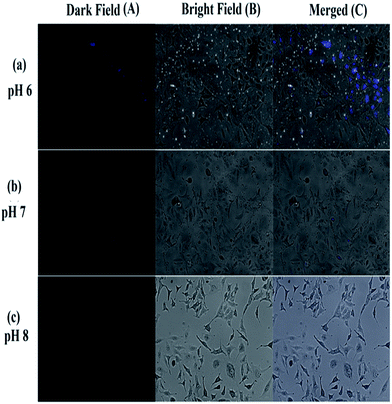 |
| Fig. 11 Microscopic live cell imaging study (pH = 6/7/8, respectively) of different HeLa cells maintained under different pH conditions treated with BPC, taken with bright field (A) and dark fluorescence backgrounds (B); the merged image of both backgrounds (C) shows the pattern of luminescence exhibited by all the cells in the microscopic fields. (a) At acidic pH, the cells exhibit higher fluorescence compared to the cells maintained in neutral (b) and alkaline conditions (c). The merged image pattern suggests that luminescence was observed in all the cells and that its intensity depends on the pH of the cell growth environment. | |
A performance index of Cd2+, F− and pH is presented in Table 2, where the performance of BPC is compared with existing literature data to prove that BPC is superior/comparable for sensing Cd2+, F− and pH in living cells.
Table 2 Performance indexing table of Cd2+, F− and pHa
Cd2+ recognition study |
Serial no. |
Ref. |
Sensor type |
Limit of detection (Cd2+) |
Sensors exhibiting dual sensing properties (for Cd2+ and F−), further showing that pH sensitivity is very rare.
|
1 |
21a
|
Quinoline-based |
0.6 × 10−6 M for FQDIPA |
0.8 × 10−6 M for FQDPA |
2
|
Present work
|
Urea-based (
BPC
)
|
2.8 × 10
−5
M
|
3 |
21b
|
Quinoline-based |
1.7 × 10−5 M |
4 |
21c
|
β-Cyclodextrin-based |
1.89 × 10−3 M |
F− recognition study |
Serial no. |
Ref. |
Sensor |
Limit of detection (F−) |
1 |
21d
|
Squaramide derivative |
4.90 × 10−6 M |
2 |
Present work |
Urea-based (BPC) |
5.75 × 10−6 M |
3 |
21e
|
Alizarin complex |
12.9 × 10−6 M |
4 |
21f
|
Organoboron-based |
0.015 × 10−3 M for compound 1, |
0.020 × 10−3 M for compound 2 in this work |
pH study |
Serial no. |
Ref. |
pH range |
Purpose |
1 |
21g
|
2.0 to 11.7 |
pH study |
2 |
Present work |
6.0 to 11.0 |
pH study and intracellular pH detection |
3 |
21h
|
3.21 to 3.50 |
Monitoring of lysosomal pH changes during physiological and pathological processes/in vitro pH sensing |
4.78 to 4.89 |
5.35 to 5.75 |
4 |
21i
|
5.0 |
Fluorescence lifetime measurement |
7.0 |
9.0 |
Electronic circuit fabrication based on different logic gates
The present pH sensor (BPC) can be further interfaced with suitable circuitry interfaced with appropriate programming for ease of access and enhancement of its practical applications (Fig. S25†). The recommended device is a micro footprint pH-monitoring subsystem (pH circuit, Atlas Scientific) that may be connected to this sensor to acquire pH-related data of solutions that alternate between particular pH ranges. The final output would be fed to a functional pin of Arduino UNO in order to interpret the pH measures as digital outputs, indicated by ON and OFF switching of LED and suitable display mechanisms.
The reversible spectrophotometric analysis of BPC with OH− and H+ impelled us to explore molecular logic gate-based complex circuit design. Molecular logic gates function by chemical input signals based on several spectroscopic findings; as a consequence, several computational complexities can be overcome with a complex circuit. The UV-Vis titration study of BPC with OH− generated a new response at 490 nm which is reversible with concomitant addition of H+. These reversible spectroscopic outcomes were used to design a Boolean algebraic logic gate-based circuit. The three chemical inputs are BPC (In1), OH− (In2) and H+ (In3). The two outputs are Y1 (224 nm) and Y2 (490 nm). The Y1 output is turned off (0 state) and Y2 is turned on (1 state) during operation. The AND–NOR–NOT–OR–XNOR–XOR based circuit is capable of performing complex logic operations in a single circuit (Fig. 12).9a,c
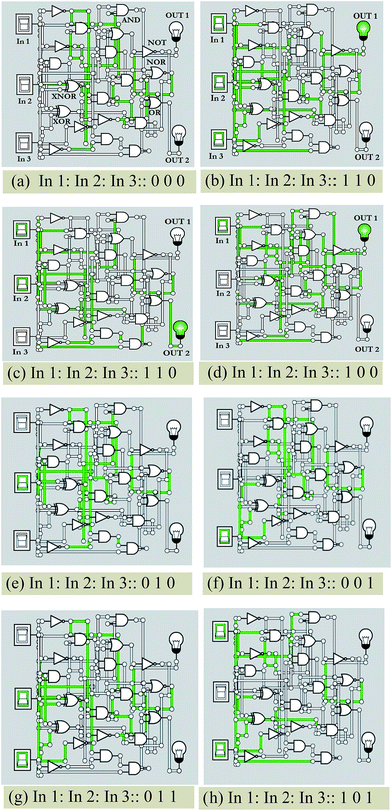 |
| Fig. 12 AND–NOR–NOT–OR–XNOR–XOR-based molecular logic circuit. | |
Conclusion
In summary, a highly novel urea-based ditopic chemosensor of Cd2+ and F− in organic–aqueous medium, BPC, has been synthesized. The chemosensor is also a good pH sensor in the range of 6 to 11. The sensing of Cd2+, F− and OH− was thoroughly evaluated through IR and CV and through UV-Vis, PL, and 1H-NMR spectroscopy as well as through mass spectrometry. Furthermore, the BPC chemosensor molecule was found to be cell permeable and non-cytotoxic; it could also detect Cd2+ inside cells. The pH-sensing application of this sensor was also demonstrated inside HeLa cells. In addition, the pH sensor BPC can be interfaced with suitable circuitry interfaced with appropriate programming for ease of access and enhancement of its practical applications. This easily prepared, promising sensor opens a new arena to synthesize similar molecules with improved multi-ion sensing abilities.
Acknowledgements
PB is thankful to Prof. Dr Harish Hirani, Director, CSIR-CMERI for his immense support of this work in CSIR-CMERI. Financial support received from CSIR-Supra Institutional research grant (ESC-0203/09) under the CSIR-XIIth five-year plan and the DST fast track project is gratefully acknowledged. ARC is also grateful for a Supra institutional research grant for her project fellowship. The authors are thankful to Ms. Meenakshi Sharma, NEIST for her support. PG is thankful to DST (GAP-183112) for his fellowship.
Notes and references
-
(a) H. N. Kim, W. X. Ren, J. S. Kim and J. Yoon, Chem. Soc. Rev., 2012, 41, 3210 RSC;
(b) C. N. McFarland, L. I. Bendell-Young, C. Guglielmo and T. D. Williams, J. Environ. Monit., 2002, 4, 791 RSC.
- L. Xue, G. Li, Q. Liu, H. Wang, C. Liu, X. Ding, S. He and H. Jiang, Inorg. Chem., 2011, 50, 3680 CrossRef CAS PubMed.
-
(a) R. T. Bronson, D. J. Michaelis, R. D. Lamb, G. A. Husseini, P. B. Farnsworth, M. R. Linford, R. M. Izatt, J. S. Bradshaw and P. B. Savage, Org. Lett., 2005, 7, 1105 CrossRef CAS PubMed;
(b) C. T. McMurray and J. A. Tainer, Nat. Genet., 2003, 34, 239 CrossRef CAS PubMed;
(c)
B. J. Alloway and E. Steinnes, in Cadmium in Soils and Plant, ed. M. J. McLaughlin and B. R. Singh, Kluwer Academic Publishers, Boston, 1999, p. 97 Search PubMed.
-
(a) C. Lu, Z. Xu, J. Cui, R. Zhang and X. Qian, J. Org. Chem., 2007, 72, 3554 CrossRef CAS PubMed;
(b) H. Li, Y. Yao, C. Han and J. Zhan, Chem. Commun., 2009, 4812 RSC;
(c) Q. Zhao, R.-F. Li, S.-K. Xing, X.-M. Liu, T.-L. Hu and X.-H. Bu, Inorg. Chem., 2011, 50, 10041 CrossRef CAS PubMed;
(d) L. Xue, C. Liu and H. Jiang, Org. Lett., 2009, 11, 7 Search PubMed.
-
(a) H. N. Kim, W. X. Ren, J. S. Kim and J. Yoon, Chem. Soc. Rev., 2012, 41, 3210 RSC;
(b) U. Fegade, H. Sharma and S. Attarde, J. Fluoresc., 2014, 24, 27 CrossRef CAS PubMed.
- R. Arabahmadi, M. Orojloob and S. Amani, Anal. Methods, 2014, 6, 7384 RSC.
-
(a)
https://drtijanadc.wordpress.com/2012/07/02/the-essentials-of-ph/
;
(b)
http://www.lifewithgreens.com/ph-and-your-health
;
(c)
http://www.mindbodygreen.com/0-3400/What-You-Need-to-Know-About-pH.html
;
(d)
http://www.google.co.in/patents/WO2004016297A1
.
-
(a) F. Doria, M. Folini, V. Grande, G. Cimino-Reale, N. Zaffaronib and M. Freccero, Org. Biomol. Chem., 2015, 13, 570 RSC;
(b) Y. Ni and J. Wu, Org. Biomol. Chem., 2014, 12, 3774 RSC;
(c) C. Y. Ang, S. Y. Tan and Y. Zhao, Org. Biomol. Chem., 2014, 12, 4776 RSC.
-
(a) P. Ghosh, B. G. Roy, S. Jana, S. K. Mukhopadhyay and P. Banerjee, Phys. Chem. Chem. Phys., 2015, 17, 20288 RSC;
(b) P. Ghosh and P. Banerjee, Phys. Chem. Chem. Phys., 2016, 18, 22815 Search PubMed;
(c) P. Ghosh and P. Banerjee, Chem. Phys., 2016, 478, 103–109 CrossRef CAS;
(d) P. Banerjee, S. Sproules, T. Weyhermuller, S. D. George and K. Weighardt, Inorg. Chem., 2009, 48, 5829 CrossRef CAS PubMed;
(e) P. Banerjee, A. Company, T. Weyhermuller, E. Bill and C. R. Hess, Inorg. Chem., 2009, 48, 2955 Search PubMed;
(f) S. Mandal, N. Paul, P. Banerjee, T. K. Mondal and S. Goswami, Dalton Trans., 2010, 39, 2726 Search PubMed.
-
(a) M. Irie, Chem. Rev., 2000, 100(5), 1685 CrossRef CAS PubMed;
(b)
J. Millman and A. Grabel, Microelectronics, McGraw-Hill, New York, 1988, ch. 6 Search PubMed;
(c)
M. M. Mano and C. R. Kime, Logic and Computer Design Fundamentals, Prentice-Hall, Upper Saddle 5 River, 4th edn, 2000 Search PubMed.
-
(a)
B. L. Feringa, Molecular Switches, Wiley-VCH Express, New York, 2001, p.37 CrossRef;
(b)
S. Salivahanan and S. Arivazhagan, Digital Circuits and Design, VikasPublishing House Pvt. Ltd, 2nd edn, 2004, ch. 3 Search PubMed;
(c) K. Rezaeian and H. Khanmohammadi, New J. Chem., 2015, 39, 2081 RSC.
-
(a) R. Ahlrichs, M. Bar, M. Haser, H. Horn and C. Kolmel, Chem. Phys. Lett., 1989, 162, 165 CrossRef CAS;
(b) A. Schafer, H. Horn and R. Ahlrichs, J. Chem. Phys., 1992, 97, 2571 CrossRef.
-
ShelXTL, v. 6.14, Bruker AXS Inc., Madison, WI, 2003 Search PubMed.
-
(a) A. Roy Chowdhury, P. Ghosh, B. G. Roy, S. K. Mukhopadhyay, P. Mitra and P. Banerjee, RSC Adv., 2015, 5, 62017 RSC;
(b) A. Roy Chowdhury, P. Ghosh, B. G. Roy, S. K. Mukhopadhyay, N. C. Murmu and P. Banerjee, Sens. Actuators, B, 2015, 220, 347 CrossRef.
- Y. Imai, T. Kawai and J. Yuasa, Chem. Commun., 2015, 51, 10103 RSC.
- J. Han and K. Burgess, Chem. Rev., 2010, 110(5), 2709 CrossRef CAS PubMed.
-
(a) R. Wang, Y. Chunwei, F. Yu and L. Chen, Trends Anal. Chem., 2010, 29(9) CrossRef;
(b) M. H. Lee, J. H. Han, J. H. Lee, N. Park, R. Kumar, C. Kang and J. S. Kim, Angew. Chem., Int. Ed., 2013, 52, 6206 CrossRef CAS PubMed;
(c) G. Despras, A. I. Zamaleeva, L. Dardevet, C. Tisseyre, J. G. Magalhaes, C. Garner, M. D. Waard, S. Amigorena, A. Feltz, J. M. Malleta and M. Collot, Chem. Sci., 2015, 6, 5928 RSC;
(d) B. Shi, Y. Sua, L. Zhanga, R. Liua, M. Huanga and S. Zhaoa, Biosens. Bioelectron., 2016, 82, 233 CrossRef CAS PubMed;
(e) X.-D. Liu, Y. Xu, R. Sun, Y.-J. Xu, J.-M. Lu and J.-F. Ge, Analyst, 2013, 138, 6542 RSC;
(f) Y. Xu, Y. Liu and X. Qian, J. Photochem. Photobiol., A, 2007, 190, 1 CrossRef CAS;
(g) P. T. Snee, R. C. Somers, G. Nair, J. P. Zimmer, M. G. Bawendi and D. G. Nocera, J. Am. Chem. Soc., 2006, 128(41), 13320 CrossRef CAS PubMed.
- A. Roy Chowdhury, P. Ghosh, S. K. Saha, P. Mitra and P. Banerjee, Spectrochim. Acta, Part A, 2014, 124, 492 CrossRef CAS PubMed.
-
(a) P. Ghosh, N. Kumar, S. K. Mukhopadhyay and P. Banerjee, Sens. Actuators, B, 2016, 224, 899 CrossRef CAS;
(b) P. Ghosh, B. G. Roy, S. K. Mukhopadhyay and P. Banerjee, RSC Adv., 2015, 5, 27387 RSC.
-
(a) U. N. Yadav, P. Panta, D. Sharma, S. K. Sahoo and G. S. Shankarling, Sens. Actuators, B, 2014, 197, 73 CrossRef CAS;
(b) A. Satheshkumar and K. P. Elango, Dyes Pigm., 2013, 96, 364 CrossRef CAS.
-
(a) H. Tian, B. Li, J. Zhu, H. Wang, Y. Li, J. Xu, J. Wang, W. Wang, Z. Sun, W. Liu, X. Huang, X. Yan, Q. Wang, X. Yao and Y. Tang, Dalton Trans., 2012, 41, 2060 RSC;
(b) Y. Ma, F. Wang, S. Kambam and X. Chen, Sens. Actuators, B, 2013, 188, 1116 CrossRef CAS;
(c) J.-T. Zhang, L. Wang, J. Luo, A. Tikhonov, N. Kornienko and S. A. Asher, J. Am. Chem. Soc., 2011, 133, 9152 CrossRef CAS PubMed;
(d) H. Niu, Q. Shu, S. Jin, B. Li, J. Zhu, L. Li and S. Chen, Spectrochim. Acta, Part A, 2016, 153, 194 CrossRef CAS PubMed;
(e) Y. Jia, Z. Li and W. Shi, Sens. Actuators, B, 2013, 188, 576 CrossRef CAS;
(f) X. Y. Liu, D. R. Bai and S. Wang, Angew. Chem., 2006, 118, 5601 CrossRef;
(g) F. Doria, M. Nadai, G. Sattin, L. Pasotti, S. N. Richter and M. Freccero, Org. Biomol. Chem., 2012, 10, 3830 RSC;
(h) X.-D. Liu, Y. Xu, R. Sun, Y.-J. Xu, J.-M. Lu and J.-F. Ge, Analyst, 2013, 138, 6542 RSC;
(i) S. Ogikubo, T. Nakabayashi, T. Adachi, Md. S. Islam, T. Yoshizawa, M. Kinjo and N. Ohta, J. Phys. Chem. B, 2011, 115, 10385 CrossRef CAS PubMed.
Footnotes |
† Electronic supplementary information (ESI) available: UV-Vis, ESI-MS, 1H-NMR and FT-IR spectra of BPC are provided. See DOI: 10.1039/c6ay02656g |
‡ Present address: Department of Biotechnology, Maharishi Markandeswar University, Mullana-Ambala, Haryana, 133203, India |
|
This journal is © The Royal Society of Chemistry 2017 |