DOI:
10.1039/C6AY02196D
(Paper)
Anal. Methods, 2017,
9, 163-169
Ni doped Ag@C core–shell nanomaterials and their application in electrochemical H2O2 sensing
Received
3rd August 2016
, Accepted 25th November 2016
First published on 28th November 2016
Abstract
In this work, attractive core–shell structured nanomaterials consisting of Ni doped Ag@C were synthesized. Further, a hydrogen peroxide (H2O2) sensor was fabricated by modifying the Ni doped Ag@C (Ni/Ag@C) nanocomposites onto the surface of a glassy carbon electrode (GCE). The composition and morphology of the Ni/Ag@C nanocomposites were characterized by scanning electron microscopy, transmission electron microscopy, energy-dispersive X-ray spectroscopy and Fourier transform infrared spectroscopy. The results indicated that the nanocomposites were synthesized successfully and showed similar grain sizes and favorable dispersity. The electrochemical and electrocatalytic properties of the Ni/Ag@C nanocomposites were studied by cyclic voltammetry. An electrochemical investigation showed that the Ni/Ag@C nanocomposite modified GCE exhibited a good electrocatalytic ability for H2O2 reduction and it was used for the determination of H2O2. The linear range for H2O2 determination was from 0.03 mM to 17.0 mM with a detection limit of 0.01 mM (S/N = 3). The sensitivity was 22.94 μA mM−1 cm−2. It is expected that the application of the Ni/Ag@C nanocomposites could be extended to the construction of other sensors and applications in various sensing fields.
1. Introduction
With such a wide application, many methods have been established for the detection of hydrogen peroxide (H2O2), such as chromatography,1 chemiluminescence,2 spectrophotometry,3 and electrochemistry.4,5 The electrochemical method, including enzyme-based biosensors and non-enzymatic sensors, was used widely thanks to its high selectivity and fast response.6–9 Because of the complicated immobilization procedure and instability of enzyme-based biosensors, non-enzymatic sensors have attracted more and more attention.
With the development of nanotechnology, many nanomaterials such as Au NPs,10,11 Ag NPs,12,13 and Pt NPs14,15 were used in H2O2 electrochemical sensors. However, there have only been a few reports about Ni NPs in the application of H2O2 sensing. The aggregation of nanoparticles hinders their extensive application, so highly dispersed metal nanoparticles are important in the fabrication of sensors.16 Nowadays, core–shell nanomaterials are attracting much attention in the fabrication of sensors17,18 because of their multifunctional properties.19 For example, core–shell Ag–Au bimetal nanoparticles exhibited good electrocatalytic ability towards the oxidation of L-methionine.20 Core–shell CdSe@Ag2Se was found to have excellent electrocatalytic ability towards methyldopa with a low detection limit of 0.04 μmol L−1.21 By using the strategy of sacrificial templating, porous Pt nanowire cores with carbon shells (Pt PNW@C), using Te nanowires as a template, were achieved. It was found that the Pt PNW@C hybrids showed a highly sensitive response for H2O2 detection with a sensitivity of 0.35 μA μM−1 cm−2 and a detection limit of 0.1 μM.22 More recently, a novel core–double shell Au@C@Pt nanocomposite was synthesized and used to fabricate an enzyme-free electrochemical sensor for the rapid and sensitive detection of H2O2. Results showed that the sensor displayed two wide linear ranges towards H2O2 detection and a low detection limit of 0.13 μM was obtained.23 Among those core–shell nanostructures, one of the most attractive shell layers was a carbon coating due to its chemical activity and low preparation cost.24 Ag@C core–shell nanomaterials were one of the most used nanomaterials because the synthetic procedure was convenient.25 The high surface to volume ratio of the Ag@C core–shell nanomaterial may alleviate the aggregation of nickel nanoparticles. Furthermore, the good conductivity of the Ag@C core–shell nanomaterial may enhance its application in the fabrication of sensors.
The aims of this work are to synthesize Ag@C core–shell nanomaterials through a one-pot hydrothermal method26 and Ni/Ag@C nanocomposites through a reduction reaction,27 then to fabricate an enzyme-free H2O2 sensor based on the nanocomposites produced with a simple casting method. Finally the sensing properties and the mechanism of the sensor were thoroughly investigated.
2. Experimental
2.1. Materials
Chitosan (CS, MW 5–6 × 105, >90% deacetylation) was obtained from Shanghai Yuanju Biotechnology Co., Ltd (Shanghai, China). H2O2 (30%, v/v aqueous solution) was obtained from Tianjin Tianli Chemistry Reagent Co., Ltd (Tianjin, China). Glucose and AgNO3 were purchased from Aladdin Reagent Co. Ltd. (Shanghai, China). Other chemicals and reagents were of analytical reagent grade. Doubly distilled water was used throughout the whole experimental process.
2.2. Apparatus and electrochemical measurements
Transmission electron microscope (TEM) images were obtained on a Tecnai G2 F20 S-TWIN (FEI, USA). Fourier transform infrared spectra (FTIR) were observed using a TENSIR 27 (Bruker, Germany). Scanning electron microscopy (SEM) and energy-dispersive X-ray spectroscopy (EDX) images were acquired using a JSM-6700F (JEOL, Japan) and measured at an operating voltage of 10 kV. X-ray powder diffraction (XRD) data were obtained using Cu Kα radiation at a light tube power of 2.2 kW on a Bruker D8 Advance (Bruker AXS, Germany) measurement system.
The electrochemical measurements were carried out using a CHI 660 electrochemical workstation (Shanghai CH Instrument Co., Ltd., China) with a three-electrode electroanalysis system. The working electrode was a glassy carbon electrode (GC electrode) or a Ni/Ag@C nanocomposite modified glassy carbon electrode (Ni/Ag@C/GCE); the counter electrode and reference electrode were a platinum wire and a saturated calomel electrode (SCE), respectively. All electrochemical experiments were performed at room temperature (25 ± 2 °C).
2.3. Preparation of the sensor
2.3.1 Synthesis of the Ag@C core–shell nanomaterials.
Glucose (3.5 g) was dissolved in doubly distilled water (20 mL). Subsequently, 0.03 M of a AgNO3 aqueous solution (2.0 mL) was added into the above-mentioned solution drop-by-drop under vigorous stirring. After stirring for another 15 min, the mixture was transferred into a Teflon-lined autoclave and heated at 180 °C for 4 h. After the reaction, the autoclave was naturally cooled to room temperature. The precipitate was collected by centrifugation, washed with doubly distilled water, and dried at room temperature (25 ± 2 °C).
2.3.2 Synthesis of the Ni/Ag@C nanocomposites.
Ag@C powders (10 mg) were dispersed in ethylene glycol (20 mL) by a hyperacoustic method. 1.0 M NiCl2·6H2O solution (0.1 mL), N2H4·H2O (0.5 mL) and 1.0 M NaOH solution (1.0 mL) were added into the above-mentioned solution sequentially under magnetic stirring conditions. Then the mixture was heated in an oil bath at 60 °C for 1 h. The precipitate was separated by centrifugation, washed with ethanol several times, and dried at room temperature (25 ± 2 °C).
2.3.3 Electrode modification.
Before modification, the GC electrode was polished with 1.0 and 0.3 μm alumina powder sequentially, and rinsed with doubly distilled water, followed by sonication in an ethanol solution and doubly distilled water successively. The GC electrode was dried in a stream of nitrogen. The Ni/Ag@C nanocomposites (1.0 mg) were dispersed into chitosan (1.0 mL, 0.5%) and sonicated for about 15 minutes to form a homogeneous suspension. Then the suspension was cast onto the GC electrode and dried at room temperature in air. The modified electrode can be expressed as a Ni/Ag@C/GC electrode (Scheme 1).
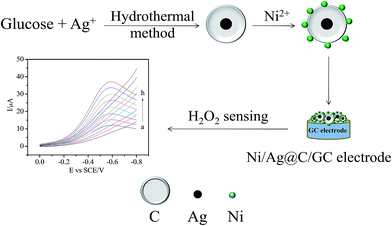 |
| Scheme 1 Schematic illustration of the preparation of Ni/Ag@C and its H2O2 sensing. | |
3. Results and discussion
3.1. Characterization of the Ni/Ag@C nanocomposites
As shown in Fig. 1, the structures and morphologies of Ag@C and Ni/Ag@C were characterized by SEM and TEM. The Ag@C nanocomposites were spherical core–shell structures with an external diameter of about 800 nm (Fig. 1A and B), and have Ag cores with diameters of nearly 80 nm. The Ag core appeared lighter in the centre of the Ag@C nanocomposites in the SEM images (Fig. 1A) while appearing dark in the TEM images (Fig. 1B). From Fig. 1C–E, we can see that the granular nanonickel was attached onto the surface of the Ag@C nanocomposites. In addition, the EDS patterns showed that the nanocomposites were composed of C, Ag, and Ni elements (Fig. 1F), indicating that the Ag@C nanocomposites were synthesized successfully.
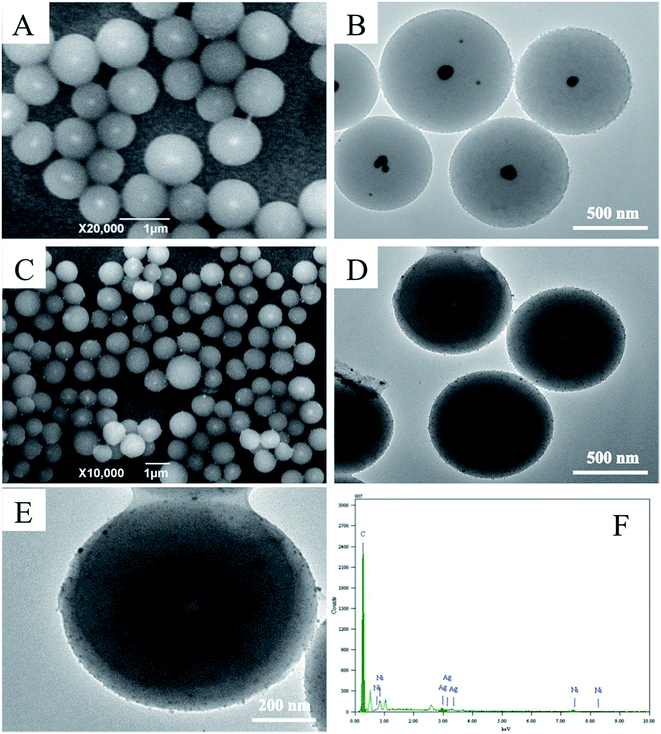 |
| Fig. 1 SEM images of (A) Ag@C and (C) Ni/Ag@C. TEM images of (B) Ag@C and (D) Ni/Ag@C. A TEM image (E) and an EDS spectrum (F) of a single Ni/Ag@C nanocomposite. | |
The FTIR spectra of the Ag@C and Ni/Ag@C are shown in Fig. 2. As we can see from curve a, the characteristic peaks at about 1700 and 1620 cm−1 are the result of C
O and C
C vibrations, respectively of the aromatization of glucose during hydrothermal treatment. The absorption band ranging from 1000 cm−1 to 1300 cm−1 is attributed to C–OH stretching and –OH bending vibrations, which indicates the existence of large numbers of residual hydroxyl groups.26 After loading of the Ni NPs (curve b), the absorption peak intensity of the functional groups becomes lower, which may be due to the absorption peak of the Ni NPs covering the absorption peak of Ag@C.
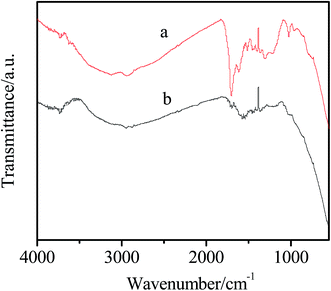 |
| Fig. 2 FTIR spectra of (a) Ag@C and (b) Ni/Ag@C nanocomposites. | |
3.2. Electrochemical properties of Ni/Ag@C
The electrochemical behavior of the Ni/Ag@C nanocomposites was investigated by cyclic voltammetry (CV). As can be seen from Fig. 3, in N2-saturated 0.1 M PBS (pH 7.4), the bare GC, Ag@C/GC and Ni/Ag@C/GC electrodes (curve a, e, and c, respectively) showed no electrochemical response in the absence of H2O2. However, after the addition of 5.0 mM H2O2, the electrochemical response of the different electrodes increased by different degrees. Compared with the bare GC electrode (curve b) and the Ag@C/GC electrode (curve f), the Ni/Ag@C/GC electrode (curve d) showed a remarkable catalytic current peak about 37 μA in intensity at nearly −0.58 V. The results indicate that Ni NP doped Ag@C core–shell nanomaterials exhibit a favorable catalytic performance for the reduction of H2O2.
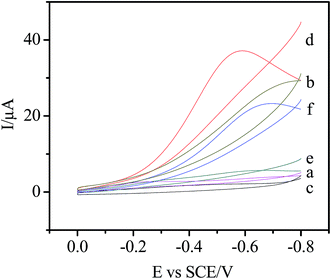 |
| Fig. 3 CVs of (a and b) the bare GC electrode, (e and f) the Ag@C modified GC electrode and (c and d) the Ni/Ag@C modified GC electrode in N2-saturated 0.1 M PBS (pH 7.4) in the absence (a, e and c) and presence (b, f and d) of 5.0 mM H2O2 at a scan rate of 100 mV s−1. | |
As seen in Fig. 4, the catalytic activity of the Ni/Ag@C nanocomposites was studied by changing the concentration of H2O2. In N2-saturated 0.1 M PBS (pH 7.4), the reduction current increased gradually with the increasing injection of H2O2 into the electrolyte, indicating that the Ni/Ag@C nanocomposites had favorable electrocatalytic activity towards H2O2. With an increase in concentration of H2O2, the reduction current increased gradually. As can be seen from Fig. 5, with the increase in scan rate from 20 to 140 mV s−1, the cathodic peak current increased. The peak current increased in a linear relation with the square root of the scan rate between 20 and 200 mV s−1 (inset of Fig. 5), indicating that the process was diffusion-controlled.
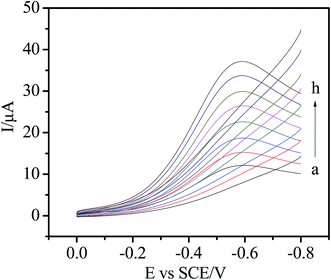 |
| Fig. 4 CVs obtained by the Ni/Ag@C modified GC electrode in N2-saturated 0.1 M PBS (pH 7.4) in the presence of different concentrations of H2O2 (from a to h: 1.5, 2.0, 2.5, 3.0, 3.5, 4.0, 4.5 and 5.0 mM) at a scan rate of 100 mV s−1. | |
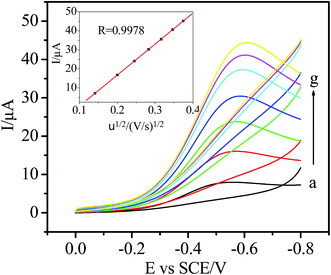 |
| Fig. 5 CVs obtained by the Ni/Ag@C modified GC electrode in N2-saturated 0.1 M PBS (pH 7.4) in the presence of H2O2 (5.0 mM) at different scan rates (from a to g: 20, 40, 60, 80, 100, 120 and 140 mV s−1). Inset: a plot of the electrocatalytic peak current of H2O2versus v1/2. | |
The effect of applied potential on the steady state current of the Ni/Ag@C nanocomposite modified GC electrode was investigated. The results showed that the best electrocatalytic response for H2O2 sensing could be observed at −0.2 V. Although the modified electrode exhibited its highest catalytic activity at about −0.6 V, the background current was high. Meanwhile at −0.2 V, a lower background current, a good signal-to-noise ratio and less interference from other electroactive species could be achieved. Moreover, the influence of oxygen reduction can be limited. Thus, −0.2 V was selected as the working potential for amperometric H2O2 detection. Fig. 6A displays the current response of the Ni/Ag@C nanocomposite modified GC electrode in N2-saturated 0.1 M PBS (pH 7.4) with different concentrations of H2O2 at a working potential of −0.2 V. The Ni/Ag@C nanocomposite modified GC electrode showed an amperometric current response towards H2O2 in less than 3 s, indicating that the response was fast.28–30 The calibration curve of the H2O2 sensor is shown in Fig. 6B. The linear regression equation was found to be Ip (μA) = 0.1812 + 0.7202C (mM) with a correlation coefficient of 0.9979 in the concentration range of 0.03–17.0 mM. The sensitivity of this sensor was 22.94 μA mM−1 cm−2, which was higher than some H2O2 sensors.31–33 The detection limit was 0.01 mM at a signal-to-noise ratio of 3. Table 1 summarizes a comparison of the analytical performances of different kinds of nanomaterials used for H2O2 sensing. It can be seen that our proposed sensor displayed a good performance with a higher applied potential, wider linear range and lower detection limit.
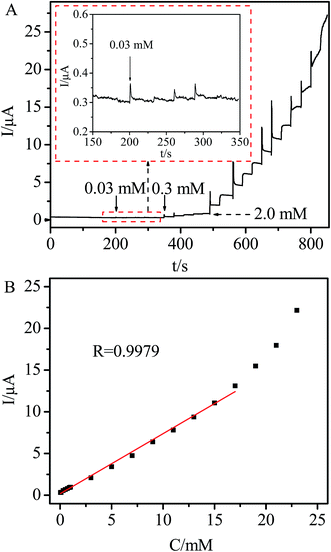 |
| Fig. 6 (A) Current response of the Ni/Ag@C modified GC electrode in stirred N2-saturated 0.1 M PBS (pH 7.4) with different concentrations of H2O2 at a working potential of −0.2 V. Inset: an amplification of the current versus time variation curve for low concentrations. (B) Calibration curve of H2O2versus its concentration. | |
Table 1 Comparison of the analytical performance of H2O2 sensors based on different nanomaterials
Nanomaterials |
Applied potential (V) |
Linear range (mM) |
Detection limit (mM) |
Ref. |
Poly[(2-ethyldimethylammonioethyl methacrylate ethyl sulfate)-co-(1-vinylpyrrolidone)] functionalized silver nanoparticles.
Ag nanoparticle decorated nanofibers.
Not available.
|
PQ11–Aga |
−0.3 |
0.1–180.0 |
0.034 |
34
|
Pd hollow nanocube |
−0.4 |
0.1–24.0 |
0.0074 |
35
|
Ag NPs-NFsb |
−0.3 |
0.1–80.0 |
0.062 |
36
|
Ag@C@Ag |
NAc |
0.07–10.0 |
0.023 |
37
|
Au@C@Pt |
0.0 |
0.009–1.86 |
0.0013 |
38
|
1.86–7.11 |
Fe3O4@C–Cu |
−0.3 |
0.08–372.0 |
0.033 |
39
|
Ni/Ag@C |
−0.2 |
0.03–17.0 |
0.01 |
This work |
3.3. Interference study
In order to investigate the anti-interference and selectivity of the sensor, the interference effects were investigated. As shown in Fig. 7, a H2O2 solution (2.0 mM) was firstly added into the N2-saturated 0.1 M PBS (pH 7.4) at a working potential of −0.2 V, then the interferents (1.0 mM, respectively) were added. There was an obvious amperometric response to the injection of H2O2, while no obvious response appeared when ascorbic acid (AA), glucose (Glu) and ethanol were injected. The results indicate that the sensor exhibits a good anti-interference ability and selectivity for the detection of H2O2.
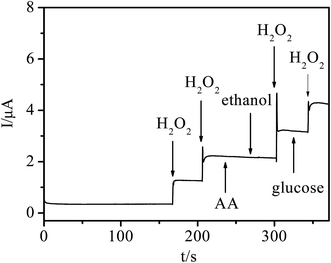 |
| Fig. 7 Amperometric response of the Ni/Ag@C modified GC electrode after adding 2.0 mM H2O2, 1.0 mM ascorbic acid (AA), glucose (Glu) and ethanol. | |
3.4. Repeatability and stability
The repeatability and stability of the sensor were evaluated. The amperometric current responses of five modified electrodes were investigated at −0.3 V towards 2.0 mM H2O2. The relative standard deviation (RSD) was less than 4%, indicating that the performance of the Ni/Ag@C nanocomposite modified GC electrode was reproducible. After three weeks, the modified electrode retained 90% of its initial current response towards 5.0 mM H2O2. Thus, this sensor exhibited acceptable repeatability and stability.
4. Conclusions
In conclusion, Ni/Ag@C nanocomposites were synthesized and an enzyme-free hydrogen peroxide sensor based on the nanocomposites was fabricated successfully. The synthesized nanocomposites showed similar grain sizes and favorable dispersity. The sensor exhibited favorable electrocatalytic properties towards H2O2, including high sensitivity and a fast response, as well as a good anti-interference ability. So this study may provide a possible method to develop new electrochemical sensors.
Acknowledgements
The authors gratefully acknowledge the financial support of this project by the National Science Fund of China (No. 21275116, 21575113), the Specialized Research Fund for the Doctoral Program of Higher Education (No. 20126101110013), the Natural Science Fund of Shaanxi Province in China (No. 2013KJXX-25), and the Scientific Research Foundation of Shaanxi Provincial Key Laboratory (14JS094, 15JS100 and 16JS099).
References
- U. Pinkernell, S. Effkemann and U. Karst, Simultaneous HPLC Determination of Peroxyacetic Acid and Hydrogen Peroxide, Anal. Chem., 1997, 69, 3623 CrossRef CAS PubMed.
- W. Lei and Z. H. Lin, Detection of Hydrogen Peroxide in River Water via a Microplate Luminescence Assay with Time-Resolved (“Gated”) Detection, Microchim. Acta, 2003, 143, 269 CrossRef CAS.
- Q. Chang, K. Deng, L. Zhu, G. Jiang, C. Yu and H. Tang, Determination of hydrogen peroxide with the aid of peroxidase-like Fe3O4 magnetic nanoparticles as the catalyst, Microchim. Acta, 2009, 165, 299 CrossRef CAS.
- S. J. He, B. Y. Zhang, M. M. Liu and W. Chen, Non-enzymatic hydrogen peroxide electrochemical sensor based on a three-dimensional MnO2 nanosheets/carbon foam composite, RSC Adv., 2014, 4, 49315 RSC.
- J. X. Zhang, L. P. Tu, S. Zhao, G. H. Liu, Y. Y. Wang, Y. Wang and Z. Yue, Fluorescent gold nanoclusters based photoelectrochemical sensors for detection of H2O2 and glucose, Biosens. Bioelectron., 2015, 67, 296 CrossRef CAS PubMed.
- K. M. Fang, Y. Y. Yang, L. Y. Fu, H. T. Zheng, J. H. Yuan and L. Niu, Highly selective H2O2 sensor based on 1-D nanoporous Pt@C hybrids with core–shell structure, Sens. Actuators, B, 2014, 191, 401 CrossRef CAS.
- H. Yue, J. He, D. Xiao and M. F. Choi, Biosensor for determination of hydrogen peroxide based on Yucca filamentosa membrane, Anal. Methods, 2013, 5, 5437 RSC.
- E. Kurowska, A. Brzózka, M. Jarosz, G. D. Sulka and M. Jaskuła, Silver nanowire array sensor for sensitive and rapid detection of H2O2, Electrochim. Acta, 2013, 104, 439 CrossRef CAS.
- S. L. Yang, G. Li, G. F. Wang, J. H. Zhao, M. F. Hu and L. B. Qu, A novel nonenzymatic H2O2 sensor based on cobalt hexacyanoferrate nanoparticles and graphene composite modified electrode, Sens. Actuators, B, 2015, 208, 593 CrossRef CAS.
- P. J. Ni, Y. Zhang, Y. J. Sun, Y. Shi, H. C. Dai, J. T. Hu and Z. Li, Facile synthesis of Prussian blue@gold nanocomposite for nonenzymatic detection of hydrogen peroxide, RSC Adv., 2013, 3, 15987 RSC.
- H. S. Li, Y. J. Li and S. Q. Wang, Water-soluble Au nanocages for enzyme-free H2O2 sensor and 4-nitrophenol reduction, CrystEngComm, 2015, 17, 2368 RSC.
- J. S. Easow and T. Selvaraju, Unzipped catalytic activity of copper in realizing bimetallic Ag@Cu nanowires as a better amperometric H2O2 sensor, Electrochim. Acta, 2013, 112, 648 CrossRef CAS.
- P. M. Nia, F. Lorestani, W. P. Meng and Y. Alias, A novel non-enzymatic H2O2 sensor based on polypyrrole nanofibers–silver nanoparticles decorated reduced graphene oxide nano composites, Appl. Surf. Sci., 2015, 332, 648 CrossRef.
- J. Zhang, J. Li, F. Yang, B. L. Zhang and X. R. Yang, Preparation of Prussian blue@Pt nanoparticles/carbon nanotubes composite material for efficient determination of H2O2, Sens. Actuators, B, 2009, 143, 373 CrossRef.
- X. H. Niu, C. Chen, H. L. Zhao, Y. Chai and M. B. Lan, Novel snowflake-like Pt–Pd bimetallic clusters on screen-printed gold nanofilm electrode for H2O2 and glucose sensing, Biosens. Bioelectron., 2012, 36, 262 CrossRef CAS PubMed.
- C. Y. Liu and J. M. Hu, Hydrogen peroxide biosensor based on the direct electrochemistry of myoglobin immobilized on silver nanoparticles doped carbon nanotubes film, Biosens. Bioelectron., 2009, 24, 2149 CrossRef CAS PubMed.
- Z. Z. Cui, H. Y. Yin and Q. L. Nie, Controllable preparation of hierarchically core–shell structure NiO/C microspheres for non-enzymatic glucose sensor, J. Alloys Compd., 2015, 632, 402 CrossRef CAS.
- J. Q. Tian, Y. L. Luo, H. L. Li, W. B. Lu, G. H. Chang, X. Y. Qin and X. P. Sun, Ag@poly(m-phenylenediamine)–Ag core–shell nanoparticles: one-step preparation, characterization, and their application for H2O2 detection, Catal. Sci. Technol., 2011, 1, 1393 CAS.
- X. Zhou, X. X. Dai, J. G. Li, Y. M. Long, W. F. Li and Y. F. Tu, A sensitive glucose biosensor based on Ag@C core–shell matrix, Mater. Sci. Eng., C, 2015, 49, 579 CrossRef CAS PubMed.
- M. Murugavelu and B. Karthikeyan, Synthesis, characterization of Ag–Au core–shell bimetal nanoparticles and its application for electrocatalytic oxidation/sensing of L-methionine, Mater. Sci. Eng., C, 2017, 70, 656 CrossRef CAS PubMed.
- K. Asadpour-Zeynali and F. Mollarasouli, A novel and facile synthesis of TGA-capped CdSe@Ag2Se core–shell quantum dots as a new substrate for high sensitive and selective methyldopa sensor, Sens. Actuators, B, 2016, 237, 387 CrossRef CAS.
- K. M. Fang, Y. Y. Yang, L. Y. Fu, H. T. Zheng, J. H. Yuan and L. Niu, Highly selective H2O2 sensor based on 1-D nanoporous Pt@C hybrids with core–shell structure, Sens. Actuators, B, 2014, 191, 401 CrossRef CAS.
- Y. Y. Zhang, Y. H. Li, Y. Y. Jiang, Y. C. Li and S. Q. Li, The synthesis of Au@C@Pt core–double shell nanocomposite and its application in enzyme-free hydrogen peroxide sensing, Appl. Surf. Sci., 2016, 378, 375 CrossRef CAS.
- J. X. Zhu, T. Sun, H. H. Hung, J. Ma, F. Y. C. Boey, X. W. Lou, H. Zhang, C. Xue, H. Y. Chen and Q. Y. Yan, Fabrication of Core–Shell Structure of M@C (M = Se, Au, Ag2Se) and Transformation to Yolk–Shell Structure by Electron Beam Irradiation or Vacuum Annealing, Chem. Mater., 2009, 21, 3848 CrossRef CAS.
- X. M. Sun and Y. D. Li, Ag@C core/shell structured nanoparticles: controlled synthesis, characterization, and assembly, Langmuir, 2005, 21, 6019 CrossRef CAS PubMed.
- S. X. Mao, W. F. Li, Y. M. Long, Y. F. Tu and A. P. Deng, Sensitive electrochemical sensor of tryptophan based on Ag@C core–shell nanocomposite modified glassy carbon electrode, Anal. Chim. Acta, 2012, 738, 35 CrossRef CAS PubMed.
- J. W. Huang, Y. Q. He, J. Jin, Y. R. Li, Z. P. Dong and R. Li, A novel glucose sensor based on MoS2 nanosheet functionalized with Ni nanoparticles, Electrochim. Acta, 2014, 136, 41 CrossRef CAS.
- W. Zhao, H. C. Wang and X. Qin, A novel nonenzymatic hydrogen peroxide sensor based on multi-wall carbon nanotube/silver nanoparticle nanohybrids modified gold electrode, Talanta, 2009, 80, 1029 CrossRef CAS PubMed.
- Y. D. Zhao, Y. H. Bi and W. D. Zhang, The interface behavior of hemoglobin at carbon nanotube and the detection for H2O2, Talanta, 2005, 65, 489 CrossRef CAS PubMed.
- W. Zheng, J. Li and Y. F. Zheng, An amperometric biosensor based on hemoglobin immobilized in poly(ε-caprolactone) film and its application, Biosens. Bioelectron., 2008, 23, 1562 CrossRef CAS PubMed.
- S. Abdollah, M. Monierosadat and N. Abdollah, A novel non-enzymatic hydrogen peroxide sensor based on single walled carbon nanotubes–manganese complex modified glassy carbon electrode, Electrochim. Acta, 2011, 56, 3387 CrossRef.
- H. Heli, N. Sattarahmady, R. Dehdari Vais and A. R. Mehdizadeh, Enhanced electrocatalytic reduction and highly sensitive nonenzymatic detection of hydrogen peroxide using platinum hierarchical nanoflowers, Sens. Actuators, B, 2014, 192, 310 CrossRef CAS.
- S. Zhang, Q. L. Sheng and J. B. Zheng, Synthesis of Ag–HNTs–MnO2 nanocomposites and their application for nonenzymatic hydrogen peroxide electrochemical sensing, RSC Adv., 2015, 5, 26878 RSC.
- W. B. Lu, F. Liao, Y. L. Luo, G. H. Chang and X. P. Sun, Hydrothermal synthesis of well-stable silver nanoparticles and their application for enzymeless hydrogen peroxide detection, Electrochim. Acta, 2011, 56, 2295 CrossRef CAS.
- G. D. Nie, X. F. Lu, J. Y. Lei, L. Yang, X. J. Bian, Y. Tong and C. Wang, Sacrificial template-assisted fabrication of palladium hollow nanocubes and their application in electrochemical detection toward hydrogen peroxide, Electrochim. Acta, 2013, 99, 145 CrossRef CAS.
- J. Q. Tian, S. Liu and X. P. Sun, Supra molecular micro fibrils of o-phenylenediamine dimers: oxidation-induced morphology change and the spontaneous formation of Ag nanoparticle decorated nanofibers, Langmuir, 2010, 26, 15112 CrossRef CAS PubMed.
- Q. M. Wang, H. L. Niu, C. J. Mao, J. M. Song and S. Y. Zhang, Facile synthesis of trilaminar core–shell Ag@C@Ag nanospheres and their application for H2O2 detection, Electrochim. Acta, 2014, 127, 349 CrossRef CAS.
- Y. Y. Zhang, Y. H. Li, Y. Y. Jiang, Y. C. Li and S. X. Li, The synthesis of Au@C@Pt core–double shell nanocomposite and its application in enzyme-free hydrogen peroxide sensing, Appl. Surf. Sci., 2016, 378, 375 CrossRef CAS.
- M. Y. Zhang, Q. L. Sheng, F. Nie and J. B. Zheng, Synthesis of Cu nanoparticles-loaded Fe3O4@carbon core–shell nanocomposite and its application for electrochemical sensing of hydrogen peroxide, J. Electroanal. Chem., 2014, 730, 10 CrossRef CAS.
|
This journal is © The Royal Society of Chemistry 2017 |
Click here to see how this site uses Cookies. View our privacy policy here.