DOI:
10.1039/C6RA22869K
(Paper)
RSC Adv., 2016,
6, 105930-105939
A novel pyrazole based single molecular probe for multi-analyte (Zn2+ and Mg2+) detection in human gastric adenocarcinoma cells†
Received
13th September 2016
, Accepted 29th October 2016
First published on 31st October 2016
Abstract
A new fluorescent sensor, 5-methyl-1H-pyrazole-3-carboxylic acid (6-methoxy-naphthalen-2-ylmethylene)-hydrazide (PYN), composed of a naphthalene group as the fluorogenic unit and a pyrazole carbohydrazide as the binding unit for metal ions has been designed and synthesized. The sensor shows excellent selectivity and sensitivity with a fluorescence enhancement towards Zn2+ and Mg2+ over other cations in aqueous acetonitrile solution. Turn-on fluorescent enhancements (FE) as high as ∼49 fold and ∼41 fold in mixed media for Zn2+ and, Mg2+ respectively were noticed. The signal enhancement of the sensor is based on chelation-enhanced fluorescence (CHEF) effect of PYN-Zn2+/Mg2+ with the inhibition of the photoinduced electron transfer (PET) effect. Moreover, the Job's plot established 1
:
1 stoichiometry of the complex formation between PYN and Zn2+ or Mg2+ ions. The limit of detection for Zn2+ and Mg2+ is as low as 2.2 × 10−7 M and 3.9 × 10−7 M respectively. PYN exhibited a second mode of selectivity for Zn2+ as it displaces Mg2+ from the PYN-Mg2+ complex. Density Functional Theory (DFT) calculations have been performed in order to show the structure and electronic properties of PYN and its complexes [PYN-Zn2+/Mg2+]. Cell imaging experiments confirmed that PYN can be used for monitoring intracellular Zn2+ and Mg2+ levels in living cells in vitro.
Introduction
Metal ion detection, both in the environment and in biological organisms, continues to be a thrust area of research in various fields of science.1 In particular, sensing multi-ion responsive molecular sensors with multiple emission modes are challenging task.2 Chemosensor with multiple analytes are cost-effective and highly advantageous for real-time applications. Fluorescent probe with selectivity for multimetal ions have received a great deal of attention in recent years. For example, multimetal ion sensors include those that show a different response to Cr3+/Al3+,3 Cr3+/Fe3+,4 Mn2+/Ag+,5 Fe2+/Fe3+,6 Fe3+/Cu2+,7 Cu2+/Zn2+,8 Cu2+/Hg2+,9 and Zn2+/Al3+.10
Mobile zinc ions, the second most abundant transition metal ion, takes part in a critical role in biological processes such as neurotransmission, signal transduction, mammalian reproduction and gene expression.11 Zinc imbalance is closely associated with many health problems, such as prostate cancer, delayed sexual maturation and impotence, type 2 diabetes mellitus (T2DM), Wilson's disease, amyotrophic lateral sclerosis (ALS) and age-related macular degeneration (AMD).12 In addition, free zinc ion is also involved in regulation of programmed cell death (i.e., apoptosis).13 To gain an insight into the crucial role of zinc ion in biological processes, a variety of fluorescent zinc sensors have been accepted and many of them have been effectively applied in vivo.14 It is seen in the literature that various fluorophores such as quinoline, naphthalimide, bipyridyl, bodipy, rhodamine, pyrene, phenanthroline, coumarin, etc. have been used as fluorescent Zn2+ sensors.14 Despite their interesting features, fluorescent zinc sensors have some intrinsic drawbacks, especially their short emission lifetimes, resulting in signal contamination by autofluorescence and scattered light, and hence greatly reducing the signal credibility. Since the zinc ion itself is spectroscopically silent due to its 3d10 electron configuration,15 the development of highly sensitive and selective fluorescent sensors for zinc ions is therefore of vital importance. Some available Zn2+ sensors16 have difficulty in distinguishing Zn2+ from Cd2+, since cadmium is in the same group of the periodic table and has similar properties. Therefore, the design of a highly selective and sensitive fluorescence sensor for Zn2+ detection without interference from other metal ions, especially Cd2+, is one of the most vital objectives.
Similarly, magnesium is the second most plentiful cation in intracellular fluids and fourth most abundant element in the human body.17 It is the heart of chlorophyll molecule, which plants use to convert carbon dioxide to glucose. Most enzyme activities, cell functions, and electrolytes levels are directly or indirectly dependent on magnesium.18 It controls neuronal activity, neuromuscular transmission, cardiac excitability, and blood pressure. From the health point of view, the daily intake of magnesium for an adult human should reach ca. 300 mg, which may come from green vegetables, dairy products, grains and seafood.19 A low Mg2+ activity has been observed in the patients suffering from migraine, and cardiac diseases.20 On the other hand, its oversupply in severe cases can cause coma and death. Mineral drinking water may also supply magnesium in fair quantities.21 The standard method for the determination of Mg2+ in drinking water is EDTA complexometric titration.22 Usually, earlier designs for Mg2+ fluorescent sensors were based on several metal chelating structures that have high affinity toward Mg2+; these sensors include β-diketone,23 crown ether,24 polyether,25 calix[4]arene,26 etc. However, these synthetic fluorescent sensors require time-consuming and synthetic difficulties with limited sensitivity or selectivity.
Naphthalene is extensively employed as molecular probes for the study of complex biological systems because of its short fluorescence life-time, low fluorescence quantum yield, and ability to act as a donor as well as an acceptor.27 On the other hand, pyrazole derivatives are well known five membered heterocycles with a broad spectrum of capable biological activity, namely, as anticancer agents.28 Furthermore, pyrazoles are recognized as important motifs to be used as ligands in coordination chemistry, as building blocks in heterocyclic synthesis, as optical brighteners, and UV stabilizers, and also as units to build supramolecular and photoinduced electron transfer systems.29 So far, much effort has been devoted for the designing of various chemosensors specific for both Zn2+ and Mg2+ detection.30 A huge number of fluorescent chemosensors for zinc or magnesium ions that utilize metal–ligand coordination have been developed because of their high sensitivity and simple operation.31 However, there are small number of fluorescent chemosensors reported so far that can selectively sense both of them.
Herein, we present the design, synthesis, spectral properties, and cell imaging studies of PYN, a new “turn-on” single fluorescent probe that allows Zn2+ and Mg2+ species in a semi-aqueous system. However, in the presence of these metal ions (Zn2+ and Mg2+) the PET32 process is restricted and fluorescence intensity is greatly enhanced due to the formation of a rigid chelated complex, causing the chelation-enhanced fluorescence effect (CHEF).33 According to this significant fluorescence signal change, PYN could detect Zn2+ and Mg2+ sensitively, selectively and quantitatively with a wide detection range, a low detection limit (2.2 × 10−7 M and 3.9 × 10−7 M for Zn2+ and Mg2+ respectively). Furthermore, the obtained probe PYN can be used to detect the Zn2+ and Mg2+ ions in cells by bioimaging. To the best of our knowledge, such type of unique chemosensor is rare in literature.
Experimental section
General
Chemicals and solvents were purchased from Sigma-Aldrich Chemicals Private Limited and were used without further purification. The metal salts used in the present investigation are as follows: Zn(ClO4)2·(H2O)6, CrCl3·(H2O)6, Cu(ClO4)2·(H2O)6, Fe(ClO4)2·(H2O)6, Ni(ClO4)2·(H2O)6, Co(ClO4)2·(H2O)6, NaClO4·H2O, KClO4·H2O, Ca(ClO4)2·4H2O, Pb(ClO4)2·3H2O, HgCl2, Cd(ClO4)2·6H2O, Mg(ClO4)2·6H2O, Al2(SO4)3·16H2O, Mn(ClO4)2·6H2O, GaCl3, InCl3 etc. The absorption and emission spectra were recorded on Hitachi UV-Vis U-3501 spectrophotometer and Perkin-Elmer LS55 fluorimeter, respectively. In all measurements the sample concentration was maintained at 2 × 10−5 M in order to avoid aggregation and reabsorption effects. All NMR spectra were recorded with TMS as internal standard on Bruker, AV 300/500 Supercon Digital NMR system. Mass spectroscopic analysis of the ligand was performed in a QTOF Micro YA263 ESI-TOF mass spectrometer using methanol as solvent. Fluorescence lifetimes were measured by the method of Time Correlated Single-Photon Counting (TCSPC) using a HORIBA Jobin Yvon Fluorocube-01-NL fluorescence lifetime spectrometer. The sample was excited using a laser diode at 375 nm and the signals were collected at the magic angle of 54.7° to eliminate any considerable contribution from fluorescence anisotropy decay.34 The typical time resolution of our experimental setup is ∼100 ps. The decays were deconvoluted using DAS-6 decay analysis software. The acceptability of the fits was judged by χ2 criteria and visual inspection of the residuals of the fitted function to the data. Mean (average) fluorescence lifetimes were calculated using the following equation:35 |
 | (1) |
in which αi is the pre-exponential factor corresponding to the ith decay time constant, τi.
Caution: Although no problems were encountered during the preparation of perchlorate salts, suitable care should be taken when handling such potentially hazardous compounds.
Synthesis
5-Methyl-1H-pyrazole-3-carboxylic acid hydrazide (C) was prepared following an established method.36 5-Methyl-1H-pyrazole-3-carboxylic acid hydrazide, C (1 g, 7.13 mmol) in 20 mL methanol was added dropwise to the methanolic solution (5 mL) of 6-methoxy-naphthalene-2-carbaldehyde (1.32 g, 7.13 mmol) with constant stirring. The resulting solution was refluxed for 5 hours and cooled to room temperature. Excess methanol was removed by rotary evaporator to have a colourless high viscous liquid. It was then kept in ice bath. After a while, the liquid froze to a microcrystalline white solid. The solid was filtered off, washed thoroughly with cold methanol and dried in vacuo over fused CaCl2. Yield (1.45 g, 65.9%). Mp > 250 °C (decomp.). 1H NMR (500 MHz, DMSO-d6): δ (ppm), 2.31 (s, 3H, –CH3), 3.91 (s, 3H, –O![[C with combining low line]](https://www.rsc.org/images/entities/char_0043_0332.gif)
![[H with combining low line]](https://www.rsc.org/images/entities/char_0048_0332.gif)
), 6.53 (s, 1H, Ar–H), 7.23 (t, 1H, Ar–H, J = 5 Hz), 7.38 (d, 1H, Ar–H, J = 2.2 Hz), 7.92–7.87 (m, 3H, Ar–H), 8.00 (s, 1H, ![[C with combining low line]](https://www.rsc.org/images/entities/char_0043_0332.gif)
N), 8.61 (s, 1H, py), 11.61 (s, 1H, ring py ![[N with combining low line]](https://www.rsc.org/images/entities/char_004e_0332.gif)
), 13.09 (s, 1H, –CO![[N with combining low line]](https://www.rsc.org/images/entities/char_004e_0332.gif)
); 13C NMR (300 MHz, DMSO-d6): δ (ppm), 10.76, 30.97, 40.83, 55.57, 79.63, 105.18, 105.57, 119.38, 123.82, 127.57, 128.57, 130.45, 135.61, 147.88, 158.57, 206.36; ESI-MS: m/z calculated for C17H16N4O2 [M + H]+ 309.13, found 309.16; anal. calc. for C17H16N4O2: C, 66.34; H, 5.28; N, 18.18; found: C, 66.22; H, 5.23; N, 18.17.
Synthesis of the PYN-Zn2+ complex
Zn(ClO4)2·6H2O (0.24 g, 0.6486 mmol) was dissolved in 5 mL of ACN and to this solution PYN (0.2 g, 0.6486 mmol) was added. After three hours of stirring at room temperature, the solution was dried using rotary evaporator which yielded a white PYN-Zn2+ complex. The resulting complex (PYN-Zn2+) is purified by recrystallization and characterized by 1H NMR, 13C NMR, FT-IR, TOF-MS (Fig. S19, S21, S23 and S8, ESI†).
Synthesis of the PYN-Mg2+ complex
Mg(ClO4)2·6H2O (0.21 g, 0.6486 mmol) was dissolved in 5 mL of ACN and to this solution, PYN (0.2 g, 0.6486 mmol) was added. After three hours of stirring at room temperature, the solution was dried using rotary evaporator which yielded a white PYN-Mg2+ complex. The resulting complex (PYN-Mg2+) is purified by recrystallization and characterized by 1H NMR, 13C NMR, FT-IR, TOF-MS (Fig. S20, S22, S24 and S9, ESI†).
Determination of the fluorescence quantum yield
The fluorescence quantum yields were determined using anthracene as a reference with a known ΦR value of 0.27 in EtOH.37 The area of the emission spectrum is integrated using the software available in the instrument and the quantum yield is calculated according to the following equation:38 |
ΦS/ΦR = [AS/AR] × [(Abs)R/(Abs)S] × [ηS2/ηR2]
| (2) |
where ΦS and ΦR are the fluorescence quantum yield of the sample and reference, respectively; AS and AR are the area under the fluorescence spectra of the sample and the reference, respectively; (Abs)S and (Abs)R are the corresponding optical densities of the sample and the reference solution at the wavelength of excitation; ηS and ηR are the refractive index of the sample and the reference, respectively.
Cyclic voltammetry
The cyclic voltammetric measurements were performed with a Sycopel model AEW21820F/L instrument. The experiments were carried out in a standard three-component cell equipped with a glassy carbon working electrode, a Pt wire as an auxiliary electrode and Ag/AgCl reference electrode. The cyclic voltammograms were acquired in a N2-bubbled acetonitrile solution containing ∼0.2 M tetraethylammonium perchlorate (TEAP) as supporting electrolyte. The scan speed was 100 mV s−1.
Calculation for detection limit
The detection limit (DL)39 of PYN for Zn2+/Mg2+ was determined using the following equation:where Sb is the standard deviation of the blank solution; S is the slope of the calibration curve.
Cell culture
Human gastric adenocarcinoma (AGS) cells were purchased from National Center for Cell Science, Pune, India, and used throughout the experiments. Cells were cultured in DMEM (Gibco BRL) supplemented with 10% FBS (Himedia), and antibiotic mixture (1%) containing PSN (Himedia) at 37 °C in a humidified incubator with 5% CO2.
Cell imaging
For imaging experiments, cells were grown in 6-well plates at 70–80% confluence. Cells were then incubated in DMEM containing different concentrations (500 nM, 1 μM, 10 μM) of PYN for 30 min at 37 °C. Cells were then washed with PBS followed by the addition of 10 μM Zn2+ and Mg2+ ions and incubation for 10 min. Bright field and fluorescence images were captured at 20× magnification by a fluorescence microscope (Olympus, 1 × 70) using Camedia software (Chicago, MI, USA) (E20P 5.0 Megapixel) and processed using Adobe Photoshop version 10.0. Also fluorescent intensity was monitored after the addition of TPEN (50 μM in the incubation media).
Cell cytotoxicity assay
To assess the cytotoxicity of the PYN, 3-(4,5-dimethylthiazol-2-yl)-2,S-diphenyltetrazolium bromide (MTT) assay was performed with AGS cells as per the procedure described earlier.40 After treatment of overnight culture of AGS cells (103 cells in each well of 96-well plate) with PYN (0.5, 1, 10 and 100 μM) for 12 h, 10 μL of a MTT solution (1 mg mL−1 in PBS) was added in each well and incubated at 37 °C for 3 h. Culture media were then removed and 100 μL of acidic isopropyl alcohol was added into each well. The intracellular formazan crystals (blue-violet) formed were solubilized with 0.04 N acidic isopropyl alcohol and absorbance of the solution was measured at 595 nm wavelength with a microplate reader (Model: THERMO MULTI SCAN EX). The cell viability was expressed as the optical density ratio of the treatment to control.
Results and discussions
The synthesis and characterization of the compound (C) has been published earlier.36 The target compound PYN was easily synthesized by simple Schiff base condensation of 5-methyl-1H-pyrazole-3-carboxylic acid hydrazide (C) with 6-methoxy-naphthalene-2-carbaldehyde (Scheme 1). The structure of compound PYN was characterized by 1H NMR, 13C NMR, FT-IR, TOF-MS and CHN elemental analyses (Fig. S1–S4, ESI†).
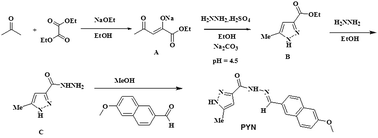 |
| Scheme 1 Synthesis of the probe PYN. | |
With an objective to evaluate the potential use of the probe PYN as a chemosensor, we investigated its interaction with various metal ions using perchlorate as a counter anion under UV-vis and fluorescence measurements. Among the competitive metal ions, such as Na+, K+, Ca2+, Mg2+, Pb2+, Al3+, Cr3+, Mn2+, Fe2+, Fe3+, Co2+, Ni2+, Cu2+, Ga3+, In3+, Zn2+, Cd2+ and Hg2+, tested in 7
:
3 (v/v) aqueous acetonitrile solution (10 mM Tris–HCl, pH 7.2 at 25 °C), only Zn2+ and Mg2+ respond to the probe PYN in the ground and excited states. The probe PYN (5 μM) itself exhibited three absorption bands at 325 nm, 274 nm and 282 nm. The absorbance of PYN (5 μM) at 325 nm, 274 nm and 282 nm gradually decreases and a new absorption band appears at 375 nm with addition of increasing concentrations of Zn2+ (Fig. S5, ESI†). In contrast, titration of PYN (5 μM) with concomitant addition of Mg2+ resulted in increase in the absorption bands at 325 nm, 274 nm and 282 nm whereas simultaneously a new band appeared at 253 nm with increased in intensity (Fig. S6, ESI†). However, no such significant change in PYN spectrum was observed with above mentioned metal cations (Fig. S7, ESI†).
The host–guest recognition abilities of PYN with Zn2+ and Mg2+ ions further investigated via a fluorescence spectroscopic method. The excitation wavelength was selected at 370 nm. No change in fluorescence was observed with various groups 1, 2 and transition metals, while exposure to Zn2+ or Mg2+ produced a large increase in fluorescence for the probe PYN (Fig. 1).
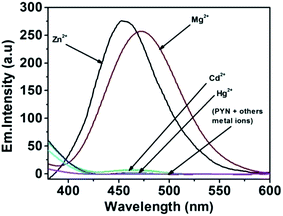 |
| Fig. 1 Fluorescence spectra (excitation at 370 nm) of the probe PYN (5 μM) in 7 : 3 (v/v) MeCN–water solution (10 mM Tris–HCl, pH 7.2 at 25 °C), by addition of 1.6 equiv. different metal ions (Na+, K+, Ca2+, Mg2+, Pb2+, Al3+, Cr3+, Mn2+, Fe2+, Fe3+, Co2+, Ni2+, Cu2+, Ga3+, In3+, Zn2+, Cd2+, Hg2+). | |
Under physiological conditions, the probe PYN (5 μM) exhibited maximum emission around 436 nm with very low quantum yields of 0.003. However, upon gradual addition of Zn2+ or Mg2+ resulted in a remarkably enhanced fluorescence at 456 nm (sky blue emission for Zn2+) or 472 nm (blue emission for Mg2+) with increasing concentration (0–1.6 equiv.), of the metal ions (Fig. 2 and 3). The plots of emission intensity of PYN as a function of added Zn2+ and Mg2+ are presented in inset Fig. 2 and 3 respectively. Therefore, the probe PYN's fluorogenic sensing ability could be observed by the naked eye in the presence of Zn2+ or Mg2+ with the enhancement of a clearly observed sky blue and blue emission respectively when illuminated with 254 nm UV-light (inset Fig. 4). The red shift of the emission band is accompanied by 49-fold fluorescence enhancement for Zn2+ (Φ, 0.15). Similarly 41-fold fluorescence enhancement (Φ, 0.12) is observed for Mg2+. Relative fluorescence enhancement of PYN in the absence and presence of various other metal ions was shown in Fig. 4.
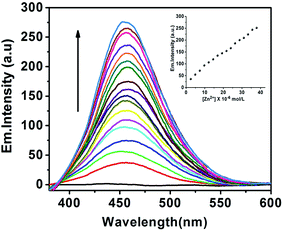 |
| Fig. 2 Emission spectra of the probe PYN (5 μM) in the presence of increasing concentrations of 1.6 equiv. of Zn2+ in 7 : 3 (v/v) MeCN–water solution (10 mM Tris–HCl, pH 7.2 at 25 °C). Excitation was performed at 370 nm. Inset: fluorescence intensity at 456 nm as a function of Zn2+ concentration. | |
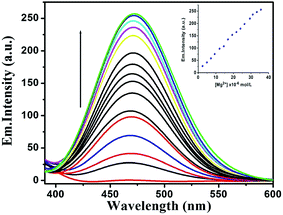 |
| Fig. 3 Emission spectra of the probe PYN (5 μM) in the presence of increasing concentrations of Mg2+ in 7 : 3 (v/v) MeCN–water solution (10 mM Tris–HCl, pH 7.2 at 25 °C). Excitation was performed at 370 nm. Inset: fluorescence intensity at 472 nm as a function of Mg2+ concentration. | |
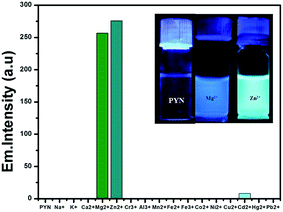 |
| Fig. 4 Bar graph shows the relative emission intensity of the probe PYN (5 μM) upon treatment with various metal ions in 7 : 3 (v/v) MeCN–water solution (10 mM Tris–HCl, pH 7.2 at 25 °C). Inset: fluorescence change after the addition of Zn2+ and Mg2+ metal ions under UV lamp. | |
The reason for the addition of Zn2+ or Mg2+ to the solution PYN resulting in fluorescence enhancement is due to suppression of photoinduced electron transfer effect (PET) from the lone pair electron of the pyrazole used for metal (Zn2+/Mg2+) binding (Scheme 2).
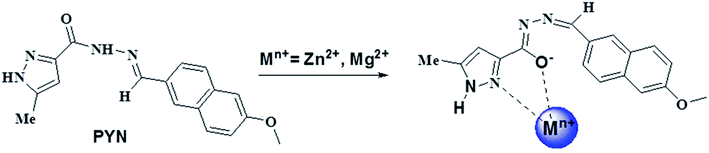 |
| Scheme 2 Proposed binding mode of PYN with Mn+ (n = Zn, Mg). | |
Additional support for the proposed binding process and PET mechanism between the pyrazole carbohydrazide and naphthalene moiety is thermodynamically feasible which is evident from the electrochemical data. PET is the most widely employed mechanism for the design of fluorescence probes. Classical PET probes for metal cations contain three parts: fluorophore, spacer and ionophore. Ionophores are normally electron donors (e.g. amino-containing group), while fluorophores are electron acceptors. In this paper pyrazole carbohydrazide is ionophores and naphthalene moiety is fluorophore. The reduction potential of the fluorophore, Ered (fluorophore) is −0.71 V (reversible), while the oxidation potential of the fluorophore is observed at around 1.08 V (reversible). With the pyrazole moiety, one irreversible oxidation wave was observed at 0.46 V (vs. Ag/AgCl). Therefore, the free energy change for the PET process34,41 (ΔGPET) is −46.06 kcal mol−1 in acetonitrile according to ΔGPET = 23.06 [Eox(recep) − Ered(fluoro)] − E0,0, where, Eox(recep) and Ered(fluoro) represent the oxidation potential of the receptor moiety and the reduction potential of the fluorophore, respectively, whereas E0,0 represents the energy of the fluorescent state. The value of E0,0 used in the calculation has been estimated from location of the first peak position in the fluorescence spectrum. Negative ΔG value dictates the thermodynamic feasibility of the PET process in the studied system.
To determine the stoichiometry, a Job's plot42 obtained from the emission data showed a 1
:
1 association of PYN with both Zn2+ and Mg2+ (Fig. 5 and 6). Furthermore, the result is consistent with the ESI-MS experiment of PYN with Zn2+ and Mg2+ (Fig. S8 and S9, ESI†). A peak at m/z 488.17 assigned to [PYN + Zn2+ + ClO4− + H2O − H+]+ is observed (calcd. 488.10). Also, the positive-ion mass spectrum of PYN upon addition of 1 equiv. of Mg2+ showed the formation of the [PYN + Mg2+ + ClO4− − H+]+ complex [m/z: 430.97; calcd, 430.05].
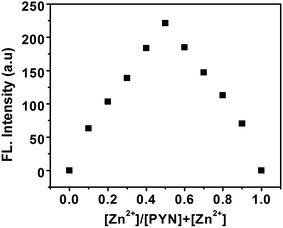 |
| Fig. 5 Job's plot for the binding of probe PYN and Zn2+, where the intensity at 456 nm was plotted against the mole fraction of Zn2+. | |
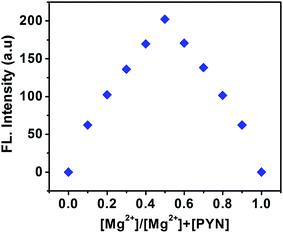 |
| Fig. 6 Job's plot for the binding of probe PYN and Mg2+, where the intensity at 472 nm was plotted against the mole fraction of Mg2+. | |
The binding constant (Ka)43 for PYN-Zn2+/Mg2+ complexes were calculated from the fluorescence titration by mathematical models such as Benesi–Hildebrand. We thus start with the equation:
|
1/(I − I0) = 1/{Ka(I∞ − I0)[Mn+]} + 1/(I∞ − I0)
| (4) |
in which
I0,
I and
I∞ are the emission intensities, respectively, in the absence of, at an intermediate and infinite concentration of the metal ion (M
n+).
A linear regression for the plot of 1/[I − I0] vs. 1/[Mn+] for Zn2+ and Mg2+ ions dictates a 1
:
1 association between PYN and each of the two metal ions. Assuming a binding interaction between the two partners, the strength of binding can be quantitatively evaluated in terms of binding constant as Ka = intercept to slope ratio of the Benesi–Hildebrand plot = 7.01 × 106 M−1 for Zn2+ and 3.23 × 104 M−1 for Mg2+ (Fig. S10 and S11, ESI†). Consequently the free energy change for the process of complexation reaction is calculated to be ΔG = −RT
ln
Ka = −39.02 kJ mol−1 for Zn2+ and −25.52 kJ mol−1 for Mg2+, i.e. a negative free energy change which dictates the thermodynamic feasibility for the occurrence of the binding interaction. Also a relatively stronger binding of PYN with Zn2+ ion is manifested through a higher binding constant value as compared with Mg2+ ion. Stronger binding implies higher rigidity of PYN-Zn2+ complex than PYN-Mg2+ complex which causes the inhibition of different non-radiative decay processes (PET) resulting in enhancement of fluorescence.
For a probe to be extensively used in the detection of specific targets, reversibility is an important issue. To examine whether the sensing process of PYN is reversible, 1 equiv. of a sodium salt of ethylenediaminetetraacetic acid (EDTA) solution was added into the solution of PYN, which was preincubated with 1 equiv. of a Zn2+/Mg2+ solution. After the addition of an EDTA solution, the initial emission intensity of PYN was almost recovered immediately from PYN-Zn2+ and PYN-Mg2+ complexes (Fig. S12 and S13, ESI†). It indicates the decomplexation of PYN-Zn2+ and PYN-Mg2+ as EDTA strips away Zn2+ and Mg2+ from the binding zone.
For practical purposes, the detection limit of probe PYN for the analysis of Zn2+ and Mg2+ ions were also an important parameter. We have determined the standard deviation (Sb) of the blank solution (Fig. S14, ESI†). In addition, the fluorescence titration profile also demonstrates that PYN has a detection limit of 2.2 × 10−7 M for Zn2+ and 3.9 × 10−7 M for Mg2+ ions (Fig. S15 and S16, ESI†), which are comparable to the other reported Zn2+ and Mg2+ chemosensors.44
The interference of other competing metal ions was investigated by the competition experiment which was conducted with Zn2+ and Mg2+ in the presence of other competing ions (Fig. S17 and S18, ESI†). The fluorescence intensity of the complex was hardly affected by other coexistent metal ions. These results indicated that PYN could be used as an efficient and selective fluorescence probe for Zn2+ and Mg2+ in the presence of a wide range of other competing cations.
Based on the fluorimetric experiments, the affinity of PYN with Zn2+ is much stronger than that with Mg2+, when Zn2+ was added to the solution of PYN-Mg2+ ensemble, Mg2+ was displaced by Zn2+, resulting in a significant decrease emission intensity at 472 nm and an increase of a blue-shifted emission band centered at 456 nm (attributed to the formation of a PYN-Zn2+ complex) (Fig. 7). This suggests that [PYN-Mg2+] can be used as a second optical response for sensing Zn2+. However, the addition of increasing concentrations of Mg2+ to PYN-Zn2+, no response was observed (Fig. 8). These results revealed that PYN can be employed as a dual-mode Zn2+ sensor via the competitive metal ion displacement approach.
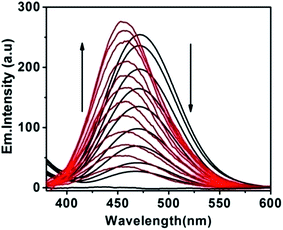 |
| Fig. 7 Fluorescence spectra of PYN-Mg2+ upon addition of Zn2+ in 7 : 3 (v/v) MeCN–water solution (10 mM Tris–HCl, pH 7.2 at 25 °C). | |
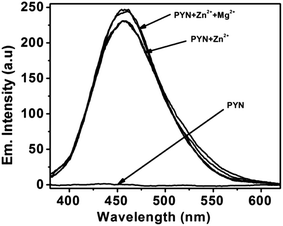 |
| Fig. 8 Fluorescence spectra of PYN-Zn2+ upon addition of Mg2+ in 7 : 3 (v/v) MeCN–water solution (10 mM Tris–HCl, pH 7.2 at 25 °C). | |
To evaluate the binding of metal ion to the probe PYN, 1H NMR titration experiments in DMSO-d6 are carried out (Fig. 9). 1H NMR titration experiment of PYN shows that the proton at δ 12.94 ppm corresponding to –CO![[N with combining low line]](https://www.rsc.org/images/entities/char_004e_0332.gif)
peak of the probe PYN disappears on adding Zn2+/Mg2+. The signal at δ 8.42 ppm corresponding to azomethine proton (C) is slightly shifted to upfield. If metals bind to the immine nitrogen then a downfield shift is expected. Now slight upfield shift of other protons (b, d and aromatic hydrogens) may be explained in terms of deprotonation of the amide proton which leads to increase in electron density over the probe and as a consequence upfield shift is observed.
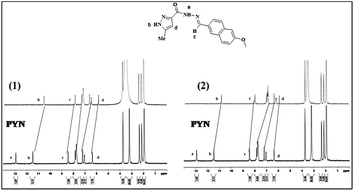 |
| Fig. 9 The 1H NMR spectra of PYN upon the addition of 1 equiv. of (1) Zn2+ and (2) Mg2+ in DMSO-d6. | |
Further, IR spectra of probe PYN with Zn2+/Mg2+ ion also confirms the proposed mechanism (Fig. S23 and S24, ESI†) as in both cases the immine peaks remains almost unaffected. Therefore, these findings clearly support that the complexation occurs between the amide carbonyl oxygen with Zn2+/Mg2+ and pyrazole N atoms to the metal center.
Time-resolved fluorescence decay studies for PYN were carried out in the absence and presence of Zn2+ and Mg2+ ions using TCSPC technique (Fig. 10). A laser excitation source of 375 nm was used and decay luminescence kinetics was monitored at 465 nm. The fluorescence decay behavior of PYN further confirms PET-induced fluorescence quenching. The decay behaviour of the bare fluorophore (PYN) or its metal complex is found to be complicated and is best fitted to triexponential functions. The fluorescence decay curve of PYN consisted of a short-lived major component (∼0.06 ns, ∼93%) which clearly represents the lifetime of the PET-quenched fluorophore.34,35 In the presence of Zn2+ and Mg2+, rigidification of the framework upon binding of these two metal ions results in lowering the nonradiative decays. Therefore, average lifetime (τav) enhances significantly upon the addition of these two cations (Table 1, ESI†).
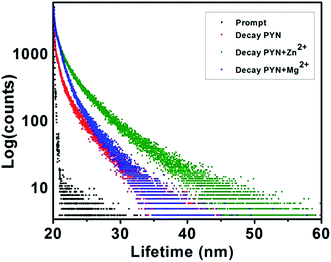 |
| Fig. 10 Time-resolved fluorescence decay of PYN (red), PYN-Zn2+ (olive green) and PYN-Mg2+ (blue). | |
To better realize the nature of the coordination of Zn2+/Mg2+ with PYN, energy-optimized structures of PYN and PYN-Zn2+/Mg2+ were obtained on Density Functional Theory (DFT) calculations at the B3LYP level using 6-311G** basis set for probe (PYN) and LANL2DZ basis set for metal complexes using the Gaussian 09 program.45 The DFT computed molecular structure of PYN and its PYN-Zn2+/Mg2+ complexes were shown in Fig. 11a. The energy gaps between the highest occupied molecular orbital (HOMO) and lowest unoccupied molecular orbital (LUMO) of the probe PYN and corresponding Zn2+ and Mg2+ complexes were found to be 3.95 eV, 3.23 eV and 3.38 eV respectively (Fig. 11b). These results exhibited that both the HOMO and LUMO of the PYN-Zn2+ complex was better stabilized than those of PYN and PYN-Mg2+ complex (Table 2, ESI†).
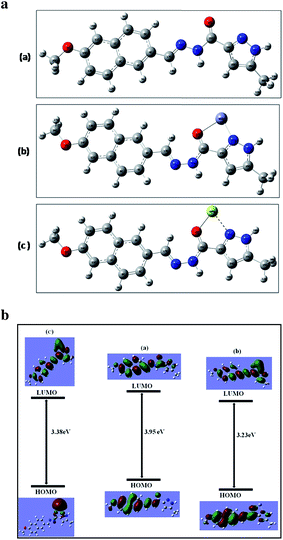 |
| Fig. 11 (a) Stereoscopic view of the energy optimized structures of (a) PYN (b) PYN-Zn2+ and (c) PYN-Mg2+. (b) HOMO–LUMO energy gaps for respective compounds and interfacial plots of the orbitals of (a) PYN (b) PYN-Zn2+ (c) PYN-Mg2+. | |
To investigate the potential biological application of the probe PYN in living cells, we performed intracellular Zn2+ and Mg2+ dual imaging of AGS cells by fluorescence microscopy. After incubation with DMSO-containing PYN (500 nM, DMSO
:
water = 1
:
99) for 30 min at 37 °C, cell could not show any recognizable fluorescence. However, addition of exogenous Zn2+ and Mg2+ ions (10 μM each) separately to the cells showed blue (Fig. 12a) and green fluorescence (Fig. 12b) respectively. These experiments indicate PYN can discriminate intracellularly between Zn2+ and Mg2+ with blue and green fluorescence, respectively. Moreover, with increasing concentration of probe PYN (500 nM, 1 μM & 10 μM) fluorescence intensity was enhanced concentration-dependently. The fluorescence signal of PYN in presence of Zn2+ and Mg2+ ions may be utilized as a signature of selective sensor response. Hence, these results indicate that the probe PYN is an efficient candidate for monitoring changes in the intracellular Zn2+ and Mg2+ ions concentration under biological conditions.
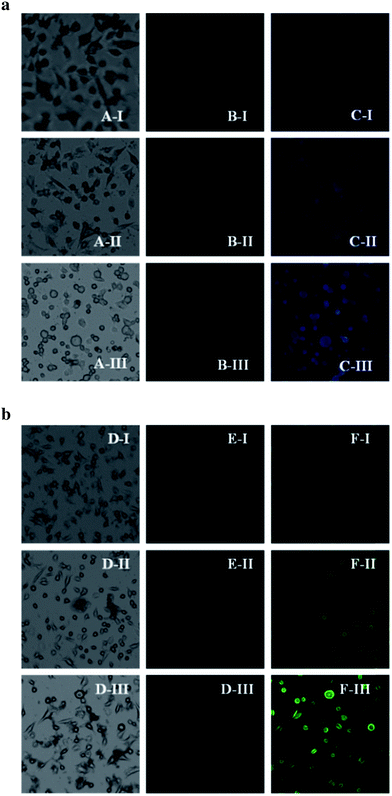 |
| Fig. 12 (a) (A-I), (A-II) & (A-III) are the bright field images of the probe PYN (500 nM, 1 μM & 10 μM) treated cells respectively. (B-I), (B-II) & (B-III) represent fluorescence images treated with different concentrations of the probe PYN, in absence of Zn2+ ions. (C–I), (C-II) & (C-III) indicate fluorescence images treated with different concentrations of probe PYN in addition of Zn2+ (10 μM) ions. The samples were excited at 375 nm. (b) (D-I), (D-II) & (D-III) are the bright field images of 500 nM, 1 μM & 10 μM probe PYN treated cells respectively. (E-I), (E-II) & (E-III) represent fluorescence images treated with different concentrations of probe PYN alone in absence of Mg2+ ions. (F-I), (F-II) & (F-III) indicate fluorescence images treated with different concentrations of probe PYN in addition of Mg2+ (10 μM) ions. For all imaging, the samples were excited at 375 nm. | |
The MTT assay results confirm that PYN has no significant cytotoxic effects on adenocarcinoma cells (AGS) up to 12 h of treatment (Fig. 13). Thus the PYN has promising potential in dual sensing of Zn2+ and Mg2+ in vitro.
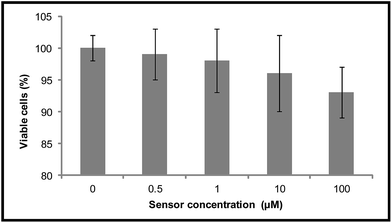 |
| Fig. 13 % cell viability of AGS cells treated with different concentrations (0.5–100 μM) of PYN for 12 h determined by MTT assay. Results are expressed as mean ± S.D of three independent experiments. | |
Conclusions
In conclusion, we have successfully developed a low cost and easily synthesize Schiff-base probe PYN for the selective detection of Zn2+ and Mg2+ showing ‘turn-on’ fluorescent response without any interference from other tested metal ions. The probe followed a turn-on mechanism with the inhibition of PET and the activation of CHEF. Moreover, Zn2+ displaces Mg2+ from the PYN-Mg2+ complex, demonstrating that a dual-mode PYN sensor can be employed to detect Zn2+ through two distinct processes based on the CHEF and competitive metal ion displacement approaches. The proposed fluorescence probe has a low sensing limit as well as a high selectivity towards Zn2+ (2.2 × 10−7 M) and Mg2+ (3.9 × 10−7 M). Furthermore, the probe PYN is found to be efficient in reporting the presence of both Zn2+ and Mg2+ ions inside the cell without showing any cytotoxicity.
Acknowledgements
A. D. acknowledges the financial support provided by University Grants Commission, India (Award letter No. F.4-2/2006(BSR)/CH/14-15/0163, dated May, 2015) through the Dr. D. S. Kothari Post Doctoral Fellowship (DSKPDF).
Notes and references
-
(a) Fluorescent Chemosensors for Ions and Molecule Recognition, ed. A. W. Czarnik, American Chemical Society, Washington, D. C., 1992 Search PubMed;
(b) A. P. de Silva, D. B. Fox, A. J. M. Huxley and T. S. Moody, Coord. Chem. Rev., 2000, 205, 41–57 CrossRef CAS;
(c) X. He, H. Liu, Y. Li, S. Wang, Y. Li, N. Wang, J. Xiao, X. Xu and D. Zhu, Adv. Mater., 2005, 17, 2811–2815 CrossRef CAS;
(d) D. T. Quang and J. S. Kim, Chem. Rev., 2007, 107, 3780–3799 CrossRef PubMed;
(e) A. W. Czarnik, Acc. Chem. Res., 1994, 27, 302–308 CrossRef CAS;
(f) A. P. de Silva, H. Q. N. Gunaratne, T. Gunnlaugsson, A. J. M. Huxley, C. P. McCoy, J. T. Rademacher and T. E. Rice, Chem. Rev., 1997, 97, 1515–1566 CrossRef CAS PubMed.
-
(a) L. Xue, Q. Liu and H. Jiang, Org. Lett., 2009, 11, 3454–3457 CrossRef CAS PubMed;
(b) H. Komatsu, T. Miki, D. Citterio, T. Kubota, Y. Shindo, Y. Kitamura, K. Oka and K. Suzuki, J. Am. Chem. Soc., 2005, 127, 10798–10799 CrossRef CAS PubMed;
(c) M. Yuan, W. Zhou, X. Liu, M. Zhu, J. Li, X. Yin, H. Zheng, Z. Zuo, C. Ouyang, H. Liu, Y. Li and D. Zhu, J. Org. Chem., 2008, 73, 5008–5014 CrossRef CAS PubMed;
(d) M. Dong, Y.-W. Wang and Y. Peng, Org. Lett., 2010, 12, 5310–5313 CrossRef CAS PubMed;
(e) P. N. Basa and A. G. Sykes, J. Org. Chem., 2012, 77, 8428–8434 CrossRef CAS PubMed.
- J. Y. Jung, S. J. Han, J. Chun, C. Lee and J. Yoon, Dyes Pigm., 2012, 94, 423–426 CrossRef CAS.
- J. Mao, L. Wang, W. Dou, X. Tang, Y. Yan and W. Liu, Org. Lett., 2007, 9, 4567–4570 CrossRef CAS PubMed.
- N. Singh, N. Kaur, C. N. Choitir and J. F. Callan, Tetrahedron Lett., 2009, 50, 4201–4204 CrossRef CAS.
-
(a) S. Sen, S. Sarkar, b. Chattopadhyay, A. Moirangthaem, A. Basu, K. Dhara and P. Chattopadhyay, Analyst, 2012, 137, 3335–3342 RSC;
(b) Q. Mei, C. Jiang, G. Guan, K. Zhang, B. Liu, R. Liu and Z. Zhang, Chem. Commun., 2012, 48, 7468–7470 RSC.
- D. Y. Lee, N. Singh and D. O. Jang, Tetrahedron Lett., 2011, 52, 3886–3890 CrossRef CAS.
- S. Wang, G. Men, L. Zhao, Q. Hou and S. Jiang, Sens. Actuators, B, 2010, 145, 826–831 CrossRef CAS.
- L. Tang, F. Li, M. Liu and R. Nandhakumar, Spectrochim. Acta, Part A, 2011, 78, 1168–1172 CrossRef PubMed.
-
(a) D. Maity and T. Govindaraju, Chem. Commun., 2012, 48, 1039–1041 RSC;
(b) R. Alam, T. Mistri, R. Bhowmick, A. Katarkar, K. Chaudhuri and M. Ali, RSC Adv., 2016, 6, 1268–1278 RSC.
-
(a) P. J. Fraker and L. E. King, Annu. Rev. Nutr., 2004, 24, 277–298 CrossRef CAS PubMed;
(b) K. Jobe, C. H. Brennan, M. Motevalli, S. M. Goldup and M. Watkinson, Chem. Commun., 2011, 47, 6036–6038 RSC;
(c) R. Bhowmick, R. Alam, T. Mistri, K. K. Das, A. Katarkar, K. Chaudhuri and M. Ali, RSC Adv., 2016, 6, 11388 RSC.
-
(a) M. P. Cuajungco and G. J. Lees, Neurobiol. Dis., 1997, 4, 137 CrossRef CAS PubMed;
(b) A. I. Bush, W. H. Pettingell, G. Multhaup, M. D. Paradis, J. P. Vonsattel, J. F. Gusella, K. Beyreuther, C. L. Masters and R. E. Tanzi, Science, 1994, 265, 1464–1467 CAS;
(c) J.-Y. Koh, S. W. Suh, B. J. Gwag, Y. Y. He, C. Y. Hsu and D. W. Choi, Science, 1996, 272, 1013–1016 CAS.
-
(a) A. Q. Truong-Tran, J. Carter, R. E. Ruffin and P. D. Zalewski, BioMetals, 2001, 14, 315–330 CrossRef CAS PubMed;
(b) P. D. Zalewski, I. J. Forbes, R. F. Seamark, R. Borlinghaus, W. H. Betts, S. F. Lincoln and A. D. Ward, Chem. Biol., 1994, 1, 153–161 CrossRef CAS PubMed;
(c) E. Kimura, S. Aoki, E. Kikuta and T. Koike, Proc. Natl. Acad. Sci. U. S. A., 2003, 100, 3731–3736 CrossRef CAS PubMed.
-
(a) J. P. Jiang and Z. J. Guo, Coord. Chem. Rev., 2004, 248, 205–229 CrossRef;
(b) E. M. Nolan and S. J. Lippard, Acc. Chem. Res., 2009, 42, 193–203 CrossRef CAS PubMed;
(c) Z. C. Xu, J. Y. Yoon and D. R. Spring, Chem. Soc. Rev., 2010, 39, 1996–2006 RSC;
(d) C. C. Zhao, Y. L. Zhang, P. Feng and J. Cao, Dalton Trans., 2012, 41, 831–838 RSC;
(e) J. Cao, C. C. Zhao, X. Z. Wang, Y. F. Zhang and W. H. Zhu, Chem. Commun., 2012, 48, 9897–9899 RSC;
(f) Y. Mikata, S. Takeuchi, H. Konno, S. Iwatsuki, S. Akaji, I. Hamagami, M. Aoyama, K. Yasuda, S. Tamotsu and S. C. Burdette, Dalton Trans., 2014, 43, 10013–10022 RSC;
(g) L. Y. Zhao, Q. L. Mi, G. K. Wang, J. H. Chen, J. F. Zhang, Q. H. Zhao and Y. Zhou, Tetrahedron Lett., 2013, 54, 3353–3358 CrossRef CAS;
(h) A. Ajayaghosh, P. Carol and S. Sreejith, J. Am. Chem. Soc., 2005, 127, 14962–14963 CrossRef CAS PubMed;
(i) L. Fan, J.-c. Qin, T.-r. Li, B.-d. Wang and Z.-y. Yang, Sens. Actuators, B, 2014, 203, 550–556 CrossRef CAS;
(j) Z. Kowser, H. Tomiyasu, X. Jiang, U. Rayhan, C. Redshaw and T. Yamato, New J. Chem., 2015, 39, 4055–4062 RSC;
(k) B. Zhang, K.-S. Cao, Z.-A. Xu, Z.-Q. Yang, H.-W. Chen, W. Huang, G. Yin and X.-Z. You, Eur. J. Inorg. Chem., 2012, 2012, 3844–3851 CrossRef CAS;
(l) K. Komatsu, Y. Urano, H. Kojima and T. Nagano, J. Am. Chem. Soc., 2007, 129, 13447–13454 CrossRef CAS PubMed.
-
(a) N. C. Lim, H. C. Freake and C. Brückner, Chem.–Eur. J., 2005, 11, 38–49 CrossRef PubMed;
(b) Z. C. Xu, J. Y. Yoon and D. R. Spring, Chem. Soc. Rev., 2010, 39, 1996–2006 RSC;
(c) H. G. Lee, J. H. Lee, S. P. Jang, H. M. Park, S. Kim, Y. Kim, C. Kim and R. G. Harrison, Tetrahedron, 2011, 67, 8073–8078 CrossRef CAS;
(d) E. Kimura and T. Koike, Chem. Soc. Rev., 1998, 27, 179–184 RSC.
-
(a) Z. Xu, J. Yoon and D. R. Spring, Chem. Soc. Rev., 2010, 39, 1996–2006 RSC;
(b) Y. Xu, J. Meng, L. Meng, Y. Dong, Y. Cheng and C. Zhu, Chem.–Eur. J., 2010, 16, 12898–12903 CrossRef CAS PubMed;
(c) X. Liu, N. Zhang, J. Zhou, T. Chang, C. Fang and D. Shangguan, Analyst, 2013, 138, 901–906 RSC;
(d) Y. Mikata, A. Yamashita, K. Kawata, H. Konno, S. Itami, K. Yasuda and S. Tamotsu, Dalton Trans., 2012, 41, 4976–4981 RSC.
- R. J. Elin, Clin. Chem., 1994, 102, 616–622 CAS.
- J. Durlach, Le Magnesium en Pratique Clinique, Bailiere JB, EM Inter, Paris, 1985 Search PubMed.
- V. K. Gupta, R. Prasad and A. Kumar, Talanta, 2004, 63, 1027–1033 CrossRef CAS PubMed.
- V. K. Gupta, S. Chandra and R. Mangla, Sens. Actuators, B, 2002, 86, 235–241 CrossRef CAS.
-
(a) M. Rosenlund, N. Berglind, J. Hallqvist, T. Bellander and G. Bluhm, Epidemiology, 2005, 16, 570–576 CrossRef PubMed;
(b) A. Rosanoff, Med. Hypotheses, 2013, 81, 1063–1065 CrossRef CAS PubMed.
- T. M. Lerga and C. K. O'Sullivan, Anal. Chim. Acta, 2008, 610, 105–111 CrossRef CAS PubMed.
- R. Sedano and J. C. Rodríguez-Ubis, Tetrahedron Lett., 1997, 38, 4459–4462 CrossRef.
- J. Brandel, M. Sairenji, K. Ichikawa and T. Nabeshima, Chem. Commun., 2010, 46, 3958–3960 RSC.
- J. Kim, T. Morozumi and H. Nakamura, Org. Lett., 2007, 9, 4419–4422 CrossRef CAS PubMed.
- A. Hamdi, S. H. Kim, R. Abidi, P. Thuéry, J. S. Kim and J. Vicens, Tetrahedron, 2009, 65, 2818–2823 CrossRef CAS.
-
(a) D. P. Roek, J. E. Chateauneuf and J. F. Brennecke, Ind. Eng. Chem. Res., 2000, 39, 3090–3096 CrossRef CAS;
(b) P. Frederick and S. P. Schwarz Wasik, Anal. Chem., 1976, 48, 524–528 CrossRef;
(c) M. Shortreed, R. Kopelman, M. Kuhn and B. Hoyland, Anal. Chem., 1996, 68, 1414–1418 CrossRef CAS PubMed;
(d) A. Caballero, R. Martinez, V. Lloveras, I. Ratera, J. Vidal-Gancedo, K. Wurst, A. Tárraga, P. Molina and J. Veciana, J. Am.
Chem. Soc., 2005, 127, 15666–15667 CAS.
- J. Elguero, in Comprehensive Heterocyclic Chemistry, ed. A. R. Katritzky, C. W. Rees and E. F. V. Scriven, Pergamon, Oxford, 1996, vol. 5 Search PubMed.
- B. Willy and T. J. Müller, Eur. J. Org. Chem., 2008, 4157–4168 CrossRef CAS.
- R. Alam, T. Mistri, A. Katarkar, K. Chaudhuri, S. K. Mandal, A. R. Khuda-Bukhsh, K. K. Das and M. Ali, Analyst, 2014, 139, 4022–4030 RSC.
-
(a) T. Mistri, M. Dolai, D. Chakraborty, A. R. Khuda-Bukhsh, K. K. Das and M. Ali, Org. Biomol. Chem., 2012, 10, 2380–2384 RSC;
(b) G. Men, C. Chen, S. Zhang, C. Liang, Y. Wang, M. Deng, H. Shang, B. Yang and S. Jiang, Dalton Trans., 2015, 44, 2755–2762 RSC.
-
(a) T. Mistri, R. Alam, M. Dolai, S. K. Mandal, A. R. Khuda-Bukhsh and M. Ali, Org. Biomol. Chem., 2013, 11, 1563–1569 RSC;
(b) R. Alam, T. Mistri, P. Mondal, D. Das, S. K. Mandal, A. R. Khuda-Bukhsh and M. Ali, Dalton Trans., 2014, 43, 2566–2576 RSC.
-
(a) A. Dhara, A. Jana, N. Guchhait, P. Ghosh and S. K. Kar, New J. Chem., 2014, 38, 1627–1634 RSC;
(b) A. Dhara, A. Jana, N. Guchhait and S. K. Kar, J. Lumin., 2014, 154, 369–375 CrossRef CAS.
- J. R. Lakowicz, Principles of Fluorescence Spectroscopy, Plenum, New York, 1999 Search PubMed.
-
(a) S. Banthia and A. Samanta, J. Phys. Chem. B, 2006, 110, 6437–6440 CrossRef CAS PubMed;
(b) B. Ramachandram, G. Saroja, N. B. Sankaran and A. Samanta, J. Phys. Chem. B, 2000, 104, 11824–11832 CrossRef CAS.
- N. Saha and K. M. Dutta, J. Inorg. Nucl. Chem., 1981, 43, 1405–1410 CrossRef CAS.
- W. H. Melhuish, J. Phys. Chem., 1961, 65, 229–235 CrossRef CAS.
- E. Austin and M. Gouterman, Bioinorg. Chem., 1978, 9, 281–298 CrossRef CAS.
- G. L. Long and J. D. Winefordner, Anal. Chem., 1983, 55, 712A–724A CrossRef CAS.
- T. Mossman, J. Immunol. Methods, 1983, 65, 55–63 CrossRef.
-
(a) B. K. Paul, S. Kar and N. Guchhait, J. Photochem. Photobiol., A, 2011, 220, 153–163 CrossRef CAS;
(b) A. Ganguly, B. K. Paul, S. Ghosh, S. Kar and N. Guchhait, Analyst, 2013, 138, 6532–6541 RSC.
- W. C. Vosburgh and G. R. Copper, J. Am. Chem. Soc., 1941, 63, 437–442 CrossRef CAS.
- H. A. Benesi and J. H. Hildebrand, J. Am. Chem. Soc., 1949, 71, 2703–2707 CrossRef CAS.
-
(a) D. J. Eide, Biochim. Biophys. Acta, 2006, 1763, 711–722 CrossRef CAS PubMed;
(b) C. S. He, W. P. Zhu, Y. F. Xu, T. Chen and X. H. Qian, Anal. Chim. Acta, 2009, 651, 227–233 CrossRef CAS PubMed;
(c) H. M. N. H. Irving, H. Freiser and T. S. West, IUPAC Compendium of Analytical Nomenclature, Definitive Rules, Pergamon Press, Oxford, 1981 Search PubMed.
- M. J. Frisch, G. W. Trucks, H. B. Schlegel, G. E. Scuseria, M. A. Robb, J. R. Cheeseman, G. Scalmani, V. Barone, B. Mennucci, G. A. Petersson, H. Nakatsuji, M. Caricato, X. Li, H. P. Hratchian, A. F. Izmaylov, J. Bloino, G. Zheng, J. L. Sonnenberg, M. Hada, M. Ehara, K. Toyota, R. Fukuda, J. Hasegawa, M. Ishida, T. Nakajima, Y. Honda, O. Kitao, H. Nakai, T. Vreven, J. A. Montgomery Jr, J. E. Peralta, F. Ogliaro, M. Bearpark, J. J. Heyd, E. Brothers, K. N. Kudin, V. N. Staroverov, R. Kobayashi, J. Normand, K. Raghavachari, A. Rendell, J. C. Burant, S. S. Iyengar, J. Tomasi, M. Cossi, N. Rega, J. M. Millam, M. Klene, J. E. Knox, J. B. Cross, V. Bakken, C. Adamo, J. Jaramillo, R. Gomperts, R. E. Stratmann, O. Yazyev, A. J. Austin, R. Cammi, C. Pomelli, J. W. Ochterski, R. L. Martin, K. Morokuma, V. G. Zakrzewski, G. A. Voth, P. Salvador, J. J. Dannenberg, S. Dapprich, A. D. Daniels, O. Farkas, J. B. Foresman, J. V. Ortiz, J. Cioslowski and D. J. Fox, Gaussian 09, Revision A. 02, Gaussian Inc, Wallingford, CT, 2009 Search PubMed.
Footnote |
† Electronic supplementary information (ESI) available. See DOI: 10.1039/c6ra22869k |
|
This journal is © The Royal Society of Chemistry 2016 |
Click here to see how this site uses Cookies. View our privacy policy here.