DOI:
10.1039/C6AY02361D
(Paper)
Anal. Methods, 2017,
9, 154-162
A label-free SERRS-based nanosensor for ultrasensitive detection of mercury ions in drinking water and wastewater effluent†
Received
23rd August 2016
, Accepted 21st November 2016
First published on 22nd November 2016
Abstract
Precisely probing mercury ions (Hg2+) is of essential importance to human health and environmental protection. Although numerous methods have been developed to detect Hg2+ in water, challenges still remain for rapid, accurate, and reliable detection of Hg2+ at the ppt level. In this study, a novel label-free Fe3O4@Ag based surface-enhanced Raman resonance scattering (SERRS) nanosensor has been developed for ultrasensitive and highly selective detection of Hg2+. The detection mechanism is on the basis of competitive binding interaction of Hg2+ and malachite green (MG) with nano-sliver on Fe3O4@Ag magnetic beads (MBs). In the absence of Hg2+, the Raman signal intensity of MG is significantly enhanced by the nano-silver when MG adsorbs on the nano-silver surface via one nitrogen atom. In the presence of Hg2+, the redox reaction occurring between zero-valent nano-silver and Hg2+ leads to the formation of a Ag/Hg amalgam at the Fe3O4@Ag surface, which prevents the adsorption of MG on the nano-silver surface. Thereby, the SERRS signal intensity of MG proportionally decreased with increasing Hg2+ concentration. Under optimized conditions, the proposed label-free nanosensor showed unprecedented Hg2+ detection sensitivity of 10 pM (2 ppt) with excellent selectivity. This simple sensor system can be directly applied for an inexpensive and easy-to-use monitoring method of Hg2+ in drinking water and wastewater treatment effluents that contains complicated organic and inorganic interferents.
Introduction
Toxic heavy metal ions pose a serious threat to the environment and human health even at ultra-low concentrations. Mercury ions (Hg2+), one of the most prevalent toxic metals, have attracted considerable attention because of their high toxicity and bio-accumulation through drinking water and the food chain.1,2 When accumulated in the human body, mercury may cause irreversible damage to DNA, the brain, and the central nervous system and cause other chronic diseases.3 Therefore, ultrasensitive, highly selective, and wide linear range detection of Hg2+ ions in water samples, especially without complicated sample pretreatment processes, is essential for environmental and food monitoring. Traditional detection technologies, such as cold vapor atomic absorption spectrometry, atomic fluorescence spectrometry, and inductively coupled plasma mass spectrometry, have been intensively developed for Hg2+ ion quantification.4–6 Most of them require complicated sample preparation, bulky and expensive instrumentation, and trained personnel. Numerous optical sensors have been developed for the Hg2+ ion detection based on colorimetric, fluorescence, plasmon resonance and surface-enhanced Raman scattering (SERS).7–10 These sensors were generally constructed using Hg2+ sensitive molecular probes and labeling tags, such as aptamers (e.g. T–T mismatch DNA sequences), metal ion-specific organic ligands, proteins, and nanomaterials (e.g. quantum dots). However, the application of molecular probes and labeling tags not only increases the complication and cost of the sensing system and decreases their selectivity, but also makes sensors more susceptible to the complicated matrix in real samples. Moreover, the sensitivity of most of these optical sensors is still insufficient to detect ultra-low concentration mercury ions (e.g. ppt level), limiting their applications in precise measurements of Hg2+ ions in environmental and food samples. However, SERS based nanosensors have demonstrated great potential for the ultrasensitive detection of trace targets (ppt and even single molecule level) through the extraction of molecular fingerprint information.11–14 A rapid direct detection of Hg2+ based on SERS by using silver colloid as an enhancement substrate has been developed with a sensitivity of 90 pM.15 However, this nanosensor was never used to detect Hg2+ in real samples, which might be attributed to the aggregation of silver nanoparticles in the presence of sample matrix. A dealloyed nanoporous gold (NPG)/aptamer based hybrid SERS nanosensor demonstrated an ultrasensitivity of 1 pM for Hg2+ detection, but the complicated and expensive construction process of the NPG SERS substrate and the usage of Cy5-labeled aptamer made it difficult for the practical application of Hg2+ detection in real samples.16 Therefore, it is of great significance to develop simple, highly sensitive, cost-effective and label-free sensors for Hg2+ detection in drinking water and wastewater.
Various noble metal nanostructures such as gold and silver can provide strongly enhanced SERS signals because of their distinctive unique surface plasmon resonance and optical and chemical properties.17,18 Silver nanostructures are much more efficient Raman signal-enhancing materials and give rise to SERS signals that are 10 to 100-fold higher than those of similar gold nanostructures.19 Fe3O4@Ag magnetic beads (MBs) have been widely used as a SERS substrate thanks to their good magnetic responsiveness and relatively facile fabrication process.20 Furthermore, Fe3O4@Ag MBs can be captured, aggregated and removed easily without centrifugation and/or filtering with the help of an external magnetic field. High sensitive SERS sensors require Raman tag molecules attached on or near the surface of SERS substrates because short-range chemical enhancement (CE) requires chemical bonding and long-range electromagnetic (EM) enhancement is strongly distance-dependent.21 Previous research has demonstrated that malachite green (MG), a popular cationic triphenylmethane dye, was an excellent Raman tag because it could tightly bind to nano-silver surfaces via its nitrogen atom to obtain typical strong Raman peaks.22 On the other hand, previous reports also proved that Hg2+ could selectively integrate into silver nanoparticles to form stable Ag/Hg amalgams through the redox reaction occurring between zero-valent nano-silver and Hg2+.13,15,23
Herein, a label-free, rapid, and cost-effective nanosensor has been, for the first time, developed for ultrasensitive optical detection of Hg2+ ions using Fe3O4@Ag as a plasmonic sensing platform and MG as a competitive Raman tag. When MG molecules are adsorbed on the surface of Fe3O4@Ag MBs by a Ag–dimethylamino bond, the SERRS signals increased significantly due to CE and EM enhancement. However, the adsorption amount of MG proportionally decreased with increasing Hg2+ concentration, thus leading to the decrease of SERS signal intensity. This simple nanosensor showed an unprecedented sensitivity of 10 pM (2 ppt) for Hg2+ ion detection, which was 100 times more sensitive than that of conventional optical sensors and about 3 orders of magnitude lower than the U.S. EPA-defined maximum level (10 nM) in drinking water.24 The proposed nanosensor also showed high selectivity toward Hg2+, even in the presence of other competitive heavy metal ions. To our best knowledge, the presented SERRS nanosensor, for the first time, could be directly applied for Hg2+ detection in drinking water and wastewater without complicated pretreatment. The proposed method was green because the Ag/Hg amalgam on the Fe3O4@Ag surface was stable and easily removed from the water environment by magnetic separation.
Experimental section
Materials and reagents
Malachite green chloride (Product of Switzerland), and Hg(NO3)2 were obtained from the National Center of Analysis and Testing for Nonferrous Metals and Electronic Materials (NCATN, Beijing, China), and the stock solution of Hg2+ was prepared by diluting standard solution of Hg2+ (1000 μg mL−1) in ultrapure water. Ferric chloride hexahydrate (FeCl3·6H2O), tetraethoxysilane (TEOS), sodium acetate (NaAc), silver nitrate (AgNO3, 99%), butylamine, adenine, polyethylene glycol (MW 3400), PEG6000, and poly(allylamine hydrochloride) (PAH, MW 70 kDa) were purchased from Sigma-Aldrich (Shanghai, China) and used as received. Ammonia solution (28.0–30.0 wt%) was obtained from Sanchun Pure Chemical Company (Beijing, China). Other chemicals, unless specified, were of reagent grade and purchased from Beijing Chemical Reagent Company (Beijing, China).
Fabrication of Fe3O4@Ag MBs
The Fe3O4@Ag MBs were synthesized according to a previously reported method.25–27 First, Fe3O4 MBs were prepared through a modified solvothermal reaction.25 Typically, 1.35 g of FeCl3·6H2O was dissolved in 40 mL of ethylene glycol under magnetic stirring for 30 min. Subsequently, 1 g of PEG6000 and 2.7 g of NaAc were added and stirred until the reactants were fully dissolved. Then, the mixture was transferred into a Teflon-lined autoclave (50 mL) and heated at 200 °C for 12 h. The collected products were clearly washed with deionized water and ethanol, and were dried under vacuum at 60 °C. To prepare Fe3O4@SiO2 MBs, the 0.1 g as-prepared Fe3O4 MBs were dispersed in a deionized water–ammonia–ethanol solution mixture (5
:
4
:
5, v/v) by sonication for 15 min. 100 μL of TEOS was slowly dropped into the above solution by vigorous sonication, and the mixture was kept for 45 min at RT. The product was washed with deionized water and ethanol, and dried in a vacuum oven at 60 °C. The surface amination of Fe3O4@SiO2 MBs was achieved through a PAH self-assembly process under sonication. Typically, 0.25 g PAH was dissolved in 50 mL de-ionized water by ultrasonication, and PAH gradually self-assembled on the Fe3O4@SiO2 MBs. Then, the resulting Fe3O4@SiO2@PAH MBs were magnetically separated and rinsed with deionized water. Next, Fe3O4@SiO2@PAH MBs and 3–5 nm AuNPs were mixed to form Fe3O4@SiO2@PAH–AuNPs through the electrostatic interaction. After sonication for 1 h, the mixture was separated by using a magnet and washed with deionized water. For preparing Fe3O4@SiO2@Ag, 10 mg Fe3O4@SiO2–Au NPs was dispersed in 100 mL AgNO3 solution (0.25 mM, containing 0.2 wt% PVP). An excessive amount of formaldehyde (150 μL) and ammonia solution (300 μL) was added in sequence. The Fe3O4@Ag MBs were obtained within 2 min under sonication at 30 °C. The products were magnetically separated and washed with deionized water to remove the excess PVP.
The as-prepared Fe3O4@Ag MBs were spherical with a mean diameter of 400 ± 50 nm, in which numerous bumps were present on the surface of the MBs according to the TEM and SEM images (Fig. S1†). These bump structures, greatly increasing the surface area of Fe3O4@Ag MBs, are beneficial for increasing the adsorption of MG and the enhancement of SERRS signals. The magnetic properties of Fe3O4@Ag MBs were further examined using a superconducting quantum interference device magnetometer (SQUID, MPMSXL-7) at 300 K. As shown in Fig. S2,† the saturation magnetization values (MS) of Fe3O4, Fe3O4@SiO2–Au seed, and Fe3O4@Ag were found to be 88.7, 41.6, and 32.5 mu g−1, respectively. The MS values showed a decreasing trend after SiO2-coating and Ag shell-formation, which is mainly attributed to the mass effect of silica and silver and diamagnetic shielding. However, the Fe3O4@Ag MBs could be magnetically concentrated and readily picked up using a small magnet.
Determination of Hg2+ by using the Fe3O4@Ag-based SERRS nanosensing platform
Fig. 1 showed the detection process of Hg2+ using a Fe3O4@Ag-based SERRS nanosensing platform. The standard Hg2+ solution from 10−5 mol L−1 to 10−11 mol L−1 was prepared with Hg2+ stock solution. MG solutions of 10−4 mol L−1, 10−5 mol L−1 and 10−6 mol L−1 were also prepared with stock solution. For the SERRS measurement of Hg2+, 25 μL of Hg2+ solution and 25 μL of MG solution were mixed with 100 μL Fe3O4@Ag MBs in an EP tube (200 μL), which was shaken in a TMR Heating ThermoMixer (1200 rpm). Afterward, the mixtures were separated with a magnet, and the supernatant was removed. The MBs were washed with purified water thrice through an ultrasonic cleaner. Then, 10 μL of purified water was added to the EP tube, and the MBs were thoroughly mixed again. Subsequently, 2 μL of the sample solution was dropped on a 50 nm gold film deposited on a glass plate. To achieve favorable reproducibility which is essential to SERS detection, the magnetic nanoparticles were dispersed on a Au-coated Si plate as uniformly as possible. Besides, eight replicate measurements were performed for each sample for reducing error. Finally, the SERRS spectra of the MG adsorbed on the MBs were detected by Raman spectroscopy after drying.
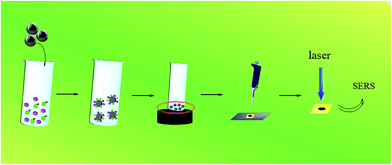 |
| Fig. 1 Schematic process of Hg2+ detection based on the Fe3O4@Ag-based SERRS nanosensing platform. | |
To evaluate the potential matrix effects of the environmental samples on Hg2+ detection, spiked samples of drinking water and several wastewater effluent samples, including regeneration water (Qinghe Reclamation Water Plant, Beijing), ultrafiltration membrane effluent (Qinghe Wastewater Treatment Plant, Beijing), and secondary sedimentation effluent (Gaobeidian Wastewater Treatment Plant, Beijing), were tested at concentrations of 1000 nM, 10 nM, and 0.1 nM.
Apparatus
The SERRS spectra were collected by using an inVia confocal Raman microscope (British Renishaw Company) with a Renishaw CCD detector. SERRS measurements were obtained with a 633 nm excitation laser, 50× long distance objective, and 1200 lines per mm gratings. The pH measurements were obtained with a pH meter (FE20-FiveEasy from METTLER TOLEDO). TEM images were obtained using a Hitachi transmission electron microscope (H-7650B, Japan). Scanning electron microscopy (SEM) images were captured using an S-4800 scanning electron microscope (Hitachi, Japan). TMR Heating ThermoMixers and KQ-250DB numerically controlled ultrasonic cleaners were used in the experiment.
Results and discussion
Detection mechanism of Hg2+ using a Fe3O4@Ag-based SERRS nanosensing platform
Fig. 2A showed the SERS-based label-free sensing mechanism of Hg2+ detection using Fe3O4@Ag MBs through combining MG as Raman tags. MG embraces several typical SERS peaks including 1176, 1217, 1370 and 1617 cm−1, attributed to ring C–H in-plane bending, C–H rocking, N-phenyl stretching, and ring C–C stretching, respectively (Fig. 2B).15 The MG tags have a S0 → S1 transition mode centered at ∼620 nm wavelength and, thus, a 633 nm excitation laser can yield a resonant vibration of MG.28 The resultant SERRS signals of MG are ∼3–5 orders of the magnitude stronger than the regular SERS ones for a low detection limit and ultrahigh sensitivity. The peak at 1617 cm−1 was used for quantification in the detection system.29,30 The Hg2+ sensitivity of the Fe3O4@Ag based SERRS sensor relies on the changes of the MG Raman intensity caused by the formation of the Ag/Hg amalgam at the surface of Fe3O4@Ag MBs, which resulted from the redox reaction between zero-valent nano-silver and Hg2+ (Fig. 2A). In the absence of Hg2+, the MG Raman tags adsorbed on the silver surface of Fe3O4@Ag MBs by dimethylamino groups in a tilted upright configuration, as verified by the strongly enhanced in-plane vibration modes of MG.29,30 An enhanced SERRS signal was detected by Raman spectroscopy (Fig. 2A). When Hg2+ and MG were simultaneously added to the Fe3O4@Ag MB solution, the formation of the Ag/Hg amalgam at the surface of the Fe3O4@Ag MBs could affect the SPR properties of nano-silver which led to the decay of EM enhancement of the substrates, and prevented the adsorption of MG on the nano-sliver surface.13,15 As a result, the MG tags are pulled away from the Fe3O4@Ag substrate, resulting in a decrease in the SERRS signals of the MG tags. Fig. 2B demonstrated that the addition of low Hg2+ concentration could result in the decrease of the SERRS signals, and higher Hg2+ concentration induced a lower SERRS signal. The bands between 1359 and 1400 wave numbers changed in high concentration of Hg2+, but the reason was not clear. As a control, when only Hg2+ was added into the Fe3O4@Ag MB solution, no SERS signal could be detected (d curve in Fig. 2B). Because the amount of MG adsorbed on the Fe3O4@Ag substrate has a linear correlation with the number of Hg2+, the Hg2+ concentrations can be determined from the decrement of the SERRS intensity of the MG tags.
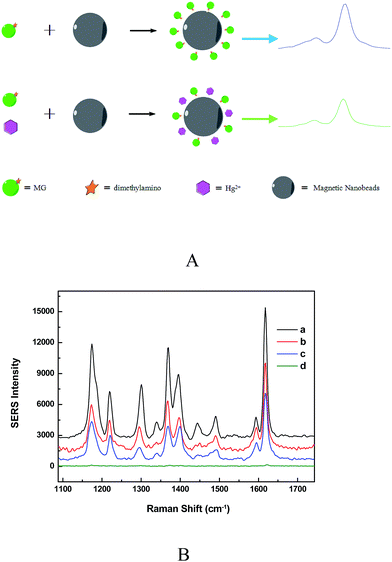 |
| Fig. 2 (A) Label-free SERS sensing mechanism of Hg2+ detection using Fe3O4@Ag MBs through combining MG tags. (B) SERS spectra of MG (10−5 mol L−1) adsorbed on Fe3O4@Ag MBs without Hg2+ concentration (a curve) and with 100 pM (b curve) and 10 nM (c curve) Hg2+; d curve was a control experiment using Fe3O4@Ag MBs with 10 nM Hg2+ and without MG. Detection conditions: laser wavelength 633 nm, accumulation times 1, exposure time 10 s, and laser power 1 mW. | |
Optimization of detection conditions
The adsorption time of MG on Fe3O4@Ag MBs was an important key factor for the Hg2+ detection period. The adsorption kinetics exhibited the preliminary phase of rapid sorption and reached a steady state within a short period of 20 min (Fig. S3†). Considering the shorter detection period, an incubation time of 20 min was used to obtain a stable SERRS signal in the following experiments.
Although the SERRS intensity would become weak with decreasing the MG concentration, the lower concentration of MG is beneficial for the effective competition of Hg2+ and yield better sensitivity according to the abovedescribed competitive detection mechanism. To experimentally prove this assumption and determine the optimum detection conditions, a sensitivity index (ε) was introduced:
|  | (1) |
where,
I0 is the SERRS signal intensity of the mixture of MG and Fe
3O
4@Ag,
Ib is the background SERRS intensity of Fe
3O
4@Ag without MG, and
Is is the SERS signal intensity of the mixture of MG and Fe
3O
4@Ag after addition of 100 pM Hg
2+. For a certain concentration of Fe
3O
4@Ag MBs, the higher the MG concentration, the more the amount of MG adsorb on the surface of Fe
3O
4@Ag MBs, leading to stronger SERRS signal intensity (
Fig. 3A). For the practical application of the sensor, the most acceptable MG concentration was chosen according to the following criteria: (1) the signal-to-noise ratio should be as high as possible; therefore, the MG solution with high concentrations (10
−4, 10
−5, and 10
−6 M) was further applied for the sensitivity index experiments; and (2) the sensitivity index should be as low as possible, and favorably below than 0.90 to be suitable for the desired dynamic range in practical applications.
Fig. 3B shows the sensitivity index calculated from the SERRS signal intensity in the presence of Hg
2+ and in the absence of Hg
2+. Therefore, 10
−5 M of MG was applied for subsequent Hg
2+ measurements.
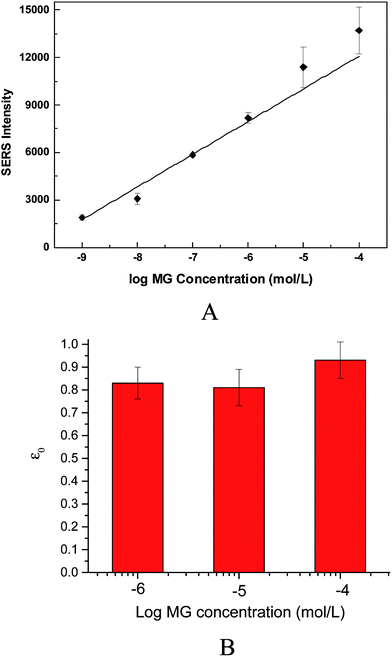 |
| Fig. 3 (A) The SERRS signal intensity of different concentrations of MG at 20 min incubation. (B) Sensitivity index was calculated from the fluorescence intensity with 100 pM Hg2+ (Is) and in the absence of targets (I0). The error bars represent standard deviation from eight measurements. | |
Several studies have demonstrated that the adsorption of MG on nanomaterials is greatly affected by the pH of the solutions.31 On the other hand, it is better to detect Hg2+ under acidic conditions because the formation of mercury ions is dependent on the pH of the solution.32 Therefore, the effect of pH on the MG SERRS intensity was investigated. As shown in Fig. S4,† the SERRS values were very small at a low pH (<2), increased obviously from 3 to 4.5. At a low pH, few MG molecules could adsorb on the nano-silver surface of Fe3O4@Ag MBs, which is almost consistent with those of previous reports.33,34 The appearance may be attributed to the following factors. First, the absorption of the hydrogen ions (H+) onto the nano-silver surface produced a repulsive force to hinder MG adsorption. Second, the excess H+ ions may compete with MG for the adsorption sites of Fe3O4@Ag MBs. At a pH value higher than 4, the SERRS signal slightly decreased. Therefore, Hg2+ determination could be performed at pH 4–6.
Determination of Hg2+ by using the Fe3O4@Ag SERRS nanosensing platform
On the basis of the results obtained above, we applied a Fe3O4@Ag SERRS nanosensing platform to detect Hg2+ using a homogeneous competitive detection mechanism. Different concentrations of Hg2+ solutions were initially mixed with a fixed concentration of Fe3O4@Ag MBs and MG solution and incubated for 20 min. During incubation, the amount of Hg2+ in the sample was expected to compete with MG to react with the limited sites of the nano-silver surface, thereby producing a target concentration-dependent SERRS signal intensity, which was the basis of quantitative detection. Fig. 4A shows the SERRS spectra of the MG/Fe3O4@Ag SERRS nanosensing system obtained with various concentrations of Hg2+. One can see that the introduction of Hg2+ at different concentrations to the mixture induced proportional decreases in the SERRS signal. Calibration curves (Fig. 4B) were obtained with a MG concentration of 10−5 mol L−1, which were normalized by expressing the signal of each standard point as the ratio of the maximum response [SERRS signal/SERRS signal max]. The SERRS signal intensity exhibited a linear trend with Hg2+ concentrations (log) ranging from 10−5 mol L−1 to 10−11 mol L−1 (R2 = 0.9923). As seen in Fig. 4B, the SERRS signal proportionally decreased as the concentration of Hg2+ was increased, which could be established by a dose–response curve (each being the average of three independent curves) for the detection of Hg2+ over a concentration range from 0 to 10 μM. The detection limits of 10 pmol L−1 (2 ppt) for Hg2+ were obtained from the calibration curve based on the average standard deviation of measurements (σ) and slope of dose–response (S) fitting curve as 3σ/S. Such high sensitivity is attributed to enhanced SERRS intensity of the Fe3O4@Ag-based nanosensing platform as well as the EM enhancement between Fe3O4@Ag MBs and gold film,35,36 and the higher binding ability of Hg2+ and the nano-silver surface of Fe3O4@Ag than the adsorption ability of MG on the nano-silver surface of Fe3O4@Ag. The detection limit obtained was several grades higher than that of a fluorescence sensor (4.5 nM),37 gold nanoparticle based electrochemical sensor (0.5 nM),14 and Au NP-enhanced SPR sensor (5 nM),38 and comparable to the plasmon-enhanced infrared spectroscopy (37 ppt),39 Ag nanoparticle colloid based SERS sensor (90 pM),15 and the dealloyed nanoporous gold (NPG)/aptamer-based nanosensor (1 ppt).16 However, our proposed sensor can be directly applied for the detection of Hg2+ in drinking water or wastewater samples without complicated pretreatment. In addition, compared to the sensors mentioned above, the developed nanosensor herein is simpler and greener because the Ag/Hg amalgam is stable and residual MG can easily be removed by the addition of Fe3O4@Ag MBs after detection.
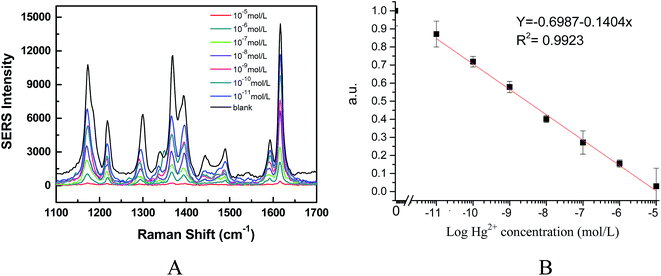 |
| Fig. 4 (A) Concentration-dependent SERS spectra of MG system in the presence of Hg2+ with its concentration ranging from 10−5 mol L−1 to 10−11 mol L−1. (B) The linear relationship between ISERS and Hg2+ concentration (R2 = 0.9923). The error bars represent standard deviation from eight measurements. Detection conditions: laser wavelength 633 nm, accumulation times 1, exposure time 10 s, and laser power 1 mW. | |
Selectivity of the sensing system
An essential feature of a practical heavy metal ion sensor is its selectivity to the other targets contained in natural water bodies in field measurements. Several metal ions, including Pb2+, Mn2+, Cd2+, Ca2+, Co2+, Zn2+, Cu2+, and Mg2+, at concentrations up to 10−4 M were tested under the same experimental conditions. As shown in Fig. 5, although the concentration of Pb2+, Co2+, Zn2+, and Cu2+ is 10 times higher than that of Hg2+ concentration, the interference of these ions only resulted in a slight decrease of SERRS intensity and could not cause a decrease as much as that induced by Hg2+. The other metal ions at high concentrations had less influence on the SERRS intensity of the system. Meanwhile, Hg2+ and three metal ions (Mn2+, Ca2+, Cu2+) were combined to form a metal ion soup as a sample for the anti-jamming capability testing of the nanosensor, and the reduction of the MG-tag SERRS intensity was almost similar as that of isolated Hg2+. These results indicated that the proposed method exhibited high selectivity for Hg2+ determination in a complex solution. The stronger redox reaction ability of nano-silver for the Hg2+ ions compared with those of the other metal ions contributed to the high specificity of the assay.
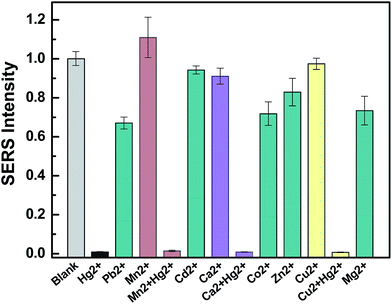 |
| Fig. 5 Selectivity of the MG/Fe3O4@Ag based sensing system. The concentration of Pb2+, Mn2+, Cd2+, Ca2+, Co2+, Zn2+, Cu2+, and Mg2+ is 10−4 mol L−1, and the concentration of Hg2+ is 10−3 mol L−1. The error bars represent standard deviation from eight measurements. | |
Analysis of environmental water samples
The high sensitivity and selectivity of the SERRS sensor can be even retained in the environmental water samples. Several samples spiked with Hg2+, with concentrations of 1000, 10, and 0.1 nmol L−1, were tested by spiking drinking water and several wastewater effluent samples, including regeneration water (Qinghe Reclamation Water Plant, Beijing), ultrafiltration membrane effluent (Qinghe Wastewater Treatment Plant, Beijing), and secondary sedimentation effluent (Gaobeidian Wastewater Treatment Plant, Beijing). The Raman spectra of these water samples were initially detected by mixing a water sample and Fe3O4@Ag MBs, and the SERRS intensity is negligible compared to that of 10−5 M MG (Fig. 6). The SERRS signal proportionally decreased with the increase of the spiked Hg2+ concentrations. The samples spiked with different concentrations of Hg2+ were detected according to the method described above with three replicates. The results are summarized in Table 1. The recovery of all measured samples was between 70 and 135%, and the parallel tests showed that the RSDs were less than 12%. These results indicated that the possible interference from the different background composition of water samples on SERRS-based nanosensor analysis was negligible. The developed nanosensor system was successfully applied to Hg2+ analysis in environmental water samples without complicated pretreatment.
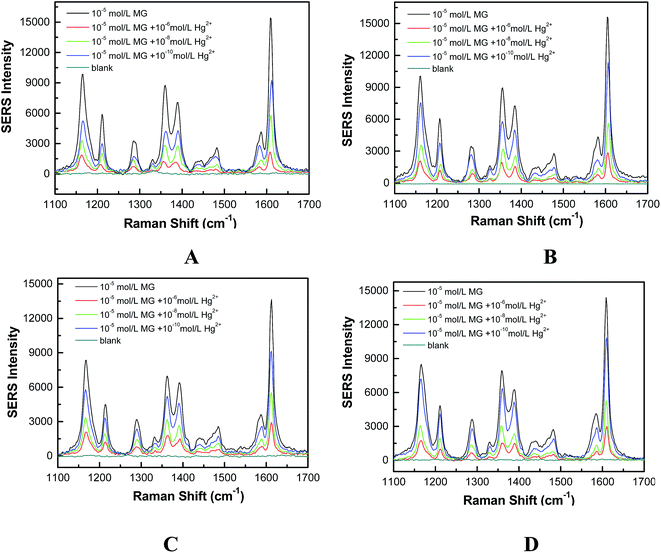 |
| Fig. 6 (A) Raman spectra of tap water without Hg2+ and MG (blank) and with MG and spiked with different concentrations of Hg2+; (B) Raman spectra of secondary sedimentation effluent without Hg2+ and MG (blank) and with MG and spiked with different concentrations of Hg2+; (C) Raman spectra of regeneration water without Hg2+ and MG (blank) and with MG and spiked with different concentrations of Hg2+; (D) Raman spectra of ultrafiltration-membrane effluent without Hg2+ and MG (blank) and with MG and spiked with different concentrations of Hg2+. Detection conditions: laser wavelength 633 nm, accumulation times 1, exposure time 10 s, and laser power 1 mW. | |
Table 1 Determination of Hg2+ in environmental water samples using the proposed method. The RSD of each sample comes from eight measurements
Samples |
Spiked Hg2+ concentration (nM) |
Detected Hg2+ concentration (nM) |
Recoveries (%) |
RSD (%) |
Tap water |
1000 |
1165 |
116.5 |
0.4 |
10 |
10.6 |
106.0 |
1.8 |
0.1 |
0.133 |
133.0 |
1.3 |
Regeneration water |
1000 |
660.7 |
66.07 |
11.2 |
10 |
9.675 |
96.75 |
0.94 |
0.1 |
0.074 |
74.13 |
7.01 |
Secondary sedimentation effluent |
1000 |
932 |
93.2 |
0.6 |
10 |
1.236 |
123.6 |
1.1 |
0.1 |
0.106 |
106.0 |
0.8 |
Ultrafiltration membrane effluent |
1000 |
933.2 |
93.32 |
9.4 |
10 |
9.528 |
95.28 |
0.97 |
0.1 |
0.076 |
76.25 |
3.0 |
Conclusion
In summary, a unique Fe3O4@Ag-based SERS nanosensing platform has been developed for Hg2+ determination in real water samples by using a MG tag. This method combined the merits of selective interactions between nano-silver and Hg2+, as well as the excellent SERS enhanced properties of Fe3O4@Ag. The proposed SERRS-based nanosensor had the following unique advantages. First, the simple nanosensing platform shows an unprecedented sensitivity of 10 pM (2 ppt) for Hg2+ ion detection. Second, the proposed sensing method does not need complicated labeling and sample-pretreatment processes, which are essential for the practical application of the nanosensor. Third, the nanosensor can directly be used to detect Hg2+ in real environmental water samples such as effluents or water bodies with good recovery, precision, and accuracy, indicating that it is less susceptible to water matrix effects. In addition, the proposed method provides a new and green solution for Hg2+ detection in environmental samples because of the high stability of the Ag/Hg amalgam that can be easily removed by magnetic separation.
Acknowledgements
This research was financially supported by the National Natural Science Foundation of China (21077063 and 21277173), the National Instrument Major Project of China (2012YQ3011105), the Special Fund of State Key Joint Laboratory of Environment Simulation and Pollution Control (14K01ESPCT), and the Basic Research Funds in Renmin University of China from the Central Government (13XNLJ01).
References
- H. N. Kim, W. X. Ren, J. S. Kim and J. Yoon, Fluorescent and colorimetric sensors for detection of lead, cadmium, and mercury ions, Chem. Soc. Rev., 2012, 41, 3210–3244 RSC.
- J. K. Virtanen, T. H. Rissanen, S. Voutilainen and T. P. Tuomainen, Mercury as a risk factor for cardiovascular diseases, J. Nutr. Biochem., 2007, 18, 75–85 CrossRef CAS PubMed.
- T. W. Clarkson, The three modern faces of mercury, Environ. Health Perspect., 2002, 110, 11–23 CrossRef CAS PubMed.
- M. Resano, J. Briceno and M. A. Belarra, Direct determination of Hg in polymers by solid sampling-graphite furnace atomic absorption spectrometry: a comparison of the performance of line source and continuum source instrumentation, Spectrochim. Acta, Part B, 2009, 64, 520–529 CrossRef.
- K. Leopold, M. Foulkes and P. J. Worsfold, Gold-coated silica as a preconcentration phase for the determination of total dissolved mercury in natural waters using atomic fluorescence spectrometry, Anal. Chem., 2009, 81, 3421–3428 CrossRef CAS PubMed.
- R. Ito, M. Kawaguchi, N. Sakui, N. Okanouchi, K. Saito, Y. Seto and H. Nakazawa, Stir bar sorptive extraction with in situ derivatization and thermal desorption-gas chromatography-mass spectrometry for trace analysis of methylmercury and mercury(II) in water sample, Talanta, 2009, 77, 1295–1298 CrossRef CAS PubMed.
- X. Xue, F. Wang and X. Liu, One-step, room temperature, colorimetric detection of mercury (Hg2+) using DNA/nanoparticle conjugates, J. Am. Chem. Soc., 2008, 130, 3244–3245 CrossRef CAS PubMed.
- M. J. Yuan, Y. L. Li and C. H. Li, A colorimetric and fluorometric dual-modal assay for mercury ion by a molecule, Org. Lett., 2007, 9(12), 2313–2316 CrossRef CAS PubMed.
- M. Hollenstein, C. Hipolito, C. Lam, D. Dietrich and D. M. Perrin, A highly selective DNAzyme sensor for mercuric ions, Angew. Chem., Int. Ed., 2008, 47(23), 4346–4350 CrossRef CAS PubMed.
- D. Li, A. Wieckowska and I. Willner, Optical analysis of Hg2+ ions by oligonucleotide–gold nanoparticle hybrids and DNA based machines, Angew. Chem., Int. Ed., 2008, 47, 3927–3931 CrossRef CAS PubMed.
- K. Kneipp, Y. Wang, H. Kneipp, L. T. Perelman, I. Itzkan, R. R. Dasari and M. S. Feld, Single molecule detection using surface-enhanced Raman scattering (SERS), Phys. Rev. Lett., 1997, 78, 1667–1670 CrossRef CAS.
- S. Nie and S. R. Emory, Probing single molecules and single nanoparticles by surface-enhanced Raman scattering, Science, 1997, 275, 1102–1106 CrossRef CAS PubMed.
- Y. Chen, L. H. Wu and Y. H. Chen, Determination of mercury(II) by surface-enhanced Raman scattering spectroscopy based on thiol-functionalized silver nanoparticles, Microchim. Acta, 2012, 177, 341–348 CrossRef CAS.
- G. Wang, C. Lim, L. Chen and J. Hong, Surface-enhanced Raman scattering in nanoliter droplets: towards high sensitivity detection of mercury(II) ions, Anal. Bioanal. Chem., 2009, 394, 1827–1832 CrossRef CAS PubMed.
- W. Ren, C. Z. Zhu and E. K. Wang, Enhanced sensitivity of a direct SERS technique for Hg2+ detection based on the investigation of the interaction between silver nanoparticles and mercury ions, Nanoscale, 2012, 4, 5902–5909 RSC.
- L. Zhang, H. Chang, A. Hirata, H. Wu, Q. K. Xue and M. Chen, Nanoporous gold based optical sensor for sub-ppt detection of mercury ions, ACS Nano, 2013, 7(5), 4595–4600 CrossRef CAS PubMed.
- Z. H. Luo, K. Chen, D. L. Lu, H. Y. Han and M. Q. Zou, Synthesis of paminothioph enol-embedded gold/silver core–shell nanostructures as novel SERS tags for biosensing applications, Microchim. Acta, 2011, 173, 149–156 CrossRef CAS.
- J. Du, L. Jiang, Q. Shao, X. Liu, R. S. Marks, J. Ma and X. Chen, Colorimetric detection of mercury ions based on plasmonic nano-particles, Small, 2013, 9, 1467–1481 CrossRef CAS PubMed.
- S. Lee, S. Kim, J. Choo, S. Y. Shin and Y. H. Lee, Biological imaging of HEK293 cells expressing PLCγ1 using surface-enhanced Raman microscopy, Anal. Chem., 2007, 79, 916–922 CrossRef CAS PubMed.
- D. Li, D. W. Li, J. S. Fossey and Y. T. Long, Cyclic electroplating and stripping of silver on Au@SiO2 core/shell nanoparticles for sensitive and recyclable substrate of surface-enhanced Raman scattering, J. Mater. Chem., 2010, 20, 3688–3693 RSC.
- B. N. R. Jana and T. Pal, Anisotropic metal nanoparticles for use as surface-enhanced Raman substrates, Adv. Mater., 2007, 19, 1761–1765 CrossRef.
- D. Fischer, W. R. Caseri and G. Hahner, Orientation and electronic structure of ion exchanged dye molecules on mica: an X-ray absorption study, J. Colloid Interface Sci., 1998, 198(2), 337 CrossRef CAS.
- Y. Bhattacharjee and A. Chakraborty, Label-free cysteamine-capped silver nanoparticle-based colorimetric assay for Hg(II) detection in water with subnanomolar exactitude, ACS Sustainable Chem. Eng., 2014, 2, 2149–2154 CrossRef CAS.
- Office of Water, Mercury Update: Impact of Fish Advisories, EPA Fact Sheet EPA-823-F-01-011, EPA, Office of Water, Washington, DC, 2001 Search PubMed.
- J. Liu, R. Che, H. Chen, F. Zhang, F. Xia, Q. Wu and M. Wang, Microwave absorption enhancement of multifunctional composite microspheres with spinel Fe3O4 cores and anatase TiO2 shells, Small, 2012, 8, 1214–1221 CrossRef CAS PubMed.
- Y. Wang, K. Wang, B. Zou, T. Gao, X. Zhang, Z. Du and S. Zhou, Magnetic-based silver composite microspheres with nanosheet-assembled shell for effective SERS substrate, J. Mater. Chem. C, 2013, 1, 2441 RSC.
- Y. Fang, S. Guo, C. Zhu, Y. Zhai and E. Wang, Self-assembly of cationic poly electrolyte-functionalized graphene nanosheets and gold nanoparticles: a two-dimensional heterostructure for hydrogen peroxide sensing, Langmuir, 2010, 26, 11277–11282 CrossRef CAS PubMed.
- K. Lai, Y. Zhang, R. Du, F. Zhai, B. A. Rasco and Y. Huang, Determination of chloramphenicol and crystal violet with surface enhanced Raman spectroscopy, Sens. Instrum. Food Qual. Saf., 2011, 5, 19–24 CrossRef.
- N. P. Pieczonka and R. F. Aroca, Single molecule analysis by surfaced enhanced Raman scattering, Chem. Soc. Rev., 2008, 37, 946–954 RSC.
- E. Z. Tan, P. G. Yin, T. T. You, H. Wang and L. Guo, Three dimensional design of large-scale TiO2 nanorods scaffold decorated by silver nanoparticles as SERS sensor for ultrasensitive malachite green detection, ACS Appl. Mater. Interfaces, 2012, 4, 3432–3437 CAS.
- E. Sumesh, M. S. Bootharaju and A. T. Pradeep, A practical silver nanoparticle-based adsorbent for the removal of Hg2+ from water, J. Hazard. Mater., 2011, 189, 450–457 CrossRef CAS PubMed.
- M. M. F. Morel, M. L. A. Kraepiel and M. Amyot, The chemical cycle and bioaccumulation, Annu. Rev. Ecol. Syst., 1998, 29, 543–566 CrossRef.
- H. N. Xie, I. A. Larmour, V. Tileli and D. C. Graham, Deciphering surface enhanced Raman scattering activity of gold nanoworms through optical correlations, J. Phys. Chem. C, 2011, 115, 20515–20522 CAS.
- K. Sivashanmugan, J. D. Liao, B. H. Liu, C. K. Yao and S. C. Luo, Ag nanoclusters on ZnO nanodome array as hybrid SERS-active substrate for trace detection of malachite green, Sens. Actuators, B, 2015, 207, 430–436 CrossRef CAS.
- F. Benz, R. Chikkaraddy, A. Salmon and H. Ohadi, SERS of individual nanoparticles on a mirror: size does matter, but so does shape, J. Phys. Chem. Lett., 2016, 7, 2264–2269 CrossRef CAS PubMed.
- F. P. Yang, Q. Ma, W. Yu and X. G. Su, Naked-eye colorimetric analysis of Hg2+ with bi-color CdTe quantum dots multilayer films, Talanta, 2011, 84, 411–415 CrossRef CAS PubMed.
- P. P. Patra, R. Chikkaraddy, R. P. N. Tripathi, A. Dasgupta and G. V. P. Kumar, Plasmofluidic single-molecule surface-enhanced Raman scattering from dynamic assembly of plasmonic nanoparticles, Nat. Commun., 2014, 5, 4357 CAS.
- L. Wang, T. Li, Y. Du, C. G. Chen and B. L. Li, Au NPs-enhanced surface plasmon resonance for sensitive detection of mercury(II) ions, Biosens. Bioelectron., 2010, 25, 2622–2626 CrossRef CAS PubMed.
- C. V. Hoang, M. Oyama, O. Saito, M. Aono and T. Nagao, Monitoring the presence of ionic mercury in environmental water by plasmon-enhanced infrared spectroscopy, Sci. Rep., 2013, 3, 1175 Search PubMed.
Footnote |
† Electronic supplementary information (ESI) available. See DOI: 10.1039/c6ay02361d |
|
This journal is © The Royal Society of Chemistry 2017 |
Click here to see how this site uses Cookies. View our privacy policy here.