DOI:
10.1039/C6RA23287F
(Paper)
RSC Adv., 2016,
6, 114061-114068
Layer-by-layer surface decoration of electrospun nanofibrous meshes for air–liquid interface cultivation of epidermal cells†
Received
19th September 2016
, Accepted 14th November 2016
First published on 25th November 2016
Abstract
Multilayered assembly of hydrophilic polymers is used to decorate electrospun nanofibrous mats for the air–liquid interface cultivation of epidermal cells. ε-Caprolactone is ring-opening polymerized into carboxyl-terminated poly(ε-caprolactone) (PCL) and subsequently conjugated with poly(ethyleneimine) (PEI) in order to prepare the PCL–PEI block copolymers. The electrospun PCL–PEI nanofibers are chemically conjugated with multivalent poly(ethyeleneglycol) (PEG) chains, and PEI is chemically tethered to the PEG layers in order to further decorate the surface with multiple layers of PEG. The layered PEG is characterized using both thermal properties and direct visualization of the whole mats with fluorescence labeling, which exhibit escalating levels of PEG content with increasing numbers of PEG layering processes. The PCL–PEI nanofibers with PEG multilayers exhibit superior water swelling rates compared with unmodified mats and have high water retaining behaviors for prolonged periods without additional water supply in a PEG layer-dependent manner. When epidermal cells are cultivated on the PEG-multilayered mats without a continuous supply of the cell culture medium, the viability is maintained for 48 h and gradually decreases after 48 h. The epidermis-specific genes are highly expressed in cells cultivated on the PEG-multilayered mats in comparison with those on an insert well or unmodified mats, which is confirmed by qualitative real-time polymerase chain reaction and immunocytochemical staining of total keratin. Thus, PEG-multilayered nanofibrous mats can be a novel cell culture matrix for cell culture systems where the cell cultivation conditions require air–liquid interfaces.
Introduction
Cell culture in air–liquid interfaces influences the differentiation of the modified gene expression properties and phenotypes in comparison with submerged cell cultivation.1 Air–liquid interface cultures of keratinocytes and epidermal fibroblasts have exhibited the formation of stratified and cornified epithelium layers and minimal contraction of the skin substitutes.2,3 Cells cultured in air–liquid interfaces have also been used to influence the cytotoxicity of cigarette smoke and drug screening.4 As the epidermal layer of skin has been well established with the air–liquid interface cell culture method, it is desirable to develop small scale scaffolds of air–liquid cell culture platforms for tissue reconstruction. Recently, the combination of cell support membranes and microfluidics has demonstrated that small scale air–liquid cell cultures can result in the development of dermis, epidermis, and cornified layers.5,6 Electrospun nanofibers have also been introduced to air–liquid interface cell culture scaffolds. The biological function of porcine tracheobronchial epithelial cells in the air–liquid interface environment using poly(ε-caprolactone) (PCL)/chitosan nanofibers has also been demonstrated.7 For multilayered cell cultures, keratinocytes were cultured on silk nanofibrous mesh where it was exposed to air and epithelial cells were cultured under the silk nanofiber mesh in submerged condition.8 These studies have demonstrated different phenotypes of cells and formations of artificial skins using nanofiber mesh for cell support membranes, but the media chamber always existed under the nanofiber. However, if the scaffold is to be implanted in the body, the cell layer with the membrane is applied or cell layer should be separated from the media container. Moist conditions are critical for wound tissue recovery that enhances epithelialization with less contraction of the wound site. Therefore, the tissue scaffold should have hydrophilic properties for the penetration of oxygen, storing water, and removing excess amounts of exudate that cause delays in healing.9 The importance of the moist environment has been considered in the wound healing process in numerous papers, but cell differentiation has not been considered. For remodeling of the epidermal layer in air–liquid interfaces, most studies have proposed that there is abundant liquid under the support membrane, but the influence of the appropriate amount of liquid on the air–liquid cell culture system remains debatable. Interestingly, a study on the moisture content effects on wound recovery and contraction has been published recently regarding the water penetration rate of a porous scaffold dressing on the wound bed.10 Hydrogel was predominantly developed to maintain the moisture rich environment of the wound bed and it results in enhanced epithelial cell migration rates in the healing process.11 There have been fewer nanofiber studies related to moisturized environments for wound recovery than those for hydrogel because post-treatment is required to modify the nanofiber properties to be hydrophilic.12
Easily electrospinnable synthetic polymers are primarily hydrophobic such as PCL, poly(lactic acid) (PLA), poly(lactic-g-glycolic acid), poly(urethane), etc. For application in tissue engineering, the hydrophobicity of the nanofibrous mesh should be modified to be partly hydrophilic. Introducing hydrophilic properties to hydrophobic nanofibers has been attempted in various ways. Plasma treatment of hydrophobic nanofibers changed the surface properties to hydrophilic.13 Hydrophilic poly(glycerol sebacate) has also been crosslinked to PLA scaffolds.14 Nanofibrous mesh has been chemically surface modified with diverse proteins, it exhibited a partly hydrophilic surface and enhanced cell attachment.15 In our previous studies, we developed various nanofiber meshes via surface modification using biotin, catechol, and growth factors for tissue engineering.16–19 We found that the surface decoration of the hydrophilic polymer on hydrophobic nanofibers can modify their properties to have a water friendly surface.
In this paper, we propose an air–liquid cell culture substrate that contains both moist and air-exposed environments in a device using electrospun nanofibrous mesh. A PCL–poly(ethyleneimine) (PEI) block copolymer is electrospun as a support for the fibrous structure, and hydrophilic poly(ethylene glycol) (PEG) is repeatedly surface conjugated on the PCL–PEI nanofiber in order to transform the original hydrophobic mesh to hydrophilic mesh via a crosslinker. Therefore, the cells attached on top of the mesh are supplied nutrients from the PEG-layered mesh wetted in serum-containing media, which is a non-submerged culture condition.
Experimental
Materials
ε-Caprolactone (ε-CL) was purchased from Alfa-Aesar (Ward Hill, MA). Octanoic acid was purchased from Junsei Chemical Co. (Tokyo, Japan). Tin(II)-ethylhexanoate (Sn(Oct)2), N-hydroxysuccinimide (NHS), 1,3-dicyclohexylcarbodiimide (DCC), para-nitrophenyl chloroformate (p-NPC) and fluorescamine were purchased from Sigma-Aldrich (St. Louis, MO). Branched poly(ethyleneimine) (PEI, Mw 1.8k) was purchased from Polysciences, Inc. (Warrington, PA). 4-Arm poly(ethyeleneglycol) (4-arm PEG, 40k) was purchased from Pharmicell (Seoul, Korea). Alexa Fluor® 750-NHS ester and Texas Red® isomers were purchased from Molecular Probe (Eugene, OR). Pan-cytokeratin antibody was purchased from Santa Cruz Biotechnology, Inc. (Dallas, TX). Dulbecco's modified Eagle's medium (DMEM), phosphate buffered saline (PBS) streptomycin/penicillin, and fetal bovine serum (FBS) were purchased from Life Technologies (Carlsbad, CA). All other chemicals were of analytical grade. HaCaT was kindly donated by Prof. Chang-Jin Lim (Division of Life Sciences, Kangwon National University, Chuncheon, Republic of Korea).
Synthesis of PCL–PEI block copolymer
The PCL–PEI block copolymer was prepared through conjugating carboxyl-terminated PCL to the primary amines of PEI (Scheme 1A). In order to synthesize the carboxyl-terminated PCL, ε-caprolactone (20 g) in a round-bottom flask was preheated to 60 °C in an N2 gas environment for 2 h, and octanoic acid (33.6 mg) and stannous octoate (47.2 mg) were subsequently injected to the flask. The mixture was reacted at 130 °C for 24 h and the reaction was quenched with methanol (1 mL). After cooling to room temperature, PCL was dissolved in chloroform (500 mL) and precipitated into ice-cold methanol twice. In order to synthesize the PCL–PEI block copolymer, carboxyl-terminated PCL (10 g) in anhydrous chloroform (500 mL) was reacted with DCC and NHS in DMSO in order to activate the carboxylate (molar ratio of PCL
:
DCC
:
NHS = 1
:
5
:
5). After 24 h, the carboxyl-terminated PCL was filtered through filter paper and precipitated in ice-cold methanol (3 L) in order to remove any unreacted chemicals. PCL (8.7 g) in anhydrous chloroform (65 mL) was slowly added to the PEI (1.17 g) in anhydrous chloroform (65 mL) using a syringe pump at a flow rate of 30 mL h−1, and it was further reacted at room temperature for 24 h. The reaction mixture was purified through precipitation into ice-cold methanol (3 L). The conjugation rate of PCL to PEI was confirmed using 400 Hz 1H NMR in CDCl3 (83 mg mL−1) at Central Laboratory Kangnational University and a fluorescamine assay, as previously described.20 The ethylene diamine in 1,4-dioxane was used as a standard material.
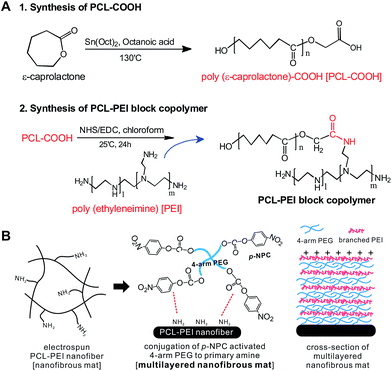 |
| Scheme 1 Scheme of the preparation of multilayered nanofibrous mat. (A) Carboxylate functionalized PCL (PCL–COOH) was synthesized and PEI was introduced to prepare PCL–PEI block copolymer. (B) Electrospun PCL–PEI nanofiber (nanofibrous mat) was surface decorated with p-NPC activated 4-arm PEG, layer by layer from 1 to 5 by introducing branched PEI for a linker between 4-arm PEGs. Each polymer layer stacking process was terminated with branched PEI for positively charged surface of multilayered nanofibrous mat. | |
Preparation of surface-layered nanofibrous mats
Post-electrospinning modification was performed on the surface of the nanofibrous mats to decorate them with multiple layers of 4-arm PEG and PEI via chemical conjugation. The PCL–PEI dissolved in a chloroform/methanol mixture (3/1, v/v) at 16% (w/v) was electrospun onto the nanofibrous mats through a 25 G needle at a speed rate of 1 mL h−1 in an electric potential of +13 kV. The nanofiber was electrospun on circular aluminum foil (∅ = 17 mm) for 1 min. The distance between the needle and the ground was 10 cm. The amount of surface-exposed primary amine on the nanofibrous mat was quantified using fluorescamine assay.21 The surface of the nanofibrous mat was decorated with multiple layered PEG. First, the hydroxyl group of the 4-arm PEG was activated with p-NPC; the 4-arm PEG (5 g) in chloroform (30 mL) was reacted with p-NPC and pyridine at a molar ratio of 4-arm PEG
:
p-NPC
:
pyridine = 1
:
8
:
20. The reaction was performed at 0 °C for 1 h and at 25 °C for 12 h; then, it was precipitated in ice-cold ether (2 L) two times in order to remove any remaining unreacted chemicals. The p-NPC activated PEG in methanol (833.33 nmol mL−1, 30 μL) was slowly dropped onto the nanofibrous mat (2.7 mg) and incubated for 30 min. The PEI in methanol (333 nmol mL−1, 30 μL) was subsequently dropped onto the first layer of the PEG decorated mat and incubated for 30 min. In order to stack multiple layers of PEG on the nanofibrous mats, the 4-arm PEG/PEI layering cycle was repeated five times. After the decoration, the nanofiber was washed in methanol five times and air dried. The amount of 4-arm PEG conjugated on the nanofibrous mat was quantified via the weight change in the dried nanofiber.
Characterization of the PEG-multilayered nanofibrous mats
The morphology of the PEG-multilayered nanofibrous mat was confirmed using field-emission scanning electronic microscopy (FE-SEM; Hitachi, S-4300, Japan) in the Central Laboratory of Kangwon National University (Korea). In order to visualize the PEG and PEI on the nanofibrous mat, they were labelled separately with fluorescence dyes according to the manufacturer's protocols with a minor modification. Ethylene diamine (3 μg) in methanol (1 mL) was slowly dropped into Texas Red® isomer (61 μg) in methanol (0.25 mL) and incubated for 2 h. The 4-arm PEG (40 mg) in methanol (10 mL) was slowly dropped into the mixture in order to prepare the Texas Red labelled 4-arm PEG. The unreacted ethylene diamine and Texas Red were removed via dialysis (molecular weight cut-off = 1000) for 24 h and freeze dried. For the fluorescent labelling of PEI, Alexa 750-NHS (0.34 mg) was reacted with PEI (1 mg) in methanol (1 mL) at 25 °C for 2 h. The unreacted Alexa 750-NHS was removed via dialysis (molecular weight cut-off = 1000) for 24 h. The fluorescently labelled PEG and PEI were conjugated to the nanofibrous mat using the same method described above and fluorescently visualized using an in vivo imaging system (IVIS 200, PerkinElmer, Waltham, MA, U.S.A.) at the Korea Basic Science Institute (KBSI). The Texas Red-labelled PEG and Alexa 750-labelled PEI were detected using DsRed (500–550 nm excitation and 575–650 nm emission pass band) and Cy 5.5 (615–665 nm excitation and 695–770 nm emission pass band) filters, respectively.
In order to determine the increasing PEG layers on the nanofibrous mats, the thermal properties of the PEG-multilayered nanofibrous mats were investigated using differential scanning calorimetry (DSC; Q2000, TA Instruments, USA) in a temperature range of 0 °C to 100 °C in the Central Laboratory of Kangwon National University. The temperature was increased to 100 °C at a rate of 10 °C min−1, cooled to 0 °C at a rate of 10 °C min−1, and re-heated to 100 °C at a rate of 10 °C min−1. The Fourier Transform Infrared Spectroscopy (FTIR; Nicolet 6700, Thermo Fisher Scientific, Inc., USA) recorded the solid state PEG layered nanofiber from 650 cm−1 to 4000 cm−1 to confirm PEG conjugation on the mat. The hydrophilicity of the mats was tested to determine the water swelling ratio and water retaining ability according to the increase of the PEG content on the PCL–PEI nanofiber. The mat was immersed in distilled water and incubated at room temperature for 6 h; then, the excess water on the mat was removed using Kimwipes for 10 s. The water swelling ratio was calculated using the following equation where Ws is the water swollen weight of the PEG layered nanofiber and Wi is the initial weight of PEG layered nanofiber:
In order to use the multilayered nanofibrous mat in an air–liquid interface animal cell culture, the water retaining ability was also measured in high humidity conditions in order to determine how long the mat can retain the bound water in simulated cell culture conditions. Therefore, the PEG-layered nanofiber was wetted with distilled water (30 μL), placed on a 12-well plate, and incubated in an incubator at 37 °C, 5% CO2, and high humidity. The remaining water ratio was calculated using the following equation where Wn is the initial weight of the wet NF and Wd is the weight of the dried NF:
Cell culture at the air–liquid interface
The mat (circular shape, ∅ = 17 mm, 226 mm2) was sterilized in 70% ethanol with UV irradiation overnight. After washing with PBS twice, the mat was placed on a 12 well tissue culture plate. A HaCaT suspension in DMEM with 10% FBS (1 × 107 cells per mL; 30 μL) was slowly dropped onto the nanofibrous mat and fresh media (100 μL) was fed every 48 h. In order to evaluate the proliferation rate, the cells on the mat were gently washed with PBS, and the MTT reagent (5 mg mL−1, 50 μL) was treated in a 37 °C, 5% CO2 incubator for 4 h. The formazan crystals were dissolved in DMSO (100 μL) and the absorbance was measured at 540 nm.
Phenotyping of the cultivated epidermal cells
In order to evaluate the phenotype of the cells on the mat, the HaCaT cells proliferated for 7 and 14 days were dissolved in Trizol® in order to extract the mRNA according to the manufacturer's protocol. The total mRNA was synthesized to cDNA using 5× Reverse Transcription Premix at 42 °C for 60 min. The gene expression level of GAPDH, keratin 14 (KRT14), loricrin (LOR), filaggrin (FLG), and involucrin (IVL) were investigated using real-time PCR with SYBR; 500 ng of cDNA was amplified by 38 cycles of 94 °C for 20 s, 52 °C for 30 s, 72 °C for 30 s, and melted from 52 °C to 90 °C in 0.5 °C increments. The sequence of the primers and melting temperatures are listed in Table S1 in the ESI.†
Cell imaging
The total keratin expression by the cells proliferated on the PEG layered nanofiber was immunochemically stained. The pan keratin primary antibody in PBS was labelled with Texas Red-NHS with a molar ratio of 1
:
1.2 and incubated overnight at 4 °C. The Texas Red-labelled pan keratin was purified using a centrifugal filter (molecular weight cut-off = 30
000; Millipore). The cells on the nanofiber were fixed in 4% formaldehyde for 15 min and treated with 0.2% Triton-X in PBS for 1 h at room temperature for permeabilization. After incubation in 1% BSA containing PBS for 2 h for blocking, the Texas Red conjugated pan keratin (1 μg mL−1) was incubated overnight at 4 °C. After washing with PBS, the cells were imaged using an in vivo imaging system (IVIS) with a DsRed filter.
Results and discussion
In order to increase the water absorbing ability on the hydrophobic surfaces of the PCL–PEI nanofiber (NF+), 4-arm PEG was multi-layered on the surface through chemically conjugating the p-NPC activated PEG to the surface-exposed primary amines on the NF+ as depicted in Scheme 1. NF+, which is a platform for continuing the PEG layering, was fabricated via electrospinning PCL–PEI, which is a block copolymer with a high density of primary amines. The 1H NMR analysis of the synthesized PCL–PEI indicated that two moles of PCL were conjugated to one mole of PEI, which is that the PEI content in the PCL–PEI was 33.33% (mol mol−1) (Fig. S1†). Considering the primary, secondary, and tertiary amine composition rates of 25
:
50
:
25 in the branched PEI, the branched PEI of Mw 1.8k contains 10 primary amines.22,23 Based on this, it was calculated that 61.4 nmol mg−1 of primary amines remained in the PCL–PEI block copolymer. The PCL/PEI ratio was reconfirmed through measuring the remaining primary amines of the PCL–PEI block copolymers using fluorescamine assay; the amount of primary amine groups in the PCL–PEI block copolymer was 58.34 ± 10.04 nmol mg−1, which is a similar value to the 1H NMR. When PCL–PEI was electrospun, being NF+, the amount of surface-exposed amines was 30.79 ± 1.48 nmol mg−1 of the nanofiber. In comparison with our previous study of electrospun nanofiber using an amine-terminated PCL–PEG block copolymer, which was 4.03 nmol mg−1 of amines on it, NF+ showed seven times higher value because of branched PEI.24
The PEG multilayers were chemically stacked on the amine functionalized NF+ via conjugating the p-NPC activated 4-arm PEG to the primary amines of PEI, which is a crosslinker tethering the respective layers (Scheme 1B). The thermal properties of the multilayered nanofibrous mats were influenced by the repeated PEG layering on NF+. When the melting and crystallization temperatures were investigated using differential scanning calorimetry (DSC), the melting temperature (Tm) of NF+ was confirmed to be 54.98 °C and gradually increased with increasing amount of PEG decoration (Table 1, Fig. S2†). When there were more than two layers of PEG on NF+, bimodal exothermic peaks (Tc) appeared. The melting enthalpy (ΔHm) was also influenced by the PEG decoration, where it increased at approximately 43 °C, and it decreased with the PCL–PEI at approximately 32–34 °C.25 When the crystallinity was calculated with respect to the ΔHm (91.16 J g−1) of PCL, the values gradually increased from 34.93% to 57.89% depending on the increase of the PEG layers from NF+ to NF-PEG 5+, respectively. The compositional analysis of the multilayered nanofibrous mat was also performed using FTIR spectroscopy to confirm the successful chemical tethering between the primary amines of PEI and p-NPC activated PEG (Fig. S3†). The CH2 stretching at 2800 cm−1 clearly indicates multiple layers of PEG on the mats. In addition, as the number of PEG layers increased, the characteristic peaks of PEG were clearly detected at 1365 cm−1 (–OH vibration), 1290 cm−1 (crystallization region of PEG),26 1100 cm−1 (C–O–C), and 1060 cm−1 (C–O stretching).24,25 In order to examine the effects of the multi-layering, the morphological features of the mat were examined using FE-SEM and IVIS after multiple PEG layering on the NF+ (Fig. 1). The SEM images revealed that the respective fibers became thicker when the PEG layers increased from NF+ to NF-PEG 5+ (Fig. 1A). Specifically, NF-PEG 5+ exhibited an average fiber diameter of 1135 ± 218 nm while the unmodified NF+ was 502 ± 61 nm, which corresponds to a 2.3-fold increase in diameter after five layers of PEG on the NF+. However, it should be noted that the fibrous structures of the mat were maintained after the repeated layering of PEG although the surface of the decorated NF+ tended to be smoother due to the tethering of the PEG chains.27,28 In order to visualize the decorated PEG layers on the NF+, the 4-arm PEG and PEI were fluorescently tagged with Texas Red and Alexa Fluor 750, respectively, and the fluorescence intensity was visualized by IVIS (Fig. 1B). When the Texas Red-PEG was observed on the mats, it was found that the fluorescence intensity gradually increased depending on the number of PEG layers from one to five layers, which indicates that the PEG layers became thicker upon repeated layering. The fluorescence of the Alexa Fluor 750-PEI also exhibited similar intensity properties depending on the number of PEG layers. These results clearly indicate that the surfaces of the mats were chemically decorated with PEG chains crosslinked with PEI.
Table 1 PEG contents (%, w/w) in multilayered nanofibrous mat and thermal property by DSC
|
PEG contents in multilayered nanofibrous mata (%, w/w) |
Tm (°C) |
ΔHm (J g−1) |
Tc (°C) |
Crystallinityb (%) |
4-Arm PEG contents in total weight of nanofiber = {(the weight of PEG layered nanofiber) − (the weight of nanofiber)}/(the weight of PEG layered nanofiber) × 100. Crystallinity (%) = {(ΔHm,sample)/(ΔHm,PCL)} × 100. |
NF+ |
— |
54.98 |
31.84 |
32.55 |
34.93 |
NF-PEG 1+ |
26.3 ± 5.1 |
54.95 |
39.80 |
32.77 |
43.66 |
NF-PEG 2+ |
42.8 ± 2.9 |
55.30 |
40.82 |
34.96/43.24 |
44.78 |
NF-PEG 3+ |
52.5 ± 3.0 |
55.23 |
46.47 |
32.76/43.44 |
50.98 |
NF-PEG 4+ |
59.3 ± 3.3 |
56.31 |
58.87 |
32.15/43.16 |
64.58 |
NF-PEG 5+ |
64.3 ± 2.4 |
56.59 |
52.77 |
33.91/42.86 |
57.89 |
4-Arm PEG |
— |
56.77 |
79.08 |
43.17 |
86.75 |
PCL |
— |
64.68 |
91.16 |
— |
— |
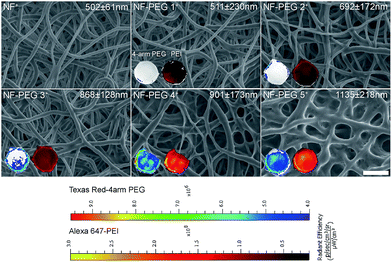 |
| Fig. 1 Visualization of PEG multilayered nanofibrous mats to fluorescently confirm PEG and PEI. Fibrous morphology was confirmed by SEM. Inset images are fluorescence image of multilayered nanofibrous mats with Texas Red-4-arm PEG and Alexa 647-PEI by IVIS (Texas Red; DsRed filter, Alexa 647; Cy 5.5 filter). Scale bar in SEM image indicates 5 μm. Colour scales of fluorescence images for Texas Red-4-arm PEG and Alexa 647-PEI were separately displayed. | |
The immediate outcome resulting from the repeated PEG tethering was that the water-swelling properties of the modified surfaces were significantly increased. When the water swelling was investigated with respect to the total weight of the decorated mat, the water-swelling property was significantly enhanced depending on the PEG layering (Fig. 2A): the swelling ratio of NF-PEG 1+ was 217.55 ± 10.57%, but after the fifth layer of PEG, this value increased to 623.99 ± 77.43%, which is a 2.9-fold increase. These values were re-calculated to the weight of only PEG layers on the NF+ in order to investigate the effects of chemical crosslinking on the swelling ratio. As depicted in Fig. 2B, the water swelling of one layer PEG (NF-PEG 1+) was 1048.06 ± 76.16%, which was the highest value among the multilayered nanofibrous mats. However, the degree of water swelling decreased significantly with the second layer of PEG and gradually increased with repeated PEG layering: 658.39 ± 43.79%, 685.61 ± 123.87%, 799.09 ± 95.23%, and 1021.67 ± 128.43% for NF-PEG 2+, NF-PEG 3+, NF-PEG 4+, and NF-PEG 5+, respectively. The significant decrease of the swelling ratio after the second layer can be clearly attributed to the increase in crosslinking density through the PEI mediated tethering, which induced a high degree of crosslinking between the PEGs. Thus, the crosslinking density of the PEI layers in NF-PEG 2+ could be significantly higher than those in NF-PEG 1+ through the PEI–PEG crosslinking, which consequently resulted in the significant decrease in the swelling ratio. However, the swelling ratios recovered rapidly from NF-PEG 2+ to NF-PEG 5+ because the PEG content in the mat increased upon subsequent PEG layering. Previous studies using PEG hydrogels indicated that the increased crosslinking density of PEG significantly decreased the swelling ratio of the hydrogels.29,30 Thus, we concluded that the PEG layers were chemically crosslinked via the PEI crosslinkers and the number of PEG layers increased the swelling ratio dependent on the PEG weight.
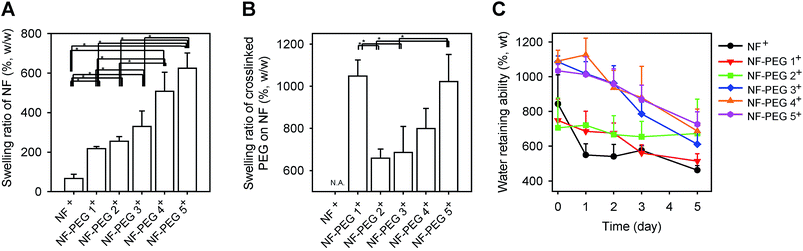 |
| Fig. 2 Hydrophilic property of PEG layered nanofibrous mats. Swelling ratio was quantified to (A) the dry weight of nanofiber and (B) crosslinked PEG weight on nanofibrous mat. (C) Water keeping ratio of multilayered nanofibrous mat in high humidity atmosphere was displayed for 5 days. Data shown as mean ± SD, n = 8. * indicates statistical significance level of P < 0.001 by one way ANOVA. N.A. is not available. | |
In order to test the multilayered nanofibrous mat as a cell cultivation medium for air–liquid interfaces, we prepared a water wetted mat and incubated it in a high humidity chamber to test the water retaining ability of the mat without additional water supply (Fig. 2C). In air–liquid interfaces, water continuously evaporates and the water retention ability of the substrate is critical in maintaining the viability of the cultivated cells on the mat. Thus, this simulation result supports the mat being used for cell cultivation at air–liquid interfaces. The water retention ability of the mats exhibited greater ability in comparison with NF+ for the initial period. In particular, NF+ exhibited a significant decrease in water retention from 843.98% to 549.79%, where the 294.19% decrease in water retention was over a period of 24 h. In contrast, NF-PEG 1+ to NF-PEG 5+ exhibited 62.98% to 21.7% decrease in water retention for 24 h and 72 h, respectively. The mats with high PEG content exhibited high degrees of water content in comparison with those with lower PEG content over a 72 h period; however, they lost a relatively larger amount of water after 72 h. This result clearly indicates that the PEG multilayers maintained higher water content for 72 h; however, after 72 h, all mats lost significant amounts of water and maintained similar levels as the surfaces without the PEG multilayers. Although the bound water on NF+ was immediately in equilibrium with the surrounding moisture, the entropy of the bound water on the PEG multilayers slowly attained thermodynamic equilibrium due to the strong hydrogen bonding between the water and the PEG chains. The bound water finally equalized at the same level of the vapour pressure in the incubator after 72 h. Consequently, through tethering the PEG multilayers on NF+, we could slow the vaporization of the bound water from the mat more than NF+, thus confirming that the mat can offer an analogous moisturizing surface for at least 72 h without additional supply of the cell culture medium. Although similar nanofibrous systems have been developed for air–liquid interface cell cultivation, they require a continuous supply of the culture medium and these systems do not offer moisturized conditions by themselves.8 Thus, we believe that this matrix offers a minimized and simplified scaffold for an air–liquid interface cultivation system because the minimum moisture is maintained with additional supplies of the culture medium every 72 h.
In order to test the feasibility of the multilayered nanofibrous mat as a new cell culture matrix at air–liquid interfaces, we cultivated a HaCaT cell, which is an immortalized keratinocyte, on the mat with a minimized supply of cell culture medium as depicted in Fig. 3A. As the cellular metabolism was modified in air–liquid interface culture, the matrix for air–liquid interface cell culture have been developed that is applicable to transfer the cell layer with the device.8 However, the study focused on the nanofibrous mesh effect and low cell cytotoxicity. Conventionally, the air–liquid cultivation of epidermal cells is performed on an insert well maintaining the culture media level to the same level of the cell layer; it was used as a control for the mats.31 When we determined the viability of cultivating cells on the mats, it was increased with the number of PEG layers for 72 h, as seen in Fig. 3B. Because the water retention ratio is differentiated for 72 h as depicted in Fig. 2C, it influences the cell proliferation rate and NF+ exhibited less cell viability than the other groups even though the initial cell binding rate for 18 h was higher than the PEG-layered groups. The initial cell binding of the multilayered nanofibrous mats was lower than NF+ because PEG hinders cell binding even when the top of the layer is positively charged with PEI.32 The cells on the insert well exhibited significantly enhanced cell viability for 72 h when confluent cell culture media was applied under the membrane.
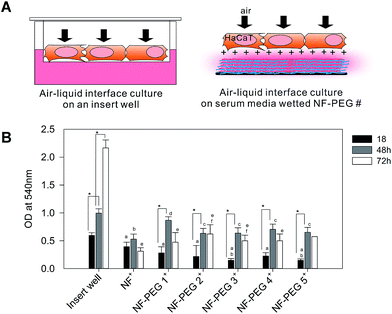 |
| Fig. 3 Cell culture on nanofibrous mat. (A) Scheme of HaCaT cell cultured on an insert well and multi-layered nanofibrous mats and (B) cell viability to NF+ by MTT assay OD at 540 nm. Cell culture medium was fed to NF-PEG # only every 2 days enough to wet the mats and no additional supply of aqueous medium was performed while cells on the insert well were continuously contacting with the medium during the cultivation period. (a, c, and e) p < 0.001 to the insert well for 18 h, 48 h, and 72 h; (b) p < 0.001 to NF+ for 18; (d and f) p < 0.05 to NF+ for 48 h and 72 h. * indicates p < 0.001. | |
In order to investigate the effect of the air–liquid interface cultivation on the keratinocyte, we also examined the gene expression levels of the keratinocyte specific markers keratin 14 (KRT14), loricrin (LOR), filaggrin (FLG), and involucrin (IVL) in Fig. 4A. Several studies have previously indicated that these markers are expressed at different levels in response to the environment even though the immortalized keratinocytes were cultivated.33,34 When the gene expression profile of the HaCaT cells on the mat was monitored on day 7 and day 14, significant differences were not observed between those on the insert well and those on the multilayered nanofibrous mats. Because keratinocytes and the keratinocyte cell line require more than 14 days to produce a complete stratum corneum,34 we observed the down-regulated KRT14 on day 14, which indicated the modified epidermal cell shape and inhibited cell proliferation.33 Furthermore, the LOR, FLG, and IVL related to the stratum corneum layer were significantly increased in the NF-PEG 1+ among the mats with a statistical significance on day 14; in comparison with those on the insert well, LOR, FLG, and IVL exhibited 5.4-, 1.9-, and 2.1-fold increases in gene expression levels on NF-PEG 1+. Overall, the gene expression properties demonstrated that the cells cultivated on the mat had more cornified properties than typical air–liquid cell culture systems using the insert well. The cells on NF-PEG 2+ (day 14) also exhibited higher expression of LOR and FLG compared with those on the insert well; however, the differences were not as high as those on NF-PEG 1+. We suppose that the more quickly dried NF-PEG 1+ among PEG layered nanofibrous mesh was strongly related to the modification of HaCaT phenotype to cornified properties.
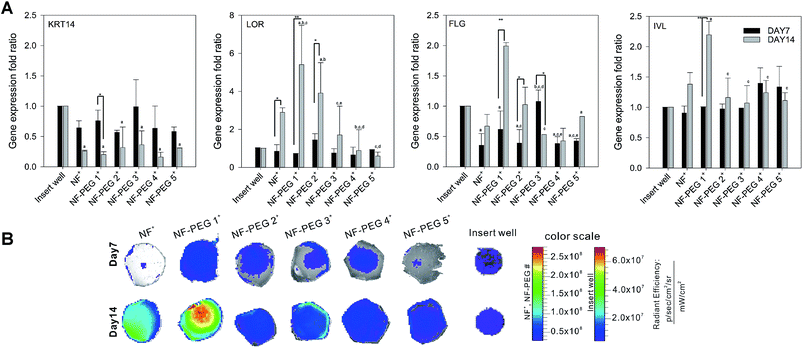 |
| Fig. 4 (A) Gene expression property of HaCaT cells cultured on multilayered nanofibrous mats for 14 days by qRT-PCR (KRT 14; keratin 14, LOR; loricrin, FLG; filaggrin, IVL; involucrin). (B) Immunocytochemical staining of total keratin of HaCaT cells proliferated on multilayered nanofibrous mat for 14 days using Alexa 647-pan keratin antibody visualized by IVIS with DsRed filter. Color scale of multilayered nanofibrous mat and an insert well were separately displayed because self-fluorescence of multilayered nanofibrous mat was stronger than the insert well. (a, b, c, d, e, f and g) p < 0.001 to the insert well, NF+, NF-PEG 1+, NF-PEG 2+, NF-PEG 3+, NF-PEG 4+, and NF-PEG 5+, respectively at each of time point; * and ** indicate p < 0.05 and p < 0.001. Statistical significance was analyzed by on way ANOVA. | |
The HaCaT cells on the mats were fluorescently visualized using pan cytokeratin via IVIS as presented in Fig. 4B. The keratin expression level of the cells on NF-PEG 1+ was the most intense and the fluorescence intensity gradually diminished as the number of PEG layers on the mat increased on day 7. The keratin expression levels became higher in those on NF-PEG 1+ on day 14 and did not exhibit significant differences to those of the rest of the group. Thus, together with the results of RT-PCR in Fig. 4A, this result clearly indicates that NF-PEG 1+ greatly increased the expression of keratinocyte-specific genes in HaCaT cells. Although the water retaining ability of the mat increased as the number of PEG layers increased, it was found that there was an inverse relationship between the number of PEG layers and the optimized environment for the cultivation of keratinocytes. Many previous studies indicated that air–liquid interfaces are advantageous in boosting the expression of keratinocyte specific genes, however none of them demonstrated the optimized level of moisture in air–liquid interface cultivation.8,35
Moreover, the PEG multilayered nanofiber mesh is potentially expendable to the device to evaluate cell differentiation on air–liquid interface that many of previous studies regulated the cellular differentiation, metabolism, and gene expression properties on the nanofiber matrix.36,37 In this study, it was found that the minimized level of water content in the air–liquid interfaces is sufficient to enhance the function of the keratinocytes, where those cultivated on NF-PEG 1+ exhibited the highest expression levels of the associated genes. However, the excess amount of PEG layers in the mat was disadvantageous in cell attachments and consequently decreased the cell proliferation.
Conclusions
The hydrophobic properties of PCL–PEI nanofibers were transformed into hydrophilic properties with PEG multilayering for air–liquid interface cell culture devices. The cells on the multilayered nanofibrous mats supplied nutrients and moisture from the mat, and they were simultaneously exposed to air. Because the mat contained the cell culture media and the PEG layers delayed the media evaporation speed, the cells proliferated on the mat for 48 h with a small volume of cell culture media.
Acknowledgements
This work was supported by National Research Foundation (NRF) in Republic of Korea (grant #2016H1D5A1908329).
References
- L. I. Bernstam, F. L. Vaughan and I. A. Bernstein, In Vitro Cell. Dev. Biol., 1986, 22, 695 CAS.
- R. Fleischmajer, E. D. MacDonald 2nd, P. Contard and J. S. Perlish, J. Histochem. Cytochem., 1993, 41, 1359 CrossRef CAS PubMed.
- R. Gauvin, D. Larouche, H. Marcoux, R. Guignard, F. A. Auger and L. Germain, J. Tissue Eng. Regener. Med., 2013, 7, 452 CrossRef CAS PubMed.
- A. Asai, T. Okuda, E. Sonoda, T. Yamauchi, S. Kato and H. Okamoto, Pharm. Res., 2015, 33, 487 CrossRef PubMed.
- H. E. Abaci, K. Gledhill, Z. Guo, A. M. Christiano and M. L. Shuler, Lab Chip, 2015, 15, 882 RSC.
- R. Rahim, O. Manuel, D. Amy, P. Tejasvi, R. D. Mehmet, K. Ali, G. Amir and Z. Babak, J. Micromech. Microeng., 2015, 25, 055015 CrossRef.
- C. Mahoney, D. Conklin, J. Waterman, J. Sankar and N. Bhattarai, J. Biomater. Sci., Polym. Ed., 2016, 27, 611 CrossRef CAS PubMed.
- H. Wendt, A. Hillmer, K. Reimers, J. W. Kuhbier, F. Schäfer-Nolte, C. Allmeling, C. Kasper and P. M. Vogt, PLoS One, 2011, 6, e21833 CAS.
- J. S. Boateng, K. H. Matthews, H. N. E. Stevens and G. M. Eccleston, J. Pharm. Sci., 2008, 97, 2892 CrossRef CAS PubMed.
- R. Xu, H. Xia, W. He, Z. Li, J. Zhao, B. Liu, Y. Wang, Q. Lei, Y. Kong, Y. Bai, Z. Yao, R. Yan, H. Li, R. Zhan, S. Yang, G. Luo and J. Wu, Sci. Rep., 2016, 6, 24596 CrossRef CAS PubMed.
- B. Balakrishnan, M. Mohanty, P. R. Umashankar and A. Jayakrishnan, Biomaterials, 2005, 26, 6335 CrossRef CAS PubMed.
- X. Liu, T. Lin, J. Fang, G. Yao, H. Zhao, M. Dodson and X. Wang, J. Biomed. Mater. Res., Part A, 2010, 94, 499 Search PubMed.
- P. P. Molamma, J. Venugopal, K. C. Casey and S. Ramakrishna, Nanotechnology, 2008, 19, 455102 CrossRef PubMed.
- H. Shi, Q. Gan, X. Liu, Y. Ma, J. Hu, Y. Yuan and C. Liu, RSC Adv., 2015, 5, 79703 RSC.
- W. He, Z. Ma, T. Yong, W. E. Teo and S. Ramakrishna, Biomaterials, 2005, 26, 7606 CrossRef CAS PubMed.
- H. S. Kim and H. S. Yoo, Acta Biomater., 2013, 9, 7371 CrossRef CAS PubMed.
- Y. I. Cho, J. S. Choi, S. Y. Jeong and H. S. Yoo, Acta Biomater., 2010, 6, 4725 CrossRef CAS PubMed.
- J. S. Choi, P. B. Messersmith and H. S. Yoo, Macromol. Biosci., 2014, 14, 270 CrossRef CAS PubMed.
- Y. J. Son, J. Kang, H. S. Kim and H. S. Yoo, Biomacromolecules, 2016, 17, 1067 CrossRef CAS PubMed.
- J. Kang and H. S. Yoo, Biomacromolecules, 2014, 15, 2600 CrossRef CAS PubMed.
- J. S. Choi, K. W. Leong and H. S. Yoo, Biomaterials, 2008, 29, 587 CrossRef CAS PubMed.
- S. C. McBain, H. H. P. Yiu, A. El Haj and J. Dobson, J. Mater. Chem., 2007, 17, 2561 RSC.
- A. von Harpe, H. Petersen, Y. Li and T. Kissel, J. Controlled Release, 2000, 69, 309 CrossRef CAS PubMed.
- Y. J. Son and H. S. Yoo, J. Biomed. Mater. Res., Part A, 2012, 100, 2678 CrossRef PubMed.
- G. Sekosan and N. Vasanthan, J. Polym. Sci., Part B: Polym. Phys., 2010, 48, 202 CrossRef CAS.
- P. Kolhe and R. M. Kannan, Biomacromolecules, 2003, 4, 173 CrossRef CAS PubMed.
- H. J. Lee, Y. H. Park and W.-G. Koh, Adv. Funct. Mater., 2013, 23, 591 CrossRef CAS.
- C. Y. Li, W. Yuan, H. Jiang, J. S. Li, F. J. Xu, W. T. Yang and J. Ma, Bioconjugate Chem., 2011, 22, 1842 CrossRef CAS PubMed.
- K. Y. Lee, J. A. Rowley, P. Eiselt, E. M. Moy, K. H. Bouhadir and D. J. Mooney, Macromolecules, 2000, 33, 4291 CrossRef CAS.
- E. A. Phelps, N. O. Enemchukwu, V. F. Fiore, J. C. Sy, N. Murthy, T. A. Sulchek, T. H. Barker and A. J. García, Adv. Mater., 2012, 24, 64 CrossRef CAS PubMed.
- S. Papini, D. Cecchetti, D. Campani, W. Fitzgerald, J. C. Grivel, S. Chen, L. Margolis and R. P. Revoltella, Stem Cells, 2003, 21, 481 CrossRef PubMed.
- P. Kingshott, H. Thissen and H. J. Griesser, Biomaterials, 2002, 23, 2043 CrossRef CAS PubMed.
- H. Alam, L. Sehgal, S. T. Kundu, S. N. Dalal and M. M. Vaidya, Mol. Biol. Cell, 2011, 22, 4068 CrossRef CAS PubMed.
- A. Deyrieux and V. G. Wilson, Cytotechnology, 2007, 54, 77 CrossRef PubMed.
- T. Sun, S. Mai, D. Norton, J. W. Haycock, A. J. Ryan and S. MacNeil, Tissue Eng., 2005, 11, 1023 CrossRef CAS PubMed.
- G. Jin, M. P. Prabhakaran and S. Ramakrishna, Acta Biomater., 2011, 7, 3113 CrossRef CAS PubMed.
- C. R. Yoo, I.-S. Yeo, K. E. Park, J. H. Park, S. J. Lee, W. H. Park and B.-M. Min, Int. J. Biol. Macromol., 2008, 42, 324 CrossRef CAS PubMed.
Footnote |
† Electronic supplementary information (ESI) available. See DOI: 10.1039/c6ra23287f |
|
This journal is © The Royal Society of Chemistry 2016 |
Click here to see how this site uses Cookies. View our privacy policy here.