DOI:
10.1039/C6SM00868B
(Paper)
Soft Matter, 2016,
12, 7364-7371
cDICE method produces giant lipid vesicles under physiological conditions of charged lipids and ionic solutions
Received
12th April 2016
, Accepted 1st August 2016
First published on 11th August 2016
Abstract
Giant unilamellar vesicles are a powerful and common tool employed in biophysical studies of lipid membranes. Here we evaluate a recently introduced method of vesicle formation, “continuous droplet interface crossing encapsulation” (cDICE). This method produces monodisperse giant unilamellar vesicles of controlled sizes and high encapsulation efficiencies, using readily available instrumentation. We find that mixtures of phospholipids within vesicle membranes produced by cDICE undergo phase separation at the same characteristic temperatures as lipids in vesicles formed by a complementary technique. We find that the cDICE method is effective both when vesicles are produced from charged lipids and when the surrounding buffer contains a high concentration of salt. A shortcoming of the technique is that cholesterol is not substantially incorporated into vesicle membranes.
Introduction
Quantitative, well-controlled biophysical studies that employ lipid membranes1–6 rely on the ability of researchers to create model vesicles de novo with known compositions. Giant unilamellar vesicles (GUVs), with diameters greater than 10 μm, are powerful model systems because they can be readily imaged by optical microscopy.7 Limitations within some techniques of fabricating GUVs render important regions of parameter space challenging to achieve.7–9 Here we evaluate a recently introduced method of vesicle formation called “continuous droplet interface crossing encapsulation” (cDICE, Fig. 1). This method produces monodisperse vesicles of controlled size and high encapsulation efficiency, using readily available instrumentation. One current application of the cDICE method is to produce deformable films of nematic microtubule assemblies inside vesicles in order to investigate the dynamics of defects within those nematics.10 The cDICE method has been previously shown to be successful in incorporating zwitterionic phospholipids into vesicle membranes.11 Here, we test whether cDICE is equally successful in producing GUVs under two other important sets of conditions: incorporation of high fractions of charged lipids within vesicle membranes and incorporation of high ionic strength buffers within surrounding solutions. These conditions can be difficult to achieve using alternate methods.
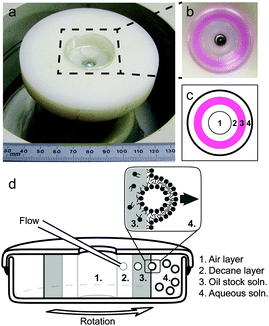 |
| Fig. 1 Apparatus and schematics of the cDICE method of producing vesicles. (a) A homebuilt plastic chuck attached to a bench-top centrifuge holds an empty cDICE chamber consisting of a sealed Petri dish with a circular hole in the top. The ruler shows millimetres. (b) The chamber is filled with layers of solutions of different densities while rotating. (c and d) Top and side schematics: [1] a glass capillary is inserted into a sealed Petri dish that is rotated by a bench top centrifuge. Aqueous solution emerges from the capillary tip. [2] Decane (which has a lower viscosity than the stock oil solution) flows past the tip of the stationary capillary, shearing off droplets of a threshold size on the order of the capillary's inner diameter. [3] As each aqueous droplet travels through a stock solution of lipids in oil, lipids partition into a monolayer at the surface of the aqueous droplet. [4] The outermost layer of the Petri dish contains an aqueous solution. A monolayer of lipids assembles at the interface between this aqueous solution and the stock oil solution. As each droplet passes through this interface, it acquires a second monolayer of lipids, to complete the bilayer membrane. | |
Fabrication of GUVs containing high fractions of charged lipids is of interest because charged lipids are common within biological membranes. Approximately 25% of all phospholipids in mammalian plasma membranes are charged,18,19 and almost all of these charged lipids are located in the cytoplasmic leaflet of the membrane.19,20 Even higher fractions of charged lipids in biological membranes can be found elsewhere, as in mitochondria21 or bacteria.22 Similarly, the use of buffers with high ionic strengths is of interest because saline solutions are widespread in biology. For example, the ionic strength of blood is approximately 0.2 M.23 Moreover, the movement of ions across membranes is itself an important field of research.24
Overview of other fabrication methods
To motivate our evaluation of the cDICE method for producing vesicles, we will briefly discuss four classes of these methods, summarized in Table 1: electroformation, gentle hydration, jetting, and reverse emulsion techniques. These methods are more extensively tabulated in recent reviews.7–9 Researchers who are familiar with these methods may prefer to progress directly to the Experimental section.
Table 1 Summary of whether four techniques for fabricating GUVs are compatible with a variety of preferable experimental conditions
Technique |
Charged lipids |
Ionic buffer |
Oil free |
Mono-disperse |
Ref. |
Electroformation |
Only if low |
Only if low |
Yes |
No |
12
|
Gentle hydration |
Yes |
Some cases |
Yes |
No |
13
|
Jetting |
Yes |
Yes |
No |
Yes |
14–16
|
Reverse emulsion |
Yes |
Yes |
No |
Yes |
7, 8 and 17
|
I. Electroformation.
This technique12 is favored for creating GUVs that contain low concentrations of charged lipids and that are surrounded by a solution containing low concentrations of ions. In these cases, electroformation protocols are straightforward, inexpensive, and produce a high yield of defect-free unilamellar vesicles, of which a significant fraction are >100 μm in diameter.25 Moreover, minimal specialized equipment or training is required. Unfortunately, incorporation of charged lipids into electroformed vesicles drastically lowers the yield.26,27 Electroforming vesicles in the presence of high concentrations of sugars in the surrounding solution partially improves the yield, but leads to artifacts.28 Similarly, electroformation of GUVs in solutions with ionic strengths greater than ∼10 mM can lead to low yields and difficulty in detaching vesicles from substrates of electroformation chambers.29,30 At least some of these problems are mitigated by electroforming at higher field strengths and frequencies,31 but electroformed vesicles of cationic lipids cannot be detached from the substrate.32 An alternate tactic is to introduce ionic solutions only after electroforming vesicles in the presence of sugar solutions.33 This method is subject to the same artifacts cited above.28 Electroformation results in a broad distribution of vesicle sizes. Techniques that have narrowed this distribution have a side effect of limiting vesicle diameters to ≤25 μm.25
II. Gentle hydration.
To address some of the challenges above while fabricating GUVs containing charged lipids, many researchers employ methods of gentle hydration of lipid layers on a solid substrate.13 However, the majority of vesicles grown on bare substrates contain defects. For example, Rodriguez et al. found that when vesicles were made with 40% charged lipid, only 15% of vesicles did not have defects in the form of paucilamellar vesicles, encapsulated vesicles, or tubules.27 The incorporation of salt further degrades GUV quality, and does so in a way that is dependent on the amount of charged lipids present.13,34 Moreover, gentle hydration of lipids on a solid substrate is generally successful only when a significant fraction of lipids are charged,7 rendering control experiments impossible because GUVs with charged lipids cannot be produced by the same technique as those without. Hydrating lipids on a gel layer instead of on a bare substrate solves some of these issues. Hydration on a gel layer results in vesicle formation on time scales as short as minutes and is successful even when no charged lipids are present.32,35 Moreover, the procedure is successful in a phosphate buffered saline solution, with the caveat that the efficiency of vesicle detachment from the substrate is minimal unless sonication is employed.32 One significant concern is that when the gel is made of agarose (as opposed to polyvinyl alcohol or cross-linked agarose), molecules of gel material associate with the vesicle membranes.32,35,36
III. Jetting.
Both electroformation and gentle hydration generally produce GUVs with high polydispersity and with symmetric lipid compositions. One way to produce GUVs with a narrower distribution of diameters is to employ jetting techniques, which produce GUVs via a controlled, localized pulse of fluid across a black lipid membrane.14–16 Qualitative (but not always quantitative) asymmetry in the membrane can be achieved by fusing small vesicles of different lipid compositions to an oily interface spanning an area where the black lipid membrane eventually forms.16 The same technique must be used to deliver cholesterol to the membrane. A disadvantage of the jetting method is that the equipment is highly specialized and that vesicles produced by current jetting protocols can be fragile and have a limited lifetime, often on the order of one hour.
IV. Reverse emulsions.
Finally, reverse emulsion techniques are commonly used in applications requiring high throughput of monodisperse GUVs with asymmetric lipid compositions.7,8,17 Early versions of the technique pioneered the centrifugation of polydisperse reverse emulsions through an oil–water interface.37,38 Droplet polydispersity and lack of control of how droplets cross the interface can lead to poor yield.11 Droplet polydispersity is reduced through the use of microfluidic devices with micron-scale features, which mechanically or hydrodynamically form vesicles one at a time.39–43 These techniques give the user a high degree of control, but they rely on specialized equipment and on significant expertise and institutional infrastructure in order to fabricate and assemble the apparatus, rendering the techniques difficult to implement in many laboratories. Some microfluidic techniques successfully incorporate significant amounts of cholesterol into GUVs, but leave large, visible solvent lenses between the monolayer leaflets of the membrane.40 In principle, all reverse emulsion techniques are compatible with charged lipids and ionic buffers.
To increase the accessibility of reverse emulsion techniques that produce monodisperse GUVs, Abkarian et al. developed the cDICE method,11 depicted schematically in Fig. 1. This setup combines concepts from microfluidics with materials that are readily available. The technique transports droplets across an oil–water interface. All techniques that successfully transport large-diameter droplets within feasible time scales require an external force, such as centrifugation.44 In the cDICE method, horizontal, concentric layers of fluids with different densities are established within a sealed, spinning Petri dish with a circular hole cut in the top. A stationary glass capillary, like those widely available for patch clamp experiments, is inserted into the innermost layer of fluid, which is decane oil. Aqueous solution is pushed through the capillary by a syringe pump or compressed gas. Aqueous droplets that emerge from the capillary are driven by centrifugal force through a second layer of oil (where they acquire their first monolayer of lipids), through an oil–water interface (where they acquire their second monolayer of lipids), and into a water layer (where the resulting vesicles accumulate). This technique has a high yield (∼100 vesicles per second), and successfully incorporates micron-scale objects inside GUVs.11
Here we evaluate the cDICE method's utility for creating vesicles that are broadly applicable for biophysical studies using charged lipids or ionic solutions. First, we demonstrate that the method creates vesicles with known, controllable compositions, the primary requirement for quantitative experiments on membrane behavior. We then show that the technique successfully creates vesicles composed of large fractions of charged lipids and that the technique successfully produces vesicles in buffers with high concentrations of salt. Together, these attributes make the cDICE method highly useful to the community.
Experimental
Materials
1,2-Diphytanoyl-sn-glycero-3-phosphocholine (DiPhyPC); 1,2-dipalmitoyl-sn-glycero-3-phosphocholine (DPPC); 1,2-diphytanoyl-sn-glycero-3-phosphoglycerol (DiPhyPG); and 1,2-dipalmitoyl-sn-glycero-3-phosphoglycerol (DPPG); were obtained from Avanti Polar Lipids (Alabaster, AL). Cholesterol was obtained from Sigma (St. Louis, MO). All lipids were used without further purification and were stored in chloroform at −20 °C until use. The fluorescently labeled lipid Texas Red 1,2-dipalmitoyl-sn-glycero-3-phosphoethanolamine (TR-DPPE, Invitrogen, Eugene, OR) was included at 0.8 mol% to visualize vesicles and to provide contrast between phases. Heavy mineral oil was obtained from Sigma (St. Louis, MO). Phosphate buffered saline (PBS) was obtained from Fisher Scientific (Fair Lawn, NJ), with a formulation of 155 mM NaCl, 2.71 mM Na2HPO4, 1.54 mM KH2PO4, and pH 7.2. To maintain a difference in density between the inside and outside of the vesicle, the inner and outer aqueous solutions contained 100 mM glucose or sucrose, respectively. 18 MΩ cm water was produced by a Barnstead filtration system (Barnstead, MA). All other chemicals were obtained from Sigma (St. Louis, MO).
Preparing stock oil solution
2 μmole of lipids in chloroform were added to a glass scintillation vial and dried at a low pressure of ∼10 mbar in a desiccator for at least 30 minutes. The desiccator was opened in a glove box filled with dry nitrogen. 4 mL of heavy mineral oil was added to the scintillation vial, for a final lipid concentration of 0.5 mM. The vial was then sealed by tightening the lid and wrapping the junction with paraffin film. Lipids were allowed to disperse in the oil for 1 hour at room temperature. The solution was then bath sonicated for 2 hours while temperature was maintained below 40 °C.
Preparing vesicles
Vesicles were prepared by the cDICE method, as depicted in the schematic and photos in Fig. 1. The top and bottom of a 35 mm plastic Petri dish were sealed together with Devon 5 minute epoxy (Danvers, MA), and a 13 mm diameter hole was cut in the center of the top to the Petri dish. This entire Petri dish assembly was attached to the spindle head of a bench top centrifuge in a home-built cylindrical chuck made from a disk of plastic ∼5 cm thick and ∼8 cm in diameter. The spindle rotated at 35 Hz. 1.5 mL of aqueous solution (usually 100 mM glucose) was added to the empty dish to form the outermost fluid layer. Next, 3.5 mL of 0.5 mM lipid stock oil solution were added to form an intermediate fluid layer. Last, 1 mL of decane was added to form the innermost layer. These layers remained separate due to their different densities. A silanized capillary with an inner diameter of ∼15 μm was connected to a reservoir of aqueous solution (usually 100 mM sucrose). Fluid was driven through the capillary by a syringe pump (Harvard Apparatus, Holliston, MA) at 0.05 mL min−1, and the capillary tip was inserted into the decane layer. To ensure that all lipid species were in a well-mixed liquid phase, the entire apparatus was placed inside an oven at least 10 °C above the highest lipid melting temperature in the system. Vesicles were formed over a period of ∼15 min. The centrifuge was then brought to rest, and the vesicles were harvested from the aqueous layer with a Pasteur pipette. Vesicles were imaged within 2 hours of formation.
Microscopy
Vesicles were examined by fluorescence microscopy as in Veatch et al.45 Briefly, vesicle solutions were diluted ∼5
:
1 in an isotonic solution and deposited between two coverslips. The coverslip assembly was sealed with vacuum grease and coupled with thermal paste (Omega Engineering, Stamford, CT) to a stage. Temperature control of the stage was achieved with a Wavelength controller connected to a Peltier device and a thermistor temperature probe with a manufacturer quoted accuracy of 0.02 °C (Wavelength Electronics, Bozeman, MT). Epifluorescence microscopy was performed with a 40× objective on a Nikon microscope with either a Coolsnap HQ or QuantEM charge-coupled device camera (Photometrics, Tucson, AZ). Transition temperatures were recorded as the temperature at which half of the vesicles in a sample had visible domains. The reported uncertainty spans the range of temperatures that begins when 10% of vesicles in a sample have phase separated and ends when 90% have done so.
Adding cholesterol to membranes
Cholesterol was added to membranes with “preloaded” methyl-β-cyclodextrin (mβCD) as in Klein et al.46 Briefly, 5 mg of cholesterol and 55.6 mg of mβCD were vigorously mixed in a solution of 1 mL methanol and 0.225 mL chloroform. This mixture was dried under nitrogen and then under vacuum for 30 minutes. The resulting crystals were rehydrated at a concentration of 11.2 mg mL−1 (1 mg mL−1 of cholesterol). The resulting cholesterol-mβCD solution was titrated into the vesicle solution until coexisting liquid phases were observed within vesicle membranes.
Results
Uncharged phospholipids
To determine whether the final composition of uncharged lipids within vesicle membranes produced by cDICE faithfully reproduces the initial composition of lipids within the stock oil solution, we exploited the fact that lipid membranes undergo gel–liquid phase transitions at temperatures that are characteristic of the different lipid species and the ratio of those species. To achieve a wide range of gel–liquid transition temperatures (Ttrans) by simply changing the ratio of lipids in a binary membrane, we chose one lipid (DiPhyPC) to have a very low main chain transition temperature (Tmelt) and the other (DPPC) to have a high Tmelt, as shown in Table 2. The data in Table 3 show that the transition temperatures, and hence the ratio of lipids incorporated into cDICE vesicles is indistinguishable from the ratio of lipids in electroformed vesicles, at least for the uncharged phospholipids in Table 3.
Table 2 Phospholipids used to produce cDICE vesicles
Common Name |
Structural Name |
Charge |
T
melt (°C) |
Ref. |
DiPhyPC |
di(4Me-16 : 0)-PC |
Zwitterionic |
<−120 |
47
|
DOPC |
di(18 : 1)-PC |
Zwitterionic |
−17 |
48
|
DPPC |
di(16 : 0)-PC |
Zwitterionic |
41 |
48
|
DiPhyPG |
di(4Me-16 : 0)-PG |
Negative |
Likely < 0 |
48
|
DPPG |
di(18 : 1)-PG |
Negative |
41 |
48
|
Table 3 GUVs composed of binary mixtures of DiPhyPC and DPPC lipids exhibit gel domains within a liquid background. The temperature, Ttrans, at which the gel domains melt is indistinguishable between vesicles produced by the cDICE method and by electroformation
mol% |
mol% |
cDICE |
Electroformation |
DiPhyPC |
DPPC |
T
trans (°C) |
T
trans (°C) |
80 |
20 |
20 ± 3 |
21 ± 1 |
70 |
30 |
28 ± 2 |
27 ± 2 |
20 |
80 |
41 ± 3 |
39 ± 2 |
The agreement between the cDICE and electroformation results in Table 3 also implies that the cDICE method successfully avoids entraining large volumes of oil within the bilayer. The existence of the transition itself is sensitive to the presence of oil in the bilayer.40 Although the cDICE method may well entrain small volumes of oil in the bilayer, Table 3 implies that any effects of the oil (if present) on Ttrans, a static physical property, are smaller than typical measurement uncertainties.
Cholesterol
Sterols are commonly incorporated in model membranes in order to approximate the compositions of plasma cell membranes49 and to adjust membrane physical parameters.45,50 One unique characteristic of membranes composed of ternary mixtures of a lipid with a low melting temperature, a lipid with a high melting temperature, and cholesterol (or similar sterol) is their ability to phase separate into coexisting liquid phases.45,50 Without sterols, membranes composed of mixtures of phospholipids demix into coexisting gel and liquid phases as in Table 3. A well-studied model system that exhibits both gel–liquid and liquid–liquid phase separation is the ternary lipid mixture of DOPC, DPPC, and cholesterol. At room temperature, electroformed vesicles with equal amounts of DOPC and DPPC demix into gel domains within a liquid background when their cholesterol mole fractions are less than 10 mol%. The vesicles demix into two liquid phases or into three phases (a solid and two liquids) when their cholesterol mole fractions are above 10 mol%.51
To test if the cDICE technique successfully incorporates cholesterol into vesicles, we prepared our initial cDICE stock oil solution with an excess of cholesterol, with a composition of 1
:
1
:
100 DOPC
:
DPPC
:
cholesterol. For this experiment, the amount of total lipid was increased from 2 μmol to 102 μmol to maintain a constant final concentration of phospholipid. Since the solubility of cholesterol in membranes composed of PC-lipids is ∼66 mol%,52,53 electroformation of this mixture would be expected to produce membranes containing 17
:
17
:
66 DOPC
:
DPPC
:
cholesterol. Surprisingly, the cDICE method incorporated less than 1% of the available cholesterol into vesicle membranes, despite cholesterol's high initial fraction in the stock oil solution. Fig. 2 shows that the resulting cDICE vesicles contain gel phase domains (identified by their noncircular shapes) within the background liquid phase. Therefore, these membranes contain less than 10 mol% cholesterol.
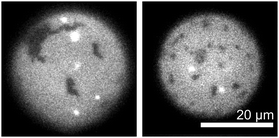 |
| Fig. 2 Two examples of cDICE vesicles produced using a stock oil solution containing 1 : 1 : 100 DOPC : DPPC : Chol. The presence of gel domains (dark regions with static, non-circular shapes) implies that <10 mol% cholesterol is present in these membranes. | |
We overcame the challenge of poor incorporation of cholesterol into cDICE vesicles by demonstrating that cholesterol-loaded methylated β cyclodextrin (mβCD) molecules successfully deliver cholesterol to previously-formed cDICE vesicles. First, we created cDICE vesicles from a mixture of 1
:
1 DiPhyPC
:
DPPC. These vesicles exhibit only gel domains in a liquid background, as in Fig. 2. We then introduced cholesterol via mβCD as described in the methods. Fig. 3 shows a time series of a vesicle of this type after the addition of cholesterol. Domains in the membrane smoothly merge over time, as is characteristic of liquid–liquid phase coexistence, implying that the cholesterol composition now exceeds 10 mol%. This result is consistent with our conclusion that the reason that gel–liquid domains are observed in Fig. 2 is because the vesicles in Fig. 2 lack sufficient cholesterol.
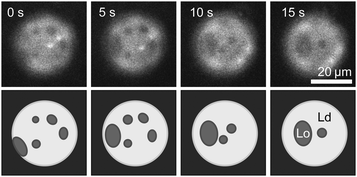 |
| Fig. 3 Time series of liquid domains diffusing and merging within the membrane of a cDICE vesicle to which cholesterol has been added via mβCD. In the lower row, schematics of domains are provided to guide the eye. The cDICE vesicle was formed using a stock oil solution containing 1 : 1 DiPhyPC : DPPC. Before the addition of cholesterol, the vesicle membrane contained gel domains on a liquid background as in Fig. 2. | |
Because the membranes in Fig. 2 exhibit only two fluorescence levels and because all vesicles from the same batch have total fluorescence levels that vary by less than a factor of two (data not shown), we conclude that vesicles made by cDICE are unilamellar. This result is consistent with findings in the literature that droplet emulsion techniques produce unilamellar vesicles.9
Charged lipids and ionic buffers
Fig. 4 shows that the cDICE method successfully creates vesicles containing entirely charged lipids, here in a ratio of 50
:
50 DiPhyPG
:
DPPG. This mixture separates into coexisting gel and liquid phases. We know of no literature values for this transition temperature. The transition temperature of 33 ± 3 °C is similar to values in the literature for transition temperatures in pure water for equimolar mixtures of DiPhyPG
:
DPPC (30 ± 2 °C), DiPhyPC
:
DPPG (34 ± 2 °C),28 and DiPhyPC
:
DPPC (35 ± 2 °C).5 More generally, the result in Fig. 2 demonstrates that there is no upper limit on the fraction of charged lipids incorporated into vesicles produced by cDICE. Our results are consistent with previous results that the presence of charged lipids does not inhibit the formation of vesicles by cDICE, and further shows that charged lipids are efficiently incorporated into vesicles.11
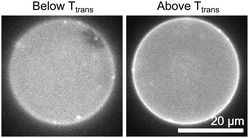 |
| Fig. 4 The images show the same cDICE vesicle composed entirely of charged lipids (50 : 50 DiPhyPG : DPPG), below and above the gel–liquid transition temperature. In the vesicle below the transition temperature, a gel domain (identified by its static, noncircular shape) appears at the top right of the image. The images were acquired at 30 °C and 35 °C, respectively. For the population of vesicles in this sample, the transition temperature was 33 ± 3 °C. | |
The cDICE method also successfully forms vesicles in solutions of phosphate buffered saline (Fig. 5). The vesicle in Fig. 5 was produced using an oil stock solution contained 50
:
50 DiPhyPG
:
DPPC. The aqueous solution contains 155 mM of monovalent salt, which is often used in vesicle preparations to produce appropriate physiological conditions for proper functioning of membrane proteins. In these experiments, the yield of vesicles produced by cDICE is similar for charged vesicles in water and in saline solution. The yield in our experiments is sufficiently high that that only a small fraction of the sample is needed for fluorescence microscopy experiments, consistent with previous reports of yields using cDICE on the order of 100 vesicles per second. Moreover, the concentration of vesicles produced by both cDICE and electroformation is much higher than is typically used in fluorescence microscopy; the same dilution protocol can be employed with both methods.
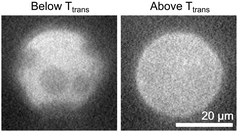 |
| Fig. 5 cDICE vesicles of 50 : 50 DiPhyPG : DPPC in phosphate buffered saline (PBS) below (left) and above (right) the gel–liquid transition temperature. The images were acquired at 25 °C and 39 °C, respectively. | |
Discussion
The cDICE method fills a niche among existing techniques for the fabrication of model membranes. The method proves to be an ideal tool to implement within experimental designs that utilize a single fabrication method when investigating stable, monodisperse GUVs with varying amounts of charged lipids at uniformly high yields. If applications require even higher yields, a new method of dispersing lipids as aggregates within oil solutions appears promising.54 Other favorable attributes of the cDICE method are that it successfully produces GUVs within buffer solutions of high salt content, and that it uses widely available materials and skills. The only pieces of equipment required are a pipette puller and a benchtop centrifuge; both are commonly found in biophysics laboratories. Several alternate methods require highly specialized fabrication techniques.
To characterize the properties of vesicles fabricated by cDICE, we evaluated phase separation in lipid bilayers. We chose this tactic for two reasons. First, assessment of phase separation is a common application of GUVs. Second, the temperature at which phase separation occurs is straightforward to quantify and is sensitive to the lipid composition of individual vesicles.
We find that vesicles created using cDICE exhibit phase separation. For vesicles composed of uncharged phospholipids, we verified that transitions occurred at the same temperature in vesicles made by the cDICE method and by a complementary technique, electroformation. The transition temperature is sensitive both to the lipid species present and to their relative abundances.5 Consequently, the agreement of transition temperatures implies that the average mixture of phospholipids in each batch of cDICE vesicles is the same as the initial composition of lipids in the stock oil solution. Vesicle-to-vesicle variations in lipid compositions yields a range of transition temperatures in cDICE vesicles of ∼3 °C, similar to the range observed in electroformed vesicles.45 Based on published phase diagrams,5 this range of temperatures limits the uncertainty in lipid composition within cDICE vesicles to less than 4 mol%.
An additional strength of the cDICE method is that it performs well in bulk solutions of high ionic strength. Typical ionic strengths employed in systems of biological relevance are greater than 100 mM. The cDICE method is successful even when GUVs containing charged lipids are produced in a solution of a higher ionic strength. This positive result is not necessarily expected given reports of strong interactions between charged solutes and anionic lipids.55 The most common methods for producing GUVs (namely, gentle hydration on a solid substrate and electroformation) have poor yields in the presence of buffers with high salt content.7 Although there have been reports in the literature of successes in modifying these procedures, as in employing gentle hydration on a polymer layer of polyvinyl alcohol,32 other modifications produce drawbacks such as an asymmetric final distribution of ions, high electric fields that may degrade lipids, or shifts in miscibility transition temperatures.36,56
The success of the cDICE method in incorporating charged lipids and ionic buffers within protocols of producing monodisperse GUVs is tempered by the method's inability to incorporate cholesterol into vesicles at significant fractions. When cholesterol is required, it must be added to previously-formed vesicles via cyclodextrin or other methods, which results in greater compositional uncertainty and more complex experimental designs. Similar challenges plague related techniques in which lipid monolayers are formed at the interface between bulk aqueous and oil phases. Whenever a method is unable to deliver a repeatable, precise, known fraction of cholesterol to model vesicles, that method's use is limited for accurately mimicking cell plasma membranes, which contain cholesterol fractions as high as 50 mol%.57
There are two promising tactics for addressing the challenges of incorporating known fractions of sterols into membranes. The first tactic involves allowing interfaces between layers of water and lipid–oil stock solutions to equilibrate for long times at high temperatures. For example, a modification of the cDICE method known as the Droplet Shooting and Size-Filtration (DSSF) uses the same principle of centrifugally propelling droplets across oil–water interfaces coated with lipid monolayers.58 An advantage of the DSSF method is that the apparatus, which is housed in a standard microcentrifuge tube, is so small that it quickly achieves a uniform temperature. After allowing lipid monolayers to form for 60 min at 40 °C, Morita et al. found that the DSSF method produced GUVs of equimolar DOPC and DPPC lipids, plus enough cholesterol to reach at least coexistence of three phases: a solid phase, an Lo phase, and an Ld phase.58 Coexistence of three phases is characterized by domains that do not merge on the surface of taut vesicles and that may be noncircular as in Fig. 6c-ii of Morita et al.58 At room temperature, three phases coexist in membranes of equimolar DOPC and DPPC at cholesterol concentrations between 10 and 15 mol%.51 Since this concentration of cholesterol is much lower than the concentration (30 mol%) used in stock solutions in the DSSF experiment,58 the DSSF method appears to incorporate only a fraction of the intended sterols into membranes, albeit a larger fraction than the cDICE method does. Disadvantages of the DSSF method are that the mean diameter of GUVs is less than 20 μm, that the number of GUVs produced is limited to hundreds, and that most GUVs appear to have defects.58
A second tactic that might be used in the future to incorporate known fractions of sterols into membranes is to tune the properties of the oil in which the lipids are dissolved. For example, Haase and Brujic (2014) found that the phase behavior of lipid monolayers at an oil/water interface changes with the fraction of cholesterol in the stock solution, indicating that cholesterol successfully partitions to the interface.59 Their studies used a silicon oil which has a significantly lower viscosity than the heavy mineral oil used in our stock oil solutions. When we performed the cDICE technique using the same silicon oil, no vesicles formed, consistent with known constraints of cDICE requiring an oil with a high viscosity.11 It is unclear if cholesterol is difficult to incorporate into cDICE vesicles because cholesterol does not readily dissolve in mineral oil and/or because cholesterol is so much more hydrophobic than phospholipids that it does not partition to the oil–water interface of a travelling droplet as efficiently.
A common concern with methods of vesicle production that involve dissolving lipids in oil is that significant oil may become trapped in the bilayer, and that this oil may affect physical properties of the membrane.7,40,60,61 The absence of an observable oil lens within cDICE vesicles implies that this method does not leave a macroscopic amount of oil in the bilayer.62 Nevertheless, complementary techniques like jetting produce vesicles that appear oil-free by optical microscopy, but can contain decane layers or lenses ∼10 nm thick.63 Thin layers or lenses of oil may be inconsequential in some applications and detrimental in others. For example, Campillo et al. pulled nanotubes both from vesicles made by a reverse emulsion technique and from vesicles made by electroformation.61 They found that static properties of the two membranes, namely the relationship between the force of pulling and the membrane tension, were the same. However, they found significant differences in the dynamic properties of the membranes. Dynamic data from reverse emulsion vesicles matched data from electroformed vesicles only when the latter were produced in the presence of oil, implying that the reverse emulsion vesicles contained residual oil. We find that vesicles produced by cDICE exhibit the same miscibility transition temperatures as those produced by electroformation, within experimental uncertainty. This result implies that any residual oil within cDICE vesicles has only a minor effect on membrane miscibility phase behavior and suggests that the effect of any residual oil on membrane packing is similarly small.
Conclusions
The cDICE method produces monodisperse GUVs that contain high fractions of charged lipids in ionic solutions. Specifically, the vesicles incorporate up to 100% charged lipids and form at ionic strengths up to physiological levels. The yield of cDICE vesicles is equally high in vesicles containing 100% charged lipids, in uncharged vesicles, and in vesicles in solutions with high ionic strengths. In conclusion, the cDICE method presents a single, widely accessible method of producing monodisperse GUVs that can be used under a variety of experimental conditions. A caveat is that the cDICE method does not incorporate substantial amounts of cholesterol into membranes.
Acknowledgements
This research was supported by the National Science Foundation MCB07444852 and MCB1402059. M. C. B. was supported by NIH Training in Molecular Biophysics T32 GMT32 GM008268. We thank Manouk Abkarian for inspiration for this work and for his generous advice about optimization of cDICE performance.
Notes and references
- M. D. Collins and S. E. Gordon, Biophys. J., 2013, 105, 2485–2494 CrossRef CAS PubMed.
- R. Rusinova, D. M. Kim, C. M. Nimigean and O. S. Andersen, Biophys. J., 2014, 106, 1070–1078 CrossRef CAS PubMed.
- A. Tsamaloukas, H. Szadkowska and H. Heerklotz, Biophys. J., 2006, 90, 4479–4487 CrossRef CAS PubMed.
-
M. C. Blosser, C. E. Cornell, S. P. Rayermann and S. L. Keller, in Giant Vesicle Book, ed. R. Dimova and C. Marques, 2016, in press Search PubMed.
- S. L. Veatch, K. Gawrisch and S. L. Keller, Biophys. J., 2006, 90, 4428–4436 CrossRef CAS PubMed.
- J. Zhao, J. Wu, F. A. Heberle, T. T. Mills, P. Klawitter, G. Huang, G. Costanza and G. W. Feigenson, Biochim. Biophys. Acta, Biomembr., 2007, 1768, 2764–2776 CrossRef CAS PubMed.
- P. Walde, K. Cosentino, H. Engel and P. Stano, ChemBioChem, 2010, 11, 848–865 CrossRef CAS PubMed.
- M. Jiménez, A. Martos, E. J. Cabré, A. Raso and G. Rivas, Environ. Microbiol., 2013, 15, 3158–3168 CrossRef PubMed.
- D. van Swaay and A. deMello, Lab Chip, 2013, 13, 752–767 RSC.
- F. C. Keber, E. Loiseau, T. Sanchez, S. J. DeCamp, L. Giomi, M. J. Bowick, M. C. Marchetti, Z. Dogic and A. R. Bausch, Science, 2014, 345, 1135–1139 CrossRef CAS PubMed.
- M. Abkarian, E. Loiseau and G. Massiera, Soft Matter, 2011, 7, 4610–4614 RSC.
-
M. I. Angelova, PhD thesis, Bulgarian Academy of Sciences, 1988.
- K. Akashi, H. Miyata, H. Itoh and K. Kinosita, Biophys. J., 1996, 71, 3242–3250 CrossRef CAS PubMed.
- C. W. Coyne, K. Patel, J. Heureaux, J. Stachowiak, D. A. Fletcher and A. P. Liu, J. Visualized Exp., 2014, e51510 Search PubMed.
- K. Funakoshi, H. Suzuki and S. Takeuchi, J. Am. Chem. Soc., 2007, 129, 12608–12609 CrossRef CAS PubMed.
- D. L. Richmond, E. M. Schmid, S. Martens, J. C. Stachowiak, N. Liska and D. A. Fletcher, Proc. Natl. Acad. Sci. U. S. A., 2011, 108, 9431–9436 CrossRef CAS PubMed.
- Y. Elani, S. Purushothaman, P. J. Booth, J. M. Seddon, N. J. Brooks, R. V. Law and O. Ces, Chem. Commun., 2015, 51, 6976–6979 RSC.
- K. Boesze-Battaglia and R. J. Schimmel, J. Exp. Biol., 1997, 200, 2927–2936 CAS.
- J. C. Holthuis and T. P. Levine, Nat. Rev. Mol. Cell Biol., 2005, 6, 209–220 CrossRef CAS PubMed.
- P. F. Devaux and A. Zachowski, Chem. Phys. Lipids, 1994, 73, 107–120 CrossRef CAS.
- G. Daum, Biochim. Biophys. Acta, Rev. Biomembr., 1985, 822, 1–42 CrossRef CAS.
- T. J. Beveridge, J. Bacteriol., 1999, 181, 4725–4733 CAS.
- M. Mouat and K. Manchester, Comp. Haematol. Int., 1998, 8, 58–60 CrossRef CAS.
- D. W. Deamer and J. Bramhall, Chem. Phys. Lipids, 1986, 40, 167–188 CrossRef CAS PubMed.
- A. Diguet, M. Le Berre, Y. Chen and D. Baigl, Small, 2009, 5, 1661–1666 CrossRef CAS PubMed.
- C. C. Vequi-Suplicy, K. A. Riske, R. L. Knorr and R. Dimova, Biochim. Biophys. Acta, Biomembr., 2010, 1798, 1338–1347 CrossRef CAS PubMed.
- N. Rodriguez, F. Pincet and S. Cribier, Colloids Surf., B, 2005, 42, 125–130 CrossRef CAS PubMed.
- M. C. Blosser, J. B. Starr, C. W. Turtle, J. Ashcraft and S. L. Keller, Biophys. J., 2013, 104, 2629–2638 CrossRef CAS PubMed.
- M. I. Angelova and D. S. Dimitrov, Faraday Discuss., 1986, 81, 303–311 RSC.
- R. Dimova, S. Aranda, N. Bezlyepkina, V. Nikolov, K. A. Riske and R. Lipowsky, J. Phys.: Condens. Matter, 2006, 18, S1151 CrossRef CAS PubMed.
- P. Méléard, L. A. Bagatolli and T. Pott, Methods Enzymol., 2009, 465, 161–176 Search PubMed.
- A. Weinberger, F.-C. Tsai, G. H. Koenderink, T. F. Schmidt, R. Itri, W. Meier, T. Schmatko, A. Schröder and C. Marques, Biophys. J., 2013, 105, 154–164 CrossRef CAS PubMed.
- D. J. Estes and M. Mayer, Biochim. Biophys. Acta, Biomembr., 2005, 1712, 152–160 CrossRef CAS PubMed.
- N. F. Morales-Penningston, J. Wu, E. R. Farkas, S. L. Goh, T. M. Konyakhina, J. Y. Zheng, W. W. Webb and G. W. Feigenson, Biochim. Biophys. Acta, Biomembr., 2010, 1798, 1324–1332 CrossRef CAS PubMed.
- K. S. Horger, D. J. Estes, R. Capone and M. Mayer, J. Am. Chem. Soc., 2009, 131, 1810–1819 CrossRef CAS PubMed.
- N. L. Mora, J. S. Hansen, Y. Gao, A. A. Ronald, R. Kieltyka, N. Malmstadt and A. Kros, Chem. Commun., 2014, 50, 1953–1955 RSC.
- S. Pautot, B. J. Frisken and D. A. Weitz, Proc. Natl. Acad. Sci. U. S. A., 2003, 100, 10718–10721 CrossRef CAS PubMed.
- A. Yamada, T. Yamanaka, T. Hamada, M. Hase, K. Yoshikawa and D. Baigl, Langmuir, 2006, 22, 9824–9828 CrossRef CAS PubMed.
-
P. C. Hu and N. Malmstadt, Methods in Membrane Lipids, Springer, 2015, pp. 79–90 Search PubMed.
- L. R. Arriaga, S. S. Datta, S. H. Kim, E. Amstad, T. E. Kodger, F. Monroy and D. A. Weitz, Small, 2014, 10, 950–956 CrossRef CAS PubMed.
-
H. C. Shum, J. Thiele and S.-H. Kim, Advances in Transport Phenomena 2011, Springer, 2014, pp. 1–28 Search PubMed.
- P. C. Hu, S. Li and N. Malmstadt, ACS Appl. Mater. Interfaces, 2011, 3, 1434–1440 CAS.
- K. Karamdad, R. V. Law, J. M. Seddon, N. J. Brooks and O. Ces, Chem. Commun., 2016, 52, 5277–5280 RSC.
- H. Ito, T. Yamanaka, S. Kato, T. Hamada, M. Takagi, M. Ichikawa and K. Yoshikawa, Soft Matter, 2013, 9, 9539–9547 RSC.
- S. L. Veatch and S. L. Keller, Biophys. J., 2003, 85, 3074–3083 CrossRef CAS PubMed.
- U. Klein, G. Gimpl and F. Fahrenholz, Biochemistry, 1995, 34, 13784–13793 CrossRef CAS PubMed.
- H. Lindsey, N. Petersen and S. I. Chan, Biochim. Biophys. Acta, Biomembr., 1979, 555, 147–167 CrossRef CAS.
-
J. Silvius, in Lipid-Protein Interactions, ed. P. C. Jost and H. Griffith, John Wiley & Sons, New York, 1982, vol. 2, pp. 239–281 Search PubMed.
- G. Van Meer, D. R. Voelker and G. W. Feigenson, Nat. Rev. Mol. Cell Biol., 2008, 9, 112–124 CrossRef CAS PubMed.
- M. E. Beattie, S. L. Veatch, B. L. Stottrup and S. L. Keller, Biophys. J., 2005, 89, 1760–1768 CrossRef CAS PubMed.
- S. L. Veatch, O. Soubias, S. L. Keller and K. Gawrisch, Proc. Natl. Acad. Sci. U. S. A., 2007, 104, 17650–17655 CrossRef CAS PubMed.
- J. Y. Huang, J. T. Buboltz and G. W. Feigenson, Biochim. Biophys. Acta, Biomembr., 1999, 1417, 89–100 CrossRef CAS.
- M. M. Stevens, A. R. Honerkamp-Smith and S. L. Keller, Soft Matter, 2010, 6, 5882–5890 RSC.
- C. Claudet, M. In and G. Massiera, Eur. Phys. J. E: Soft Matter Biol. Phys., 2016, 39, 1–6 CrossRef CAS PubMed.
- S. Hui, L. Boni, T. Stewart and T. Isac, Biochemistry, 1983, 22, 3511–3516 CrossRef CAS.
- Y. Zhou, C. K. Berry, P. A. Storer and R. M. Raphael, Biomaterials, 2007, 28, 1298–1306 CrossRef CAS PubMed.
- G. J. Nelson, J. Lipid Res., 1967, 8, 374–379 CAS.
- M. Morita, H. Onoe, M. Yanagisawa, H. Ito, M. Ichikawa, K. Fujiwara, H. Saito and M. Takinoue, ChemBioChem, 2015, 16, 2029–2035 CrossRef CAS PubMed.
- M. F. Haase and J. Brujic, Angew. Chem., Int. Ed., 2014, 53, 11793–11797 CrossRef CAS PubMed.
- R. S. Ries, H. Choi, R. Blunck, F. Bezanilla and J. R. Heath, J. Phys. Chem. B, 2004, 108, 16040–16049 CrossRef CAS.
- C. Campillo, P. Sens, D. Köster, L.-L. Pontani, D. Lévy, P. Bassereau, P. Nassoy and C. Sykes, Biophys. J., 2013, 104, 1248–1256 CrossRef CAS PubMed.
- J. Requena, D. A. Haydon and S. B. Hladky, Biophys. J., 1975, 15, 77–81 CrossRef CAS.
- S. R. Kirchner, A. Ohlinger, T. Pfeiffer, A. S. Urban, F. D. Stefani, A. Deak, A. A. Lutich and J. Feldmann, J. Biophotonics, 2012, 5, 40–46 CrossRef CAS PubMed.
|
This journal is © The Royal Society of Chemistry 2016 |
Click here to see how this site uses Cookies. View our privacy policy here.